Open Access
REVIEW
Engendered nanoparticles for treatment of brain tumors
1 Department of Cell and Molecular Biology, School of Biological Sciences, University of Leicester, Leicester, LE1 7RH, UK
2 Department of Cell and Molecular Biology, Faculty of Biological Sciences, Kharazmi University, Tehran, 15719-14911, Iran
3 Cancer Epidemiology Research Center, Aja University of Medical Sciences, Tehran, 14117-18541, Iran
4 Medical Biotechnology Research Center, Aja University of Medical Sciences, Tehran, 14117-18541, Iran
5 Department of Life Sciences, Faculty of Natural Sciences, Imperial College London, London, SW7 2AZ, UK
6 School of Pharmacy and Biomolecular Sciences, Royal College of Surgeons in Ireland (RCSI), Dublin, D02 YN77, Ireland
7 Research and Development, Science and Emerging Technologies, Aerogen Ltd., Galway Business Park, Galway, H91 HE94, Ireland
8 School of Pharmacy and Pharmaceutical Sciences, Trinity College, Dublin, D02 PN40, Ireland
* Corresponding Authors: MOHAMMAD DOROUDIAN. Email: ; RONAN MACLOUGHLIN. Email:
Oncology Research 2025, 33(1), 15-26. https://doi.org/10.32604/or.2024.053069
Received 23 April 2024; Accepted 04 September 2024; Issue published 20 December 2024
Abstract
Brain metastasis and primary glioblastoma multiforme represent the most common and lethal malignant brain tumors. Its median survival time is typically less than a year after diagnosis. One of the major challenges in treating these cancers is the efficiency of the transport of drugs to the central nervous system. The blood-brain barrier is cooperating with advanced stages of malignancy. The blood-brain barrier poses a significant challenge to delivering systemic medications to brain tumors. Nanodrug delivery systems have emerged as promising tools for effectively crossing this barrier. Additionally, the development of smart nanoparticles brings new hope for cancer diagnosis and treatment. These nanoparticles improve drug delivery efficiency, allowing for the creation of targeted and stimuli-responsive delivery methods. This review highlights recent advancements in nanoparticle and smart nanoparticle technologies for brain cancer treatment, exploring the range of nanoparticles under development, their applications, targeting strategies, and the latest progress in enhancing transport across the blood-brain barrier. It also addresses the ongoing challenges and potential benefits of these innovative approaches.Keywords
The brain organizes most of the body’s functions and processes. The brain controls breathing movements and digestion through sensory processing [1]. The cerebellum covers approximately 10 percent of the brain, and it accompanies the right and left hemispheres. They are responsible for performing higher sensory and motor functions and controlling movements [1,2]. The brain stem conducts a variety of automatic tasks. Furthermore, the appropriate propagation of behavioral processes is linked to the brain’s four lobes [1,3]. Brain diseases can cause serious damage to an individual’s body and life span [1]. Brain cancer is considered one of the most fatal diseases. The BBB is a significant limitation to treatment efficacy in brain cancer [4–6]. The BBB and strategies for minimizing its effect shall be discussed in detail throughout this review. Based on the information provided by the National Brain Tumor Society, 78,000 individuals are diagnosed with malignant brain tumors yearly in the United States [7]. The abnormal static and metastatic proliferation of cells can lead to benign and malignant tumors. Increased intracranial pressure, headaches, vomiting, altered consciousness, and seizures are among the symptoms of brain tumors. Brain tumors are typically defined based on their size and location within the brain. Glial cells are the most prevalent site of tumor invasion. Low-grade tumors are classed as Grades I and II [8,9], while higher-grade tumors are classified as Grades III and IV [10].
Among various cancer treatments, targeted therapies have shown promising results compared to traditional methods like chemotherapy. Conventional therapies often cause several undesired side effects, which can cause a tendency to prescribe lower doses than necessary for effective treatment [11]. Recent studies, including clinical trials outlined in Table 1, have expanded the scope of nanoparticle (NP) applications beyond traditional roles in drug delivery, imaging, and gene therapy. These studies explore the multifaceted potential of NPs, delving into advanced therapeutic strategies and diagnostic enhancements [12–14].
Extensive research has been conducted on nanoparticles due to their remarkable potential to traverse the BBB [15,16] (Figs. 1 and 2). In this review, we detail the current state of the art in active and passive targeting nanoparticle delivery systems. In the active targeting of cancer cells with high affinity and precision, the nanoparticles can be coupled with tumor-targeting moieties such as particular ligands, monoclonal antibodies, and carbohydrates [17]. We also provide an overview of brain cancer treatments using nanoparticles, covering the diversity of nanoparticles, some drug transportation mechanisms, and the most common issues encountered by these various high-potential drug delivery systems.
Figure 1: Nanoparticles can be thought of as ingenious couriers in the medical field, possessing an exceptional ability to assist medicines in reaching the brain by bypassing the blood-brain barrier. These diminutive heroes not only transport therapeutic agents but also provide protection and precise release at the intended site. Remarkably, these nanoparticles can be customized to execute diverse tasks, such as reducing their size to less than 100 nm, enabling them to effortlessly traverse biological barriers like agile acrobats. Furthermore, we have the innovative option to integrate different types of nanoparticles to create hybrids with augmented capabilities [18]. Organic nanoparticles, recognized for their compatibility with the human body and minimized harm, present immense potential for various applications [19,20]. By modifying the molecules on their surface, we can impart these nanoparticles with unique abilities, including electrical charges or the aptitude to target specific areas. Essentially, comprehending these nanoparticle experts and refining their design is akin to possessing a Swiss Army knife for drug delivery, presenting limitless possibilities for more precise and efficacious medical treatments [21–23].
Figure 2: Nanoparticles are divided into different categories, including organic, inorganic, and hybrid-based.
The blood-brain barrier as an obstacle to brain tumor drug delivery
The BBB is a barrier that separates the body’s circulatory system from the central nervous system (CNS) [24–26]. It is a diffusion barrier that prevents pathogens, neurotoxic plasma components, blood cells, therapeutic substances, and other chemicals from entering the brain, thereby maintaining brain homeostasis [27–30].
Endothelial cells (ECs), pericytes, astrocytes, tight junctions (TJs), neurons, and the basement membrane work in concert to ensure that the brain capillaries are virtually pore-free, thereby maintaining the integrity of the BBB and preventing the transport of small molecules and proteins. The BBB’s permeability is influenced by physiochemical characteristics such as molecular weight, charge, lipid solubility, surface activity, and the relative size of the molecules. These molecules can pass through paracellular conduits (between adjacent cells) or transcellular channels (through cells) of the BBB.
In the paracellular pathway, ions and solutes migrate across the BBB via passive diffusion, exploiting concentration gradients. Additionally, small lipophilic molecules like carbon dioxide can pass through the BBB via the transcellular pathway through passive diffusion [29,31,32]. Physiological factors that influence BBB permeability include efflux transporters (i.e., P-glycoprotein (P-GP)), enzymatic activity, and plasma protein concentration [33–36].
The breakdown of the BBB in disease conditions results in the leakage of toxic blood components into the CNS, cellular infiltration, and abnormal transport resulting in neurological impairments [27,37].
Strategies for overcoming the blood-brain barrier
The BBB presents a significant obstacle in the delivery of drugs to the brain, permitting only small, lipophilic molecules to pass through naturally. Complicating this challenge, the BBB is further protected by drug efflux transporters, predominantly from the ATP-binding cassette (ABC) superfamily, which frequently expels drugs trying to cross [38]. Overcoming this obstacle is essential for the treatment of brain cancer, given its intricacy, side effects, and the BBB’s resistance to most macromolecules [33,39].
To navigate this intricate barrier, nanomedicine has emerged as a promising solution [40–42]. Diverse inorganic, organic, and natural nanomaterials, enhanced with BBB-targeting ligands and cell-penetrating peptides (CPPs), have been engineered to facilitate drug delivery and theranostic approaches for brain tumors [43,44]. This transformative nanomedicine is poised to revolutionize brain cancer treatment by creating multifunctional BBB-crossing nanoparticles with remarkable properties like magnetic, optical, thermal, and tumor microenvironment-responsive capabilities [45,46].
Ultimately, the physiological role of the blood-brain barrier remains the primary culprit behind complications in current drug delivery strategies, emphasizing the critical need for innovative approaches to brain cancer treatment [47–50].
Nanoparticles offer a promising strategy for delivering therapeutic agents across the BBB [51]. The mechanism by which nanoparticles cross the BBB is multifaceted and involves several strategies. One of the primary methods is receptor-mediated transcytosis where nanoparticles are engineered to bind to specific receptors on the BBB, triggering a process that transports them across the barrier. Another mechanism is adsorptive-mediated transcytosis, which relies on the electrostatic interaction between the positively charged nanoparticles and the negatively charged cell membranes, facilitating their entry into the brain [52]. Nanoparticles can also be designed to temporarily disrupt the BBB, allowing them to pass through. This can be achieved by adjusting the nanoparticle’s surface properties or by using external forces like magnetic fields if the nanoparticles are magnetic [53]. Additionally, cell-penetrating peptides (CPPs) attached to nanoparticles can help them penetrate the BBB by inducing endocytosis [53].
Furthermore, nanoparticles can exploit natural transport mechanisms such as carrier-mediated transcytosis for substances like glucose or amino acids, by mimicking these molecules [53]. The intranasal route is another approach, offering a direct connection to the brain via the olfactory and trigeminal nerves, bypassing the BBB entirely. These mechanisms are under continuous research and development, aiming to enhance the delivery of therapeutics to the brain for the treatment of various central nervous system disorders. The ultimate goal is to achieve efficient and targeted delivery without compromising the integrity of the BBB or causing adverse effects [53].
The role of smart nanoparticles in the treatment of brain tumors
In the realm of advanced medical science, smart nanoparticles emerge as remarkable tools to overcome the formidable barrier known as the BBB and offer hope in treating brain tumors. Smart nanoparticles are devised to ferry drugs and therapeutic genes (in this case, anticancer drugs) into virulent cells without any lethal effect on normal cells [54,55]. The critical facet of tumor treatment is delivering as many drugs as possible to the targeted cells [56,57]. These nanocarriers, including lipid materials, polymers, nanocrystals, and inorganic nanomaterials, possess distinctive structural attributes that enable them to effectively traverse the BBB and precisely target elusive glioma cells [58]. Among the promising candidates for drug delivery in brain diseases, gold nanoparticles (AuNPs) are highly notable due to their adjustable size, optical characteristics, surface adaptability, and strong biocompatibility [59]. Meanwhile, clinical feasibility has introduced strategies involving liposomes, extracellular vesicles, and biomimicry, with liposomes emerging as particularly effective methods for enhancing drug delivery to the brain [34]. Numerous anticancer medications exhibit hydrophobic properties, which allows them to integrate seamlessly into the liposomal lipid bilayer. This characteristic facilitates their transport to other hydrophobic regions within the bloodstream [56,60]. A rat model study manifests that Liposomes with firm bilayers provide a higher transfer rate than liposomes with pliable bilayers. Moreover, it showed that PEGylated Liposomes are capable of releasing a hydrophobic drug at a higher speed rate in comparison to the control [56,61]. Another intriguing method reveals itself with magnetic nanoparticles (MNPs), coated with gold and linked to polyethylene glycol (PEG), exhibiting the potential to efficiently navigate the BBB under the influence of an external static magnetic field (SMF) [62]. Furthermore, nanoparticle customization through the integration of anti-transferrin receptor antibodies emerges as an exciting approach, allowing for precise targeting of brain endothelial cells (BECs) and seamless passage through the BBB, thus illuminating a promising path for the future delivery of biologics to the brain [63].
Smart delivery systems have been fabricated to define different stimuli. These external factors are ultrasound, radiofrequency, light, and temperature [56]. Ultrasound, a pressure wave, has excellent spatial and temporal resolution. The energy wasted during ultrasound interaction with tissues can cause heating and non-thermal mechanical effects. In drug delivery, a complex of ultrasound and microbubbles is being utilized. Microbubbles are capable of producing a diversity of mechanical forces when they are confronting ultrasound. By treating a particular site with concentrated ultrasound, sonoporation can be employed to augment the extravasation of drugs from blood to interstitial space [64,65]. More smart drug delivery methods using nanoparticles have shown remarkable improvements in cancer therapeutics, including active and passive targeted delivery systems [66].
Targeted nanoparticles represent precision-guided missiles within the sphere of drug delivery, exhibiting considerable potential to enhance the delivery and efficacy of therapies [67]. To address the obstacles presented by glioblastoma multiforme (GBM), scientists have designed polymeric nanoparticles that respond to Chlorotoxin, honing in on cancer cells and prompting their selective elimination [68]. Through the creation of a versatile nanoparticle platform, they have achieved an impressive degree of precision in targeting tumors, while concurrently minimizing any unintended adverse effects [69]. Additionally, in the realm of proteomics, modified nanoparticles have been employed to selectively retrieve peptides and proteins, creating high-throughput platforms that promote the advancement of our comprehension of intricate biological systems [70].
Zhu et al. developed an investigation around the creation of ANG-LP-MSN-PTX, a variant of mesoporous silica nanoparticles coated with lipids and modified with angiopep-2, for the aim of treating glioma, a deadly brain tumor, using targeted therapy. LRP1 (low-density lipoprotein receptor-related protein 1), a receptor protein that is abundant on the surface of both BBB and glioma cells, is planned to transport Paclitaxel (PTX) across the BBB using Nanoparticles. ANG-LP-MSN-PTX exhibited uniform hydrodynamic size, elevated drug loading capacity, and sustained release. Subsequent in vitro experiments revealed heightened targeting efficiency and increased apoptosis of C6 glioma cells. Pharmacokinetic analyses further demonstrated superior targeting efficiency of ANG-LP-MSN-PTX relative to LP-MSN-PTX. LP-MSN-PTX is a widely used chemotherapeutic agent, to target cells. This system typically involves loading PTX into mesoporous silica nanoparticles (MSNs) and then coating them with a lipid layer (LP), which helps to improve the stability and biocompatibility of the nanoparticles. Notably, in vivo experiments conducted on intracranial C6 glioma-bearing rats evinced increased therapeutic efficacy and prolonged survival time with ANG-LP-MSN-PTX. Ultimately, ANG-LP-MSN-PTX represents a promising targeted delivery system for the treatment of glioma [71].
Emerging medical approaches such as gene therapies, immunotherapies, targeted treatments, small molecules, and antibodies have been extensively studied to improve the survival rates of patients with glioblastoma multiforme (GBM) [45] Among these, the first approach, Nucleic Acid Therapeutics, provides precise control over gene expression. It utilizes DNA and mRNA to express specific genes that combat GBM, enhancing apoptosis in GBM cells or making them more receptive to chemotherapy [72]. Additionally, siRNA and miRNA selectively silence genes that contribute to GBM progression, angiogenesis, and immune suppression [73,74]. The versatility of nucleic acids also allows for customized treatments targeting unique genetic factors within individual tumors [75,76]. Furthermore, CRISPR-Cas9 technology enables gene editing to knock out oncogenes specific to GBM [77].
The second approach is Immunotherapy, which harnesses the immune system against GBM. Although once considered immune-privileged, recent studies have shown immune activity in the central nervous system [78]. Despite GBM creating a highly immunosuppressive microenvironment, immunotherapies such as checkpoint inhibitors and cancer vaccines aim to overcome these challenges [79,80]. Checkpoint inhibitors target immune checkpoints like PD-1, and personalized cancer vaccines based on neoantigens have shown promise. Additionally, CAR T-cell therapy introduces engineered T cells specific to GBM antigens into the patient [81]. However, resistance mechanisms and the need for combination therapies pose significant challenges in immunotherapy [81,82].
The third approach, Suicide Gene Therapy, involves reprogramming cancer cells to undergo targeted apoptosis upon exposure to a prodrug. Herpes simplex virus thymidine kinase (HSV-tk) is one such gene used, where HSV-tk-expressing cancer cells convert a non-toxic prodrug like ganciclovir into a toxic substance, leading to cell death [83,84]. However, mixed results in clinical trials have highlighted the need for improved gene delivery methods and higher transfection efficiency [85,86].
The fourth approach involves Small Molecules and Antibodies. Genetic and epigenetic screening has identified molecular targets in GBM, such as often overexpressed or mutated tyrosine kinase receptors EGFR and PDGFR, along with targets like VEGF in the tumor microenvironment [87]. Small molecule inhibitors can block the activity of these receptors, disrupting essential signaling pathways for GBM growth. Monoclonal antibodies, meanwhile, can neutralize factors like VEGF [88]. Despite the potential of these targeted therapies, GBM’s heterogeneity and the development of resistance mechanisms present challenges. These therapies may be more effective when used in combination with other treatments [45,89].
Each of these approaches holds potential in the fight against GBM but also comes with unique sets of challenges that require further research and development for more effective treatments.
Stimuli Responsive Nanoparticles
Stimuli Responsive Nanoparticles are advanced materials designed to react to specific triggers such as pH and temperature, enabling controlled drug delivery and release. These smart nanosystems adapt their behavior in response to the body’s microenvironment or external stimuli, offering precision in treatments. Stimuli-responsive nanoparticles possessing intelligent features have surfaced as a promising technique for targeted drug delivery in biomedical domains [90]. These nanoparticles do not seem to be customizable to interact with any specific stimuli that are present in the tumor microenvironment, including high glutathione concentration, acidity, reactive oxygen species (ROS), hypoxia, and over-expressed enzymes [91]. pH and redox, nanoparticles that respond to endogenous stimuli, and polymeric nanoparticles that can be transformed into systems that are sensitive to stimuli. These nanoparticles can sustain stability in physiological conditions and discharge drugs exclusively in tumor tissues, providing improved therapeutic efficacy and better biosafety [90,92–94].
With the advancement of nanomedicine technology, stimuli-responsive nanocarriers have become increasingly prominent in antitumor therapy. The tumor microenvironment (TME) is distinguished by unique characteristics, such as sourness, raised glutathione (GSH) concentrations, lack of oxygen, hyperactive enzymes, and ample reactive oxygen species (ROS), all of which are intricately tied to tumor development. Interestingly, these TME characteristics can be leveraged for intelligent drug delivery systems that target tumor tissues. Stimuli-responsive nanoparticles (srNPs) exhibit stability in physiological conditions but can rapidly release drugs upon specific triggers, extending circulation and enhancing cancer cell uptake, ultimately achieving superior therapeutic efficacy and enhanced safety [95].
This study delves into the challenges and future directions in the development of stimulus-responsive nanoparticles (srNPs), shedding light on their potential clinical implications. Nanomedicine has notably transformed drug delivery to tumors, leveraging unique molecular characteristics. Despite the enhancements nano drug delivery systems (NDDS) have brought to traditional chemotherapy, optimizing drug bioavailability within tumor tissues remains critical, especially in terms of cellular uptake and intracellular release. srNPs, designed with an intricate understanding of the disparities between normal and cancerous tissues, render this review an invaluable guide to the progress in srNPs for cancer treatment. The review briefly covers the endogenous stimuli of the tumor microenvironment (TME)—such as low pH, elevated glutathione (GSH) levels, overexpressed enzymes, excessive reactive oxygen species (ROS), and hypoxia—summarizes srNPs’ therapeutic applications, and encourages further research to hasten their clinical integration. Additionally, Table 2 is introduced to discuss the properties of the TME in detail [92,96]. These characteristics collectively contribute to the complex and challenging nature of the tumor microenvironment, impacting cancer progression and therapeutic strategies.
Passive and active targeting drug delivery systems
A tumor-targeting drug delivery system comprises a tumor recognition moiety and a cytotoxic agent coupled directly or via a linker to form a conjugate [97–100]. The fact that the endothelium of blood vessels becomes more permeable than in a healthy state under specific situations (inflammation/hypoxia, which is typical of tumors) is now well-established. Rapidly growing tumors generate new vessels or engulf existing blood vessels in response to hypoxia. These newly generated leaky vessels allow for more preferential permeation of larger macromolecules and nano-systems into the tumor stroma. Furthermore, the retention of NPs is aided by the absence of normal lymphatic outflow in the tumor [101–103]. Nanoparticle targeting can be accomplished in two ways: passive targeting and active targeting [104,105] (Fig. 3).
Figure 3: Active targeting makes it easier for tumor cells to actively take up nanoparticles. The effective localization of nanoparticles into the tumor microenvironment is made possible by passive targeting.
The size of a nanoparticle is an essential factor in determining passive targeting and biodistribution within brain tumors. Nanoparticles in the 10–100 nm size range take advantage of a brain tumor’s hyper-vascularized, leaky, and impaired lymphatic drainage system to passively target and access the intra-tumoral region while being denied access to the healthy brain tissue [106]. Passive targeting, also known as physical targeting, is based on creating a drug carrier complex resistant to removal by bodily functions such as metabolism, excretion, opsonization, and phagocytosis, allowing the complex to circulate in the bloodstream and be transmitted [107–109]. Due to physicochemical or pharmacological considerations, passive targeting installs a drug or drug-carrier structure at a specified spot. Passive targeting leverages the enhanced permeability and retention (EPR) effect, allowing nanoparticles to accumulate in brain tissue without specific targeting mechanisms. Due to the vast phenotypic variability of malignant cells and tumors, only a few regularly adopted approaches for targeting tumors and tumor cells exist. Many cancers exhibit an EPR effect that can be utilized for passive anti-tumor drug targeting [110]. The preferential accumulation of chemotherapeutic drugs in solid tumors is the most well-known example of passive targeting. As a result, tumor tissues have increased vascular permeability compared to healthy tissue. Passive targeting allows for highly selective ligand-receptor interactions, which permits precise targeting at the site of interest. On the other hand, active targeting involves functionalizing nanoparticles with ligands that bind to specific receptors on the BBB, facilitating receptor-mediated transcytosis. This process is influenced by the nanoparticle’s size, surface coating, and charge, which are optimized to improve BBB crossing efficiency. Smaller nanoparticles with a neutral or slightly negative charge and a hydrophilic surface coating are generally favored for efficient BBB penetration [111].
The active targeted drug delivery system is based on a technology that delivers a specific amount of a therapeutic or diagnostic agent or both to an affected tissue/organ of the body [112,113]. Targeting ligands are added to the surface of the Nanocarrier in active targeting to bind to suitable receptors expressed at the target site. The ligand is chosen to attach to a receptor that is overexpressed by tumor cells or vessels but not by normal cells [114–116]. Targeted receptors should also be expressed uniformly on all targeted cells. Due to the “binding-site barrier,” the ligands’ binding affinity influences tumor penetration. Due to the dynamic flow environment of the bloodstream, high-affinity binding appears to be desirable for sites where cells are readily accessible, such as tumor vasculature [117]. The diverse moieties investigated thus far as targeting ligands include carbohydrates (e.g., galactose), monoclonal antibodies (e.g., anti-Her2, anti-EGFR), peptides (e.g., Arg-Gly-Asp or RGD), proteins (e.g., lectins, transferrin), vitamins (e.g., vitamin D), and aptamers (e.g., RNA aptamers against HIV glycoprotein) [118]. Some of the drawbacks of passive targeting approaches may be overcome by using a more active targeting mechanism. The discovery of disease-specific biomarkers has paved the way for this approach in the nanomedicine sector [119]. Active targeting usually entails using one or more targeting moieties attached to the nanoparticle’s surface that interact particularly with antigens or receptors that are either uniquely expressed or overexpressed on tumor cells compared to normal tissues [120]. If inter-finalizing receptors are targeted, this technique also has the added benefit of boosting nanoparticle transport into cells via a specific pathway after the particles reach the tumor extracellular space. Moreover, ligands can be used to target intravascular tumor cells or tumor blood vessels’ endothelial cells to increase nanoparticle accumulation within the disease location [121]. Because several molecular mechanisms of chemoresistance characterize Glioblastoma (GB), developing dynamically targeted NPs to surface cell markers, signaling pathways, and the tumor microenvironment poses an exciting and demanding possibility. These methods work by attaching particular ligands to the NPs’ structure, allowing for the selective identification of specific receptors overexpressed on tumor cell surfaces [122].
Solid lipid nanoparticles (SLNs) have emerged as a promising vehicle for delivering therapeutic agents across the BBB, offering a range of advantages such as controlled drug release, extended circulation within the bloodstream, precise targeting, and reduced potential for toxicity [123]. Notably, researchers have made significant strides in this field, with the development of lipid-coated mesoporous silica nanoparticles modified with Angiopep-2. These innovative nanocarriers have demonstrated the ability to transport paclitaxel (PTX) through the BBB, facilitated by the low-density lipoprotein receptor-related protein 1 (LRP1), thus showing great potential for treating gliomas [71].
Nanoparticle-based therapies show great promise for the diagnosis and treatment of brain tumors, offering advantages such as high sensitivity, lower toxicity, and enhanced safety compared to traditional methods. These therapies, particularly those utilizing nanomaterials, have been developed to transport therapeutic drugs across the blood-brain barrier (BBB), a major obstacle in treating central nervous system diseases. Nanomaterials are valuable due to their high drug-loading capacity, stability, prolonged circulation in the bloodstream, controlled drug release, and targeted impact. However, existing in vitro and in vivo BBB models face limitations, which complicates the clinical translation of these nanodrug delivery systems.
Navigating the regulatory landscape for nanoparticles in medicine is challenging, with ethical considerations focused on patient safety, potential long-term effects, and managing the risks associated with nanotoxicity. It is crucial to balance the benefits of nanomedicine with the responsibility to avoid harm, adhering to ethical principles like non-maleficence and beneficence. Ongoing research and dialogue will be essential to address these challenges, ensuring that the application of nanomedicine in treating brain cancer is both safe and effective.
Financial barriers, such as high production costs and limited insurance coverage, can limit patient access to these innovative therapies. To address these challenges, efforts should be made to optimize manufacturing processes, advocate for policy changes, and explore public-private partnerships to reduce costs and improve accessibility.
Nanotechnology is becoming increasingly important in various scientific fields due to its numerous applications and benefits. The primary limitation in treating central nervous system diseases is the difficulty of drug transmission across the BBB, a challenge that nanotechnology is helping to overcome. Nanotechnology’s ability to transport drugs to targeted organs offers higher efficacy and fewer side effects on other organs. Future advancements in nanotechnology will likely lead to the development of multifunctional nanoparticles that can cross the BBB more efficiently, potentially incorporating targeting ligands or magnetic properties to direct them to tumor sites. The integration of diagnostic and therapeutic functions, known as theranostics, will enable real-time monitoring of treatment responses.
In gene therapy, nanoparticles could deliver genetic material directly to brain tumor cells to correct genetic mutations or silence oncogenes. The use of nanoparticles in immunotherapy to help the immune system recognize and attack brain tumor cells also holds significant potential. The future of nanoparticles in brain tumor treatment may involve personalized medicine, with treatments tailored to the unique molecular profile of each patient’s tumor.
This study highlights recent advancements in nanotechnology for brain cancer treatment, particularly the use of nanoparticles to cross the BBB and target brain tumors with precision. These advancements offer promising alternatives to conventional therapies, which often fail due to the restrictive nature of the BBB. Additionally, the integration of theranostic functions in these nanoparticles represents an important development in personalized medicine.
Despite these advancements, the study acknowledges several limitations. The in vitro and in vivo models used may not fully replicate the complexity of the human brain environment. Furthermore, while this study discusses the potential of various nanoparticles, it lacks comprehensive clinical data on the long-term safety and efficacy of these therapies in human patients. The regulatory and ethical challenges associated with nanomedicine, including potential nanotoxicity and the high cost of nanoparticle production, also need to be addressed.
Future research should focus on developing more accurate in vitro and in vivo models that better mimic the human BBB and brain tumor environment. Clinical trials are necessary to validate the safety and efficacy of these therapies in human patients. Additionally, optimizing the cost-effectiveness of nanoparticle production will be crucial to making these advanced therapies more accessible in clinical practice. Personalized nanomedicine has the potential to become a key approach in treating brain tumors, particularly for patients who do not respond to traditional therapies.
Finally, this study recognizes the importance of considering biological variables, such as sex and gender, which may influence the effectiveness and safety of nanoparticle-based therapies. Future research should take these factors into account to develop more personalized and effective treatments for brain cancer.
In summary, nanotechnology holds significant potential for treating brain cancer, and this study provides valuable insights into overcoming the challenges posed by the BBB. As the field of nanomedicine continues to advance, addressing current limitations and pursuing innovative solutions will be essential for improving the efficacy and accessibility of these therapies, ultimately leading to better outcomes for patients with brain cancer.
Acknowledgement: None.
Funding Statement: The authors received no specific funding for this study.
Author Contributions: The authors confirm their contribution to the paper as follows: Conceptualization: Mohammad Doroudian, Mahdieh Soezi, Ronan MacLoughlin; Writing—Review & Editing: Soroush Soleymani, Mohammad Doroudian, Ronan MacLoughlin, Ali Beladi, Kiarash Asgari, Aso Mobarakshahi, Aryana Aghaeipour; Artwork: Soroush Soleymani, Aso Mobarakshahi; Supervision: Mohammad Doroudian, Mahdieh Soezi. All authors reviewed the results and approved the final version of the manuscript.
Availability of Data and Materials: Not applicable.
Ethics Approval: Not applicable.
Conflicts of Interest: The authors declare no conflicts of interest to report regarding the present study.
References
1. Mukhtar M, Bilal M, Rahdar A, Barani M, Arshad R, Behl T, et al. Nanomaterials for diagnosis and treatment of brain cancer: recent updates. Chemosensors. 2020;8(4):117. [Google Scholar]
2. Gonçalves FG, Hwang M. Superficial anatomy of the neonatal cerebrum—an ultrasonographic roadmap. Pediatr Radiol. 2021;51(3):353–70. [Google Scholar]
3. Zhang JW. Secrets of the brain: an introduction to the brain anatomical structure and biological function. 2019. doi:10.48550/arXiv.1906.03314. [Google Scholar] [CrossRef]
4. Alphandéry E. Nano-therapies for glioblastoma treatment. Cancers. 2020;12(1):242. [Google Scholar]
5. Zhou Y, Peng Z, Seven ES, Leblanc RM. Crossing the blood-brain barrier with nanoparticles. J Control Release. 2018;270:290–303. [Google Scholar] [PubMed]
6. Yang T, Martin P, Fogarty B, Brown A, Schurman K, Phipps R, et al. Exosome delivered anticancer drugs across the blood-brain barrier for brain cancer therapy in Danio rerio. Pharm Res. 2015;32(6):2003–14. doi:10.1007/s11095-014-1593-y. [Google Scholar] [PubMed] [CrossRef]
7. Stiller CA, Gatta G. The epidemiology of cancer in children and adolescents. In: Caron HN, Biondi A, Boterberg T, Doz F, editors. Oxford textbook of cancer in children. Oxford: Oxford University Press; 2020. [Google Scholar]
8. Morshed RA, Young JS, Hervey-Jumper SL, Berger MS. The management of low-grade gliomas in adults. J Neurosurg Sci. 2019;63(4):450–7. doi:10.23736/S0390-5616.19.04701-5. [Google Scholar] [PubMed] [CrossRef]
9. Lapointe S, Perry A, Butowski NA. Primary brain tumours in adults. Lancet. 2018;392(10145):432–46. doi:10.1016/S0140-6736(18)30990-5. [Google Scholar] [PubMed] [CrossRef]
10. Tang W, Fan W, Lau J, Deng L, Shen Z, Chen X. Emerging blood-brain-barrier-crossing nanotechnology for brain cancer theranostics. Chem Soc Rev. 2019;48(11):2967–3014. doi:10.1039/C8CS00805A. [Google Scholar] [PubMed] [CrossRef]
11. Senapati S, Mahanta AK, Kumar S, Maiti P. Controlled drug delivery vehicles for cancer treatment and their performance. Signal Transduct Target Ther. 2018;3:7. doi:10.1038/s41392-017-0004-3. [Google Scholar] [PubMed] [CrossRef]
12. Farokhzad OC, Langer R. Impact of nanotechnology on drug delivery. ACS Nano. 2009;3(1):16–20. [Google Scholar] [PubMed]
13. Aghebati-Maleki A, Dolati S, Ahmadi M, Baghbanzhadeh A, Asadi M, Fotouhi A, et al. Nanoparticles and cancer therapy: perspectives for application of nanoparticles in the treatment of cancers. J Cell Physiol. 2020;235(3):1962–72. [Google Scholar] [PubMed]
14. Verma NK, Crosbie-Staunton K, Satti A, Gallagher S, Ryan KB, Doody T, et al. Magnetic core-shell nanoparticles for drug delivery by nebulization. J Nanobiotechnol. 2013;11:1. [Google Scholar]
15. Guido C, Baldari C, Maiorano G, Mastronuzzi A, Carai A, Quintarelli C, et al. Nanoparticles for diagnosis and target therapy in pediatric brain cancers. Diagnostics. 2022;12(1):173. [Google Scholar] [PubMed]
16. Kashyap K, Shukla R. Drug delivery and targeting to the brain through nasal route: mechanisms, applications and challenges. Curr Drug Deliv. 2019;16(10):887–901. [Google Scholar] [PubMed]
17. Bertrand N, Wu J, Xu X, Kamaly N, Farokhzad OC. Cancer nanotechnology: the impact of passive and active targeting in the era of modern cancer biology. Adv Drug Deliv Rev. 2014;66:2–25. [Google Scholar] [PubMed]
18. Bahrami A, Delshadi R, Jafari SM, Williams L. Nanoencapsulated nisin: an engineered natural antimicrobial system for the food industry. Trends Food Sci Technol. 2019;94:20–31. [Google Scholar]
19. Hanjani NA, Esmaelizad N, Zanganeh S, Gharavi AT, Heidarizadeh P, Radfar M, et al. Emerging role of exosomes as biomarkers in cancer treatment and diagnosis. Crit Rev Oncol Hematol. 2022;169:103565. [Google Scholar] [PubMed]
20. Nahand JS, Vandchali NR, Darabi H, Doroudian M, Banafshe HR, Moghoofei M, et al. Exosomal microRNAs: novel players in cervical cancer. Epigenomics. 2020;12(18):1651–60. [Google Scholar] [PubMed]
21. Hejabi F, Abbaszadeh MS, Taji S, O’Neill A, Farjadian F, Doroudian M. Nanocarriers: a novel strategy for the delivery of CRISPR/Cas systems. Front Chem. 2022;10:957572. [Google Scholar] [PubMed]
22. Tundisi LL, Ataide JA, Costa JS, Coêlho DF, Liszbinski RB, Lopes AM, et al. Nanotechnology as a tool to overcome macromolecules delivery issues. Colloids Surf B Biointerfaces. 2023;222:113043. [Google Scholar] [PubMed]
23. Galvin P, Thompson D, Ryan KB, McCarthy A, Moore AC, Burke CS, et al. Nanoparticle-based drug delivery: case studies for cancer and cardiovascular applications. Cell Mol Life Sci. 2012;69(3):389–404. [Google Scholar] [PubMed]
24. Bruinsmann FA, Richter Vaz G, de Cristo Soares Alves A, Aguirre T, Raffin Pohlmann A, Stanisçuaski Guterres S, et al. Nasal drug delivery of anticancer drugs for the treatment of glioblastoma: preclinical and clinical trials. Molecules. 2019;24(23):4312. [Google Scholar] [PubMed]
25. Tang L, Feng Y, Gao S, Mu Q, Liu C. Nanotherapeutics overcoming the blood-brain barrier for glioblastoma treatment. Front Pharmacol. 2021;12:786700. [Google Scholar] [PubMed]
26. Sharma RK, Calderon C, Vivas-Mejia PE. Targeting non-coding RNA for glioblastoma therapy: the challenge of overcomes the blood-brain barrier. Front Med Technol. 2021;3:678593. [Google Scholar] [PubMed]
27. Sweeney MD, Zhao Z, Montagne A, Nelson AR, Zlokovic BV. Blood-brain barrier: from physiology to disease and back. Physiol Rev. 2019;99(1):21–78. [Google Scholar] [PubMed]
28. Umlauf BJ, Shusta EV. Exploiting BBB disruption for the delivery of nanocarriers to the diseased CNS. Curr Opin Biotechnol. 2019;60:146–52. [Google Scholar] [PubMed]
29. Lombardo SM, Schneider M, Türeli AE, Günday Türeli N. Key for crossing the BBB with nanoparticles: the rational design. Beilstein J Nanotechnol. 2020;11:866–83. [Google Scholar] [PubMed]
30. Agrawal M, Saraf S, Saraf S, Dubey SK, Puri A, Patel RJ, et al. Recent strategies and advances in the fabrication of nano lipid carriers and their application towards brain targeting. J Control Release. 2020;321:372–415. [Google Scholar] [PubMed]
31. Han L, Jiang C. Evolution of blood-brain barrier in brain diseases and related systemic nanoscale brain-targeting drug delivery strategies. Acta Pharm Sin B. 2021;11(8):2306–25. [Google Scholar] [PubMed]
32. Bagchi S, Chhibber T, Lahooti B, Verma A, Borse V, Jayant RD. In-vitro blood-brain barrier models for drug screening and permeation studies: an overview. Drug Des Devel Ther. 2019;13:3591–605. [Google Scholar] [PubMed]
33. Sarkaria JN, Hu LS, Parney IF, Pafundi DH, Brinkmann DH, Laack NN, et al. Is the blood-brain barrier really disrupted in all glioblastomas? A critical assessment of existing clinical data. Neuro Oncol. 2018;20(2):184–91. [Google Scholar] [PubMed]
34. Lundy DJ, Nguyễn H, Hsieh PCH. Emerging nano-carrier strategies for brain tumor drug delivery and considerations for clinical translation. Pharmaceutics. 2021;13(8):1193. [Google Scholar] [PubMed]
35. Pardridge WM. Blood-brain barrier genomics and the use of endogenous transporters to cause drug penetration into the brain. Curr Opin Drug Discov Devel. 2003;6(5):683–91. [Google Scholar] [PubMed]
36. Zhou X, Smith QR, Liu X. Brain penetrating peptides and peptide-drug conjugates to overcome the blood-brain barrier and target CNS diseases. Wiley Interdiscip Rev Nanomed Nanobiotechnol. 2021;13(4):e1695. [Google Scholar] [PubMed]
37. Liebner S, Dijkhuizen RM, Reiss Y, Plate KH, Agalliu D, Constantin G. Functional morphology of the blood-brain barrier in health and disease. Acta Neuropathol. 2018;135(3):311–36. [Google Scholar] [PubMed]
38. Knox EG, Aburto MR, Clarke G, Cryan JF, O’Driscoll CM. The blood-brain barrier in aging and neurodegeneration. Mol Psychiatry. 2022;27(6):2659–73. [Google Scholar] [PubMed]
39. Pandit R, Chen L, Götz J. The blood-brain barrier: physiology and strategies for drug delivery. Adv Drug Deliv Rev. 2020;165-166:1–14. [Google Scholar] [PubMed]
40. Doroudian M, Azhdari MH, Goodarzi N, O’Sullivan D, Donnelly SC. Smart nanotherapeutics and lung cancer. Pharmaceutics. 2021;13(11):1972. [Google Scholar] [PubMed]
41. Hosseinkazemi H, Samani S, O’Neill A, Soezi M, Moghoofei M, Azhdari MH, et al. Applications of iron oxide nanoparticles against breast cancer. J Nanomater. 2022;2022(2):1–12. doi:10.1155/2022/6493458. [Google Scholar] [CrossRef]
42. Doroudian M, Zanganeh S, Abbasgholinejad E, Donnelly SC. Nanomedicine in lung cancer immunotherapy. Front Bioeng Biotechnol. 2023;11:1144653. doi:10.3389/fbioe.2023.1144653. [Google Scholar] [PubMed] [CrossRef]
43. Bergmann S, Lawler SE, Qu Y, Fadzen CM, Wolfe JM, Regan MS, et al. Blood-brain-barrier organoids for investigating the permeability of CNS therapeutics. Nat Protoc. 2018;13(12):2827–43. doi:10.1038/s41596-018-0066-x. [Google Scholar] [PubMed] [CrossRef]
44. Tam DY, Ho JW, Chan MS, Lau CH, Chang TJH, Leung HM, et al. Penetrating the blood-brain barrier by self-assembled 3D DNA nanocages as drug delivery vehicles for brain cancer therapy. ACS Appl Mater Interfaces. 2020;12(26):28928–40. [Google Scholar] [PubMed]
45. Karlsson J, Luly KM, Tzeng SY, Green JJ. Nanoparticle designs for delivery of nucleic acid therapeutics as brain cancer therapies. Adv Drug Deliv Rev. 2021;179:113999. doi:10.1016/j.addr.2021.113999. [Google Scholar] [PubMed] [CrossRef]
46. Zhang H, Van Os WL, Tian X, Zu G, Ribovski L, Bron R, et al. Development of curcumin-loaded zein nanoparticles for transport across the blood-brain barrier and inhibition of glioblastoma cell growth. Biomater Sci. 2021;9(21):7092–103. doi:10.1039/D0BM01536A. [Google Scholar] [PubMed] [CrossRef]
47. Bajracharya R, Song JG, Back SY, Han HK. Recent advancements in non-invasive formulations for protein drug delivery. Comput Struct Biotechnol J. 2019;17:1290–308. [Google Scholar] [PubMed]
48. Ferraris C, Cavalli R, Panciani PP, Battaglia L. Overcoming the blood-brain barrier: successes and challenges in developing nanoparticle-mediated drug delivery systems for the treatment of brain tumours. Int J Nanomed. 2020;15:2999–3022. doi:10.2147/IJN.S231479. [Google Scholar] [PubMed] [CrossRef]
49. Doroudian M, MacLoughlin R, Poynton F, Prina-Mello A, Donnelly SC. Nanotechnology based therapeutics for lung disease. Thorax. 2019;74(10):965–76. [Google Scholar] [PubMed]
50. Doroudian M, O’Neill A, Mac Loughlin R, Prina-Mello A, Volkov Y, Donnelly SC. Nanotechnology in pulmonary medicine. Curr Opin Pharmacol. 2021;56:85–92. [Google Scholar] [PubMed]
51. Shahrivar RY, Fakhr ZA, Abbasgholinejad E, Doroudian M. Smart lipid-based nanoparticles in lung cancer treatment: current status and future directions. Adv Ther. 2023;6(12):140. doi:10.1002/adtp.202300275. [Google Scholar] [CrossRef]
52. Mhaske A, Shukla S, Ahirwar K, Singh KK, Shukla R. Receptor-assisted nanotherapeutics for overcoming the blood-brain barrier. Mol Neurobiol. 2024;26:1944. doi:10.1007/s12035-024-04015-9. [Google Scholar] [PubMed] [CrossRef]
53. Xie J, Shen Z, Anraku Y, Kataoka K, Chen X. Nanomaterial-based blood-brain-barrier (BBB) crossing strategies. Biomaterials. 2019;224:119491. [Google Scholar] [PubMed]
54. Zhu Y, Liao L. Applications of nanoparticles for anticancer drug delivery: a review. J Nanosci Nanotechnol. 2015;15(7):4753–73. [Google Scholar] [PubMed]
55. Farjadian F, Ghasemi S, Akbarian M, Hoseini-Ghahfarokhi M, Moghoofei M, Doroudian M. Physically stimulus-responsive nanoparticles for therapy and diagnosis. Front Chem. 2022;10:952675. [Google Scholar] [PubMed]
56. Lee BK, Yun YH, Park K. Smart nanoparticles for drug delivery: boundaries and opportunities. Chem Eng Sci. 2015;125:158–64. [Google Scholar] [PubMed]
57. Boswell GW, Buell D, Bekersky I. AmBisome (liposomal amphotericin Ba comparative review. J Clin Pharmacol. 1998;38(7):583–92. doi:10.1002/j.1552-4604.1998.tb04464.x. [Google Scholar] [PubMed] [CrossRef]
58. Zeng Y, Yao X, Liu X, He X, Li L, Liu X, et al. Anti-angiogenesis triggers exosomes release from endothelial cells to promote tumor vasculogenesis. J Extracell Vesicles. 2019;8(1):1629865. doi:10.1080/20013078.2019.1629865. [Google Scholar] [PubMed] [CrossRef]
59. Zhang J, Yang T, Huang W, Yu Y, Sun T. Applications of gold nanoparticles in brain diseases across the blood-brain barrier. Curr Med Chem. 2022;29(39):6063–83. doi:10.2174/0929867329666220527121943. [Google Scholar] [PubMed] [CrossRef]
60. Papahadjopoulos D, Allen TM, Gabizon A, Mayhew E, Matthay K, Huang SK, et al. Sterically stabilized liposomes: improvements in pharmacokinetics and antitumor therapeutic efficacy. Proc Natl Acad Sci U S A. 1991;88(24):11460–4. doi:10.1073/pnas.88.24.11460. [Google Scholar] [PubMed] [CrossRef]
61. Chen H, Kim S, Li L, Wang S, Park K, Cheng JX. Release of hydrophobic molecules from polymer micelles into cell membranes revealed by Forster resonance energy transfer imaging. Proc Natl Acad Sci U S A. 2008;105(18):6596–601. doi:10.1073/pnas.0707046105. [Google Scholar] [PubMed] [CrossRef]
62. Chen J, Yuan M, Madison CA, Eitan S, Wang Y. Blood-brain barrier crossing using magnetic stimulated nanoparticles. J Control Release. 2022;345:557–71. [Google Scholar] [PubMed]
63. Thomsen MS, Johnsen KB, Kucharz K, Lauritzen M, Moos T. Blood-brain barrier transport of transferrin receptor-targeted nanoparticles. Pharmaceutics. 2022;14(10):2237. [Google Scholar] [PubMed]
64. Yudina A, Lepetit-Coiffé M, De Smet M, Langereis S, Grüll H, Moonen C. In vivo temperature controlled ultrasound-mediated intracellular delivery of cell-impermeable compounds. J Control Release. 2012;161(1):90–7. [Google Scholar] [PubMed]
65. Sanches PG, Rossin R, Böhmer M, Tiemann K, Grüll H. Real-time imaging and kinetics measurements of focused ultrasound-induced extravasation in skeletal muscle using SPECT/CT. J Control Release. 2013;168(3):262–70. [Google Scholar] [PubMed]
66. Morales-Cruz M, Delgado Y, Castillo B, Figueroa CM, Molina AM, Torres A, et al. Smart targeting to improve cancer therapeutics. Drug Des Devel Ther. 2019;13:3753–72. [Google Scholar] [PubMed]
67. Kou L, Sun R, Xiao S, Cui X, Sun J, Ganapathy V, et al. OCTN2-targeted nanoparticles for oral delivery of paclitaxel: differential impact of the polyethylene glycol linker size on drug delivery in vitro, in situ, and in vivo. Drug Deliv. 2020;27(1):170–9. [Google Scholar] [PubMed]
68. Locatelli E, Bost W, Fournelle M, Llop J, Gil L, Arena F, et al. Targeted polymeric nanoparticles containing gold nanorods: a therapeutic approach against glioblastoma. J Nanopart Res. 2014;16:2304. doi:10.1007/s11051-014-2304-7. [Google Scholar] [CrossRef]
69. Chen F, Ma K, Zhang L, Madajewski B, Zanzonico P, Sequeira S, et al. Target-or-clear zirconium-89 labeled silica nanoparticles for enhanced cancer-directed uptake in melanoma: a comparison of radiolabeling strategies. Chem Mater. 2017;29(19):8269–81. [Google Scholar] [PubMed]
70. Stolowitz ML. On-target and nanoparticle-facilitated selective enrichment of peptides and proteins for analysis by MALDI-MS. Proteomics. 2012;12(23–4):3438–50. [Google Scholar] [PubMed]
71. Zhu J, Zhang Y, Chen X, Zhang Y, Zhang K, Zheng H, et al. Angiopep-2 modified lipid-coated mesoporous silica nanoparticles for glioma targeting therapy overcoming BBB. Biochem Biophys Res Commun. 2021;534:902–7. [Google Scholar] [PubMed]
72. Pardi N, Hogan MJ, Porter FW, Weissman D. mRNA vaccines—a new era in vaccinology. Nat Rev Drug Discov. 2018;17(4):261–79. doi:10.1038/nrd.2017.243. [Google Scholar] [PubMed] [CrossRef]
73. Riley RS, June CH, Langer R, Mitchell MJ. Delivery technologies for cancer immunotherapy. Nat Rev Drug Discov. 2019;18(3):175–96. doi:10.1038/s41573-018-0006-z. [Google Scholar] [PubMed] [CrossRef]
74. Yang B, Jeang J, Yang A, Wu TC, Hung CF. DNA vaccine for cancer immunotherapy. Hum Vaccin Immunother. 2014;10(11):3153–64. doi:10.4161/21645515.2014.980686. [Google Scholar] [PubMed] [CrossRef]
75. Liu MA. DNA vaccines: an historical perspective and view to the future. Immunol Rev. 2011;239(1):62–84. doi:10.1111/j.1600-065X.2010.00980.x. [Google Scholar] [PubMed] [CrossRef]
76. Singh P, Singh A, Shah S, Vataliya J, Mittal A, Chitkara D. RNA interference nanotherapeutics for treatment of glioblastoma multiforme. Mol Pharm. 2020;17(11):4040–66. doi:10.1021/acs.molpharmaceut.0c00709. [Google Scholar] [PubMed] [CrossRef]
77. Guo D, Wang B, Han F, Lei T. RNA interference therapy for glioblastoma. Expert Opin Biol Ther. 2010;10(6):927–36. doi:10.1517/14712598.2010.481667. [Google Scholar] [PubMed] [CrossRef]
78. Louveau A, Smirnov I, Keyes TJ, Eccles JD, Rouhani SJ, Peske JD, et al. Structural and functional features of central nervous system lymphatic vessels. Nature. 2015;523(7560):337–41. doi:10.1038/nature14432. [Google Scholar] [PubMed] [CrossRef]
79. Kamran N, Calinescu A, Candolfi M, Chandran M, Mineharu Y, Asad AS, et al. Recent advances and future of immunotherapy for glioblastoma. Expert Opin Biol Ther. 2016;16(10):1245–64. doi:10.1080/14712598.2016.1212012. [Google Scholar] [PubMed] [CrossRef]
80. Reardon DA, Freeman G, Wu C, Chiocca EA, Wucherpfennig KW, Wen PY, et al. Immunotherapy advances for glioblastoma. Neuro Oncol. 2014;16(11):1441–58. doi:10.1093/neuonc/nou212. [Google Scholar] [PubMed] [CrossRef]
81. Preusser M, Lim M, Hafler DA, Reardon DA, Sampson JH. Prospects of immune checkpoint modulators in the treatment of glioblastoma. Nat Rev Neurol. 2015;11(9):504–14. doi:10.1038/nrneurol.2015.139. [Google Scholar] [PubMed] [CrossRef]
82. Wollmann G, Ozduman K, Van den Pol AN. Oncolytic virus therapy for glioblastoma multiforme: concepts and candidates. Cancer J. 2012;18(1):69–81. doi:10.1097/PPO.0b013e31824671c9. [Google Scholar] [PubMed] [CrossRef]
83. Fillat C, Carrió M, Cascante A, Sangro B. Suicide gene therapy mediated by the Herpes Simplex virus thymidine kinase gene/Ganciclovir system: fifteen years of application. Curr Gene Ther. 2003;3(1):13–26. doi:10.2174/1566523033347426. [Google Scholar] [PubMed] [CrossRef]
84. Kianmanesh AR, Perrin H, Panis Y, Fabre M, Nagy HJ, Houssin D, et al. A “distant” bystander effect of suicide gene therapy: regression of nontransduced tumors together with a distant transduced tumor. Hum Gene Ther. 1997;8(15):1807–14. doi:10.1089/hum.1997.8.15-1807. [Google Scholar] [PubMed] [CrossRef]
85. Rainov NG. A phase III clinical evaluation of herpes simplex virus type 1 thymidine kinase and ganciclovir gene therapy as an adjuvant to surgical resection and radiation in adults with previously untreated glioblastoma multiforme. Hum Gene Ther. 2000;11(17):2389–401. doi:10.1089/104303400750038499. [Google Scholar] [PubMed] [CrossRef]
86. Wilson KM, Stambrook PJ, Bi WL, Pavelic ZP, Pavelic L, Gluckman JL. HSV-tk gene therapy in head and neck squamous cell carcinoma. Enhancement by the local and distant bystander effect. Arch Otolaryngol Head Neck Surg. 1996;122(7):746–9. doi:10.1001/archotol.1996.01890190042011. [Google Scholar] [PubMed] [CrossRef]
87. Le Rhun E, Preusser M, Roth P, Reardon DA, Van den Bent M, Wen P, et al. Molecular targeted therapy of glioblastoma. Cancer Treat Rev. 2019;80:101896. [Google Scholar] [PubMed]
88. Taylor OG, Brzozowski JS, Skelding KA. Glioblastoma multiforme: an overview of emerging therapeutic targets. Front Oncol. 2019;9:963. [Google Scholar] [PubMed]
89. Chinot OL, Wick W, Mason W, Henriksson R, Saran F, Nishikawa R, et al. Bevacizumab plus radiotherapy-temozolomide for newly diagnosed glioblastoma. N Engl J Med. 2014;370(8):709–22. [Google Scholar] [PubMed]
90. Xie F, Wang M, Chen Q, Chi T, Zhu S, Wei P, et al. Endogenous stimuli-responsive nanoparticles for cancer therapy: from bench to bedside. Pharmacol Res. 2022;186:106522. [Google Scholar] [PubMed]
91. Korovkina O, Polyakov D, Korzhikov-Vlakh V, Korzhikova-Vlakh E. Stimuli-responsive polypeptide nanoparticles for enhanced DNA delivery. Molecules. 2022;27(23):8495. [Google Scholar] [PubMed]
92. Zhou W, Jia Y, Liu Y, Chen Y, Zhao P. Tumor microenvironment-based stimuli-responsive nanoparticles for controlled release of drugs in cancer therapy. Pharmaceutics. 2022;14(11):2346. doi:10.3390/pharmaceutics14112346. [Google Scholar] [PubMed] [CrossRef]
93. Chauhan M, Basu SM, Qasim M, Giri J. Polypropylene sulphide coating on magnetic nanoparticles as a novel platform for excellent biocompatible, stimuli-responsive smart magnetic nanocarriers for cancer therapeutics. Nanoscale. 2023;15(16):7384–402. doi:10.1039/D2NR05218K. [Google Scholar] [PubMed] [CrossRef]
94. Seelam ML, Yarraguntla SR, Paravastu VKK, Vurukuti SS, Mylavarapu SSV. Polymeric nanoparticles with stimuli-responsive properties for drug delivery. GSC Biol Pharm Sci. 2022;20(1):44–55. doi:10.30574/gscbps.2022.20.1.0259. [Google Scholar] [CrossRef]
95. Balkwill FR, Capasso M, Hagemann T. The tumor microenvironment at a glance. J Cell Sci. 2012;125(23):5591–6. doi:10.1242/jcs.116392. [Google Scholar] [PubMed] [CrossRef]
96. Yang S, Gao H. Nanoparticles for modulating tumor microenvironment to improve drug delivery and tumor therapy. Pharmacol Res. 2017;126:97–108. doi:10.1016/j.phrs.2017.05.004. [Google Scholar] [PubMed] [CrossRef]
97. Alas M, Saghaeidehkordi A, Kaur K. Peptide-drug conjugates with different linkers for cancer therapy. J Med Chem. 2021;64(1):216–32. doi:10.1021/acs.jmedchem.0c01530. [Google Scholar] [PubMed] [CrossRef]
98. Chen S, Zhao X, Chen J, Chen J, Kuznetsova L, Wong SS, et al. Mechanism-based tumor-targeting drug delivery system. Validation of efficient vitamin receptor-mediated endocytosis and drug release. Bioconjug Chem. 2010;21(5):979–87. doi:10.1021/bc9005656. [Google Scholar] [PubMed] [CrossRef]
99. Chen J, Chen S, Zhao X, Kuznetsova LV, Wong SS, Ojima I. Functionalized single-walled carbon nanotubes as rationally designed vehicles for tumor-targeted drug delivery. J Am Chem Soc. 2008;130(49):16778–85. doi:10.1021/ja805570f. [Google Scholar] [PubMed] [CrossRef]
100. Dissanayake S, Denny WA, Gamage S, Sarojini V. Recent developments in anticancer drug delivery using cell penetrating and tumor targeting peptides. J Control Release. 2017;250:62–76. doi:10.1016/j.jconrel.2017.02.006. [Google Scholar] [PubMed] [CrossRef]
101. Torchilin V. Tumor delivery of macromolecular drugs based on the EPR effect. Adv Drug Deliv Rev. 2011;63(3):131–5. doi:10.1016/j.addr.2010.03.011. [Google Scholar] [PubMed] [CrossRef]
102. Attia MF, Anton N, Wallyn J, Omran Z, Vandamme TF. An overview of active and passive targeting strategies to improve the nanocarriers efficiency to tumour sites. J Pharm Pharmacol. 2019;71(8):1185–98. [Google Scholar] [PubMed]
103. Radu M, Chernoff J. An in vivo assay to test blood vessel permeability. J Vis Exp. 2013;(73):e50062. doi:10.3791/50062. [Google Scholar] [PubMed] [CrossRef]
104. Bazak R, Houri M, El Achy S, Kamel S, Refaat T. Cancer active targeting by nanoparticles: a comprehensive review of literature. J Cancer Res Clin Oncol. 2015;141(5):769–84. [Google Scholar] [PubMed]
105. Cho K, Wang X, Nie S, Chen Z, Shin DM. Therapeutic nanoparticles for drug delivery in cancer. Clin Cancer Res. 2008;14(5):1310–6. [Google Scholar] [PubMed]
106. Cheng Y, Morshed RA, Auffinger B, Tobias AL, Lesniak MS. Multifunctional nanoparticles for brain tumor imaging and therapy. Adv Drug Deliv Rev. 2014;66:42–57. [Google Scholar] [PubMed]
107. Longden J. Drug targeting. Encycl Cell Biol. 2016;4:264–8. doi:10.1016/B978-0-12-394447-4.40043-X. [Google Scholar] [CrossRef]
108. M.Rabanel J, Aoun V, Elkin I, Mokhtar M, Hildgen P. Drug-loaded nanocarriers: passive targeting and crossing of biological barriers. Curr Med Chem. 2012;19(19):3070–102. [Google Scholar]
109. Kumar Khanna V. Targeted delivery of nanomedicines. ISRN Pharmacol. 2012;2012:571394. [Google Scholar] [PubMed]
110. Patel JK, Patel AP. Passive targeting of nanoparticles to cancer. In: Pathak YV, editor. Surface modification of nanoparticles for targeted drug delivery. Cham, Switzerland: Springer; 2019. p. 125–43. [Google Scholar]
111. Bhatia S. Natural polymer drug delivery systems: nanoparticles, plants, and algae. Cham, Switzerland: Springer; 2016. [Google Scholar]
112. Rani K, Paliwal S. A review on targeted drug delivery: its entire focus on advanced therapeutics and diagnostics. Sch J App Med Sci. 2014;2(1C):328–31. [Google Scholar]
113. Anarjan FS. Active targeting drug delivery nanocarriers: ligands. Nano-Struct Nano-Objects. 2019;19:100370. [Google Scholar]
114. Dadwal A, Baldi A, Kumar Narang R. Nanoparticles as carriers for drug delivery in cancer. Artif Cells Nanomed Biotechnol. 2018;46(sup2):295–305. [Google Scholar] [PubMed]
115. Torchilin VP. Passive and active drug targeting: drug delivery to tumors as an example. Handb Exp Pharmacol. 2010;197:3–53. doi:10.1007/978-3-642-00477-3_1. [Google Scholar] [PubMed] [CrossRef]
116. Yoo J, Park C, Yi G, Lee D, Koo H. Active targeting strategies using biological ligands for nanoparticle drug delivery systems. Cancers. 2019;11(5):640. [Google Scholar] [PubMed]
117. Danhier F, Feron O, Préat V. To exploit the tumor microenvironment: passive and active tumor targeting of nanocarriers for anti-cancer drug delivery. J Control Release. 2010;148(2):135–46. [Google Scholar] [PubMed]
118. Morshed M, Chowdhury EH. Gene delivery and clinical applications. In: Narayan R, editor. Encyclopedia of biomedical engineering. Oxford: Elsevier; 2019. p. 345–51. [Google Scholar]
119. Pearce AK, O’Reilly RK. Insights into active targeting of nanoparticles in drug delivery: advances in clinical studies and design considerations for cancer nanomedicine. Bioconjug Chem. 2019;30(9):2300–11. [Google Scholar] [PubMed]
120. Wang MD, Shin DM, Simons JW, Nie S. Nanotechnology for targeted cancer therapy. Expert Rev Anticancer Ther. 2007;7(6):833–7. [Google Scholar] [PubMed]
121. Marcucci F, Lefoulon F. Active targeting with particulate drug carriers in tumor therapy: fundamentals and recent progress. Drug Discovery Today. 2004;9(5):219–28. [Google Scholar] [PubMed]
122. Mendes M, Sousa JJ, Pais A, Vitorino C. Targeted theranostic nanoparticles for brain tumor treatment. Pharmaceutics. 2018;10(4):181. [Google Scholar] [PubMed]
123. Satapathy MK, Yen TL, Jan JS, Tang RD, Wang JY, Taliyan R, et al. Solid Lipid Nanoparticles (SLNsan advanced drug delivery system targeting brain through BBB. Pharmaceutics. 2021;13(8):1183. [Google Scholar] [PubMed]
Cite This Article
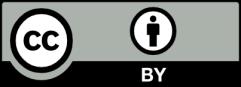
This work is licensed under a Creative Commons Attribution 4.0 International License , which permits unrestricted use, distribution, and reproduction in any medium, provided the original work is properly cited.