Open Access
ARTICLE
TMED3 promotes prostate cancer via FOXO1a and FOXO3a phosphorylation
1 Department of Urology, The People’s Hospital of Guangxi Zhuang Autonomous Region, Nanning, 530000, China
2 Department of Rehabilitation, The People’s Hospital of Guangxi Zhuang Autonomous Region, Nanning, 530000, China
* Corresponding Author: FENGNING WU. Email:
Oncology Research 2025, 33(1), 161-169. https://doi.org/10.32604/or.2024.048054
Received 26 November 2023; Accepted 27 March 2024; Issue published 20 December 2024
Abstract
Background: Transmembrane emp24 trafficking protein 3 (TMED3) is associated with the development of several tumors; however, whether TMED3 regulates the progression of prostate cancer remains unclear. Materials and Methods: Short hairpin RNA was performed to repress TMED3 in prostate cancer cells (DU145 cells) and in a prostate cancer mice model to determine its function in prostate cancer in vitro and in vivo. Results: In the present study, we found that TMED3 was highly expressed in prostate cancer cells. In vitro, shTMED3 treatment suppressed the proliferation, invasion, and migration and promoted the apoptosis of DU145 cells. Additionally, the Kyoto Encyclopedia of Genes and Genomes pathway enrichment analysis showed a strong correlation between TMED3 and forkhead box O transcription factor (FOXO) pathway. Furthermore, TMED3 inhibition efficiently decreased FOXO1a and FOXO3a phosphorylation. In vivo, TMED3 downregulation suppressed the apoptosis, growth, and metastasis of prostate cancer cells via FOXO1a and FOXO3a. Conclusion: The present findings show that TMED3 participates in the regulation of prostate cancer progression via FOXO1a and FOXO3a phosphorylation, thereby revealing a novel mechanism underlying prostate cancer development and suggesting that TMED3 inhibition may serve as a novel strategy for prostate cancer treatment.Keywords
Prostate cancer, the most commonly diagnosed cancer in men, is an androgen-dependent tumor. Androgen can stimulate prostate cancer cell growth and disease progression. Despite many advances in traditional therapies including androgen deprivation therapy and antiandrogen therapy may be useful for early-stage prostate cancer [1–4]. However, the therapeutic effect of such approaches on prostate cancer is limited once the cancer cells metastasize to distant organs. Thus, the elucidation of molecular mechanisms underlying prostate cancer development and progression will help improve treatment strategies against the cancer.
Prostate cancer exhibits characteristics of increased proliferation, migration, and invasion and decreased apoptosis of prostate cancer cells [5–7]. Transmembrane emp24 domain (TMED)-containing proteins have been recognized for their role in pancreatic diseases, acute inflammatory responses, and cancer [8–10]. Emerging evidence has shown that TMED3 is closely related to cancer tumorigenesis progression, proliferation, invasion, and metastasis [11,12]. High-throughput transcriptomics and RNAi analysis in a previous study showed that the mRNA expression of ERGIC1 and TMED3 was upregulated in cultured prostate cancer cells [13]. However, the involvement of TMED3 in the pathophysiological progression of prostate cancer cells and the potential molecular mechanisms remain unclear.
The forkhead box O transcription factor (FOXO) family has recently been highlighted as crucial for diseases [14,15]. FOXO1a and FOXO3a activation plays a protective role in prostate cancer [16,17]. Furthermore, FOXO1a phosphorylation and inhibition contribute to cell proliferation and survival in prostate cancer [16]. Additionally, FOXO3a inhibition enhances the migration of prostate cancer cells and differential regulation of epithelial–mesenchymal transition (EMT) markers [18]. Similarly, blocking FoxO3a activity accelerates prostate cancer progression in vivo [19]. Thus, in the present study, we hypothesize that the TMED3-induced phosphorylation of FOXO1a and FOXO3a may modulate the proliferation, migration, invasion, and apoptosis of prostate cancer cells, thereby causing prostate cancer.
Herein, initially, we examined the proliferation, migration, invasion, and apoptosis of prostate cancer cells after knocking down TMED3 in vitro. Next, we investigated the possible downstream signaling pathway of TMED3 using gene chips and analyzed the causative association of TMED3 and the FOXO pathway by performing the Kyoto Encyclopedia of Genes and Genomes (KEGG) pathway enrichment analysis. Finally, we determined whether TMED3 downregulation alleviated prostate cancer development in vivo. The present results suggest that TMED3 serves as a potential tumor-promoting gene that enhances the proliferation and metastasis of prostate cancer, and TMED3 inhibition may be a useful therapeutic target against prostate cancer.
An apoptosis kit (88–8007) was purchased from eBioscience (CA, USA). The following antibodies were purchased from Abcam (Cambridge, MA, USA): anti-TMED3 (ab223175), anti-phosphorylation FOXO1a (ab131339), anti-phosphorylation FOXO3a (ab154786), anti-laminB (ab133741), anti-Ki67 (ab16667), and anti-GAPDH (ab8245). The CCK8 kit (96992) and antibody-propidiumiodide (PI) (P4170) were obtained from Sigma-Aldrich (St. Louis, USA). Hematoxylin and eosin were obtained from Solarbio Science & Technology (Beijing, China).
The human prostate cancer cell line DU145 without mycoplasma was obtained from Procell Life Science & Technology Co., Ltd. DU145 cells were cultured using DMEM with 10% FBS, penicillin and streptomycin under 5% CO2 at 37 °C. To silence TMED3 in DU145, the cells were seeded in 6-well plates and infected with a lentivirus containing TMED3 short hairpin RNA (shRNA) (shTMED3 group) target sequences. A negative control (shCtrl group) was also set. The cells were then cultured at 37°C for 72 h. The recombinant lentivirus plasmid targeting shTMED3-1 (CTCTCACAAGACCGTCTACTT), shTMED3-2 (CACCTTCGAGCTGCCGGACA-A), shTMED3-3 (CGTGAAGTTCTCCCTGGATTA), and the control shRNA (ACGACGTCAGCTGGTGCATGT) were developed.
Animal treatment and in vivo imaging
Four-week-old specific pathogen free male BALB/c mice were purchased from GemPharmatech Co., Ltd. (China). All procedures performed in the study involving animals were in compliance with the ARRIVE guidelines. To establish a prostate cancer model in vivo, DU145 cells (1 × 107) were subcutaneously inoculated into the mice (shCtrl group). Further, to investigate the protective effect of TMED3 inhibition on prostate cancer metastasis, the mice were subcutaneously injected with shTMED3 (shTMED3 group) on day 10 after DU145 cell inoculation. Next, tumor tissues were collected on day 23 after DU145 cell inoculation. Hematoxylin and eosin (HE) staining was performed to observe morphological alterations in prostate tumors, and the IVIS Spectrum animal imaging system (LB983, Berthold Technologies, Belgium) was used to evaluate tumor growth and whole metastasis conditions after administering 100 μL of XenoLight D-luciferin Potassium Salt (15 mg/mL, Perkin Elmer, USA) to each mouse on day 23. All procedures on the mice followed the guidelines for humane treatment by the People’s Hospital of Guangxi Zhuang Autonomous Region (Number: K-Y-LW-2022-04).
DU145 cells were seeded in 96-well plates and infected with a lentivirus at different time points. Afterward, 10 μL of the CCK-8 solution was supplemented to each well, followed by incubation for 1 h at 37°C. Absorbance was measured at 450 nm using a microplate reader.
To determine the cell cycle and apoptosis of DU145 cells, we examined the positive expression of Annexin V and PI in DU145 cells by FCM. Briefly, DU145 cells were incubated with 10% fetal bovine serum (FBS). Then, the cells were incubated with the PI/RNase solution or phycoerythrin-PI and fluorescein isothiocyanate (FITC)-Annexin V for 30 min at 4°C in the dark. The cell apoptosis (early, late, and total apoptosis) and cell cycle (G0/G1, S, and G2/M phase) of DU145 cells were examined using a flow cytometer (Beckman Coulter, USA). PI−Annexin V+ cells were defined as cells at the early stage of apoptosis, whereas PI+Annexin V+ cells were defined as cells at the late stage of apoptosis.
Migration and wound healing assays
After lentivirus infection, DU145 cells were seeded into the Transwell plates (upper chamber), and FBS was added to the lower chamber as a chemoattractant. After 24 h, the invading cells were stained with the 0.1% crystal violet staining solution and observed under a light microscope (Olympus, Tokyo, Japan). Cells infected with shTMED3 or shCtrl were scratched, wound healing was monitored, and the migration distance was imaged 24 h after scratch treatment.
Prostate tumors and cells were homogenized for isolating total proteins, which were separated by sodium dodecyl sulfate–polyacrylamide gel electrophoresis and transferred to membranes. After blocking, the membranes were stained with anti-TMED3 (1:2000), anti-p-FOXO1a (1:2000), anti-FOXO1a (1:2000), anti-pFOXO3a (1:2000), anti-FOXO3a (1:2000), anti-laminB (1:2000), and anti-GAPDH (1:2000) antibodies, followed by staining with a secondary antibody (1:5000) and enhanced ECL detection kit.
Real-time quantitative reverse transcriptase polymerase chain reaction (qRT-PCR)
Total RNA was extracted from the cells using the TRIzol reagent, and the extracted RNA was used to synthesize cDNA using the Promega M-MLV kit. qRT-PCR was performed using Light Cycler 480 SYBR Green I Master Mix, as described previously [20]. Primers used for qRT-PCR are listed in Suppl. Table 1.
The tumor tissues were harvested and fixed using 4% neutral buffered formalin before stepwise dehydration and embedded in paraffin. Then, the samples were stained with HE to observe morphological alterations under a light microscope (Olympus, Tokyo, Japan).
The tumor tissue sections were deparaffinized and incubated in mixed phosphate-buffered saline containing 0.1% Triton X-100 and 3% H2O2 in the dark. After performing antigen retrieval, the sections were blocked with 5% normal goat serum and stained with anti-ki67 at 4°C overnight. The sections were incubated with the correspondent horseradish peroxidase-conjugated secondary antibodies, followed by DAB reactions and HE staining. Cells with brownish-yellow particles were considered ki67-positive cells.
For immunofluorescence staining, the slides were blocked, and anti-p-FOXO1a or anti-p-FOXO3a antibodies were directly added to the samples, followed by overnight incubation. Next, the samples were incubated with FITC-conjugated secondary antibodies. Finally, the slides were observed under a fluorescence microscope after nucleus staining.
The data are presented as the mean ± standard deviation. t-test and one-way analysis of variance were performed to statistically analyze the data. Prostate cancer gene expression data were obtained from The Cancer Genome Atlas (TCGA) database. Visualization and Integrated Discovery were used to perform KEGG pathway enrichment analysis. p < 0.05 was considered statistically significant.
Knockdown of TMED3 suppressed proliferation and promoted apoptosis in prostate cancer cells
The silencing efficiency of shTMED3-3 was the most potent among the three shTMED3s (Fig. 1A). The western blotting results showed that the levels of the TMED3 protein decreased significantly after shTMED3-3 intervention (Fig. 1B). Hence, we selected shTMED3-3 to inhibit TMED3 in DU145 cells in subsequent in vitro experiments.
Figure 1: The silencing efficiency of shTMED3. (A) DU145 cells were treated with shTMED3-1, shTMED3-2, and shTMED3-3 for 72 h and subjected to qRT-PCR. Relative TMED3 mRNA expression in DU145 was analyzed. (B) DU145 cells were treated with shTMED3-3 for 72 h and subjected to western blotting. The TMED3 protein level in DU145 was determined. *p < 0.05; **p < 0.01.
First, we observed the levels of the TMED3 protein in normal human prostate cells (RWPE-1 cells) and prostate cancer cells (DU145, PC-3, and LNCap cells). The results showed that TMED3 was more highly expressed in PC-3, LNCap, and DU145 cells than in RWPE-1 cells (Fig. 2A). Thus, the results indicated that TMED3 may play a crucial role in prostate cancer progression.
Figure 2: TMED3 regulated proliferation and promoted apoptosis in DU145 cells. (A) TMED3 protein levels in normal human prostate cells and prostate cancer cells were determined by western blotting. DU145 cells were infected with a lentivirus to silence TMED3 expression, and shCtrl was used as a scrambled control. (B) Cytotoxicity at different time points was determined by performing the CCK-8 assay. (C) A representative scatter plot of PI+Annexin V+ and PI−Annexin V+ DU145 cells by flow cytometry. (D) The proportions of DU145 cells at different stages of apoptosis (early apoptosis, late apoptosis, and total apoptosis) by flow cytometry. (E and F) The cell cycle (G1, S, and G2 phases) of DU145 cells was determined by flow cytometry. **p < 0.01; ***p < 0.001.
CCK-8 and FCM assays were performed to investigate the effect of TMED3 inhibition on prostate cancer cell growth. shTMED3 reduced cell growth in a time-dependent manner, and it decreased markedly on day 5 after shTMED3 intervention compared with that in the shCtrl group (Fig. 2B). The FCM results showed that the proportions of PI+Annexin V+ and PI−Annexin V+ cells in the shTMED3 group were higher than those in the shCtrl group (Figs. 2C and 2D). Additionally, TMED3 knockdown decreased the cell percentage at the G1 phase (Figs. 2E and 2F). These results indicated that TMED3 promoted cell proliferation and inhibited apoptosis in prostate cancer.
Knockdown of TMED3 inhibited the invasion and migration of prostate cancer cells
We investigated alterations in the invasion and migration of prostate cancer cells induced by shTMED3. The invasion capacity of prostate cancer cells was suppressed by shTMED3 (Figs. 3A and 3B). Moreover, prostate cancer cells transfected with shTMED3 exhibited lower migration activity than did the control cells (Figs. 3C and 3D). Collectively, these results suggested that TMED3 knockdown could be an important defense mechanism against prostate cancer development.
Figure 3: TMED3 regulated the invasion and migration of prostate cancer cells. DU145 cells were infected with a lentivirus to silence TMED3 expression, and shCtrl was used as a scrambled control. (A and B) The wound-healing assay was performed to determine the invasion ability of DU145 cells. (C and D) DU145 cell migration was assessed by performing the Transwell assay. ***p < 0.001. Original magnification, 200×.
The correlation of the FOXO signaling pathway and TMED3 during the progression of prostate cancer
To investigate the potential molecular mechanism underlying the role of TMED3 in prostate cancer, we used the TCGA database. We identified 26 signaling pathways that were correlated to TMED3 mRNA levels. Among them, the FOXO pathway plays a protective role in prostate cancer, and a strong correlation was observed between TMED3 and the FOXO pathway (Figs. 4A and 4B). Thus, we focused on the potential cascade correlation between TMED3 and the FOXO pathway.
Figure 4: TMED3 inhibited the FOXO pathway by inducing FOXO1a and FOXO3a phosphorylation during prostate cancer progression. (A) The enrichment analysis of the TCGA data was performed to analyze the correlation of TMED3 with 26 signaling pathways. The sizes of the circles indicate the significance of enrichment, whereas their colors represent the strength and direction of the enrichment (red: positive correlation, blue: negative correlation). (B) The key role of the FOXO family in cancer-related pathways. (C) Western blotting of FOXO1a, p-FOXO1a, FOXO3a, and p-FOXO3a in DU145 cells.
To better determine whether the marked TMED3 upregulation was a driving cause for FOXO signaling pathway activation or a correlative hallmark during prostate cancer progression, TMED3 was downregulated by injecting shTMED3 in vivo. TMED3 protein levels in the tumor tissues decreased significantly after shTMED3 administration in vivo. Furthermore, TMED3 silencing decreased FOXO1a and FOXO3a phosphorylation (Fig. 4C). Thus, the results indicated that TMED3 inhibited the FOXO pathway by inducing FOXO1a and FOXO3a phosphorylation during prostate cancer progression.
TMED3 downregulation suppressed prostate cancer progression by decreasing FOXO1a and FOXO3a phosphorylation in vivo
We investigated whether TMED3 downregulation alleviated prostate cancer in mice. TMED3 downregulation greatly inhibited prostate tumor growth in the mice (Figs. 5A and 5B). Next, we observed prostate cancer cell metastasis using an in vivo imaging system. Mice in the shCtrl group showed prostate cancer metastasis (Fig. 5C). However, compared with mice injected with shCtrl cells, mice injected with TMED3-downregulated prostate cancer cells showed lower prostate cancer metastasis in vivo on day 23 after the treatment. The histological analysis indicated that mice receiving shTMED3 showed a lower number of prostate cancer foci than did the mice in the shCtrl group (Fig. 5D). The IHC results showed that TMED3-downregulated mice exhibited a lower percentage of Ki67+ tumor cells than did mice in the shCtrl group, which implied that TMED3 was associated with the modulatory process of apoptosis in prostate cancer (Fig. 5E). Based on this mouse model of prostate cancer, we confirmed that TMED3 downregulation decreased p-FOXO1a and p-FOXO3a levels rather than FOXO1a and FOXO3a levels (Fig. 6). These findings support the notion that the TMED3-regulated phosphorylation of FOXO1a and FOXO3a was involved in prostate cancer progression.
Figure 5: TMED3 downregulation alleviated prostate cancer in vivo. (A and B) The sizes of prostate tumors in mice. (C) Prostate cancer metastasis in mice was evaluated using an in vivo imaging system. (D) Histological alterations in prostate tumors were observed by performing HE staining. (E) Immunohistochemistry of ki67-positive expression in prostate tumors of mice. Original magnification, 400×.
Figure 6: TMED3 downregulation decreased p-FOXO1 and p-FOXO3 levels in a mouse model of prostate cancer. (A) Western blotting of FOXO1a, p-FOXO1a, FOXO3a, and p-FOXO3a in tumor tissues. Immunofluorescence analysis of p-FOXO1a (B) and p-FOXO3a (C) in tumor tissues. Original magnification, 200×.
In this study, we found that TMED3 protein levels increased in prostate cancer cells, and TMED3 knockdown inhibited the proliferation, migration, and invasion and promoted the apoptosis of prostate cancer cells in vitro. The KEGG pathway enrichment analysis revealed a strong correlation between TMED3 and FOXO signaling pathway phosphorylation. Additional experiments confirmed that the TMED3-induced phosphorylation of FOXO1a and FOXO3a played a role in prostate cancer progression. The in vivo experiments showed that TMED3 downregulation suppressed prostate cancer progression in mice. These findings provide novel evidence that TMED3 downregulation is a promising strategy to counteract tumor cell proliferation, migration, and invasion.
Accumulating evidence shows that TMED3 is a newly identified cancer-related protein in several malignancies, such as breast cancer, gastric cancer, chordoma, and lung cancer. TMED3 exerts antitumor effects by downregulating Wnt/β-catenin signaling via AKTO regulation [21]. Zhang et al. reported that TMED3 enhanced cell viability and migration and inhibited apoptosis in chordoma cells by greatly silencing TMED3 expression and activation, suggesting that TMED3 may be a novel target for tumor therapy [11]. Guo et al. found that TMED3 protein levels were high in malignant melanoma; thus, they identified TMED3 as a crucial tumor-promoting gene in malignant melanoma progression [22]. However, evidence on the role of TMED3 in prostate cancer is lacking. Herein, we compared TMED3 protein levels between normal human prostate cells and prostate cancer cells and found its higher levels in prostate cancer cells than in normal cells. Tissue homeostasis is maintained through a balance of proliferative and antiproliferative signals, and tumor cell migration and invasion are considered key indicators to evaluate the capacity of tumor metastasis [23–27]. Herein, we silenced TMED3 expression both in vitro and in vivo. The in vitro results showed that TMED3 knockdown in prostate cancer cells inhibited the proliferation, migration, and invasion and promoted the apoptosis of prostate cancer cells. The in vivo results showed that TMED3 downregulation suppressed the apoptosis of prostate tumor cells and alleviated prostate cancer. The present findings imply that TMED3 is a novel inducer of prostate cancer progression, and its downregulation may hold prognostic value for metastasis.
TMED3 is involved in multiple pathways that may contribute to prostate cancer development [8,28,29]. Herein, the KEGG pathway enrichment analysis was performed to investigate the possible downstream cooperator of TMED3 in prostate cancer, and a strong correlation between TMED3 and FOXO signaling pathway phosphorylation was observed. FOXO is an important downstream effector and transcriptional regulator associated with tumor metastasis [30–32]. Growing evidence shows that FOXO proteins, including FOXO1a and FOXO3a, suppress tumors via being regulated by oncogenic proteins such as Akt, inhibitor of nuclear factor-κB kinase, and S-phase kinase-associated protein 2 [33–35]. Boudjadi et al. demonstrated that fibroblast growth factor 8, a direct transcriptional target of paired box gene 3 (PAX3)–FOXO1, was responsible for PAX3–FOXO1-independent tumor recurrence via an autocrine mechanism, implying the crucial role of FOXO1 in tumor metastasis [36]. Liu et al. reported that FOXO1 phosphorylation regulated by cyclin-dependent kinase 1 played a role in tumorigenesis by promoting cell proliferation and survival [16]. Additionally, FOXO3a modulated Wnt/β-catenin signaling and suppressed EMT in prostate cancer cells [18]. Therefore, we hypothesized that the TMED3-induced phosphorylation of FOXO1a and FOXO3a may regulate the proliferation, migration, invasion, and apoptosis of prostate cancer cells. Herein, we confirmed that FOXO1a and FOXO3a phosphorylation was regulated by TMED3. High TMED3 expression resulted in the loss of function of FOXO1a and FOXO3a owing to S249 phosphorylation, thereby promoting prostate cancer development.
Nevertheless, this study has several limitations that need further investigation. The reason behind TMED3 activation in prostate cancer was not clarified. Thus, future studies are warranted to investigate whether FOXO signaling pathway activation alleviates prostate cancer.
We demonstrated that TMED3 phosphorylated FOXO1a and FOXO3a, thereby contributing to the loss of their transcriptional activities. TMED3 inhibition suppressed prostate cancer cell metastasis in vivo and in vitro. The present findings suggested that TMED3 inhibition may serve as a potent therapeutic target against prostate cancer progression.
Acknowledgement: Not applicable.
Funding Statement: This work was supported by Guangxi Medical and Health Appropriate Technology Development and Promotion Application Project (S2022022).
Author Contributions: Xiuwang Wei and Fengning Wu designed the research; Xiuwang Wei, Jianbo Liang, Huanwen Huang, Daming Yang, Xinxin Wang and Xiujia Wang performed experiments; Changsheng Chen and Kaiqiang Li analyzed data; Taiseng Pang wrote the manuscript; Bin Hu revised the manuscript.
Availability of Data and Materials: The data that support the findings of this study are available on request from the corresponding author upon reasonable request.
Ethics Approval: All procedures on the mice followed the guidelines for humane treatment by the People’s Hospital of Guangxi Zhuang Autonomous Region (Number: K-Y-LW-2022-04).
Conflicts of Interest: The authors declare no conflicts of interest to report regarding the present study.
References
1. Culig, Z., Santer, F. R. (2014). Androgen receptor signaling in prostate cancer. Cancer Metastasis Reviews, 33(2–3), 413–427. https://doi.org/10.1007/s10555-013-9474-0. [Google Scholar] [PubMed] [CrossRef]
2. Green, S. M., Mostaghel, E. A., Nelson, P. S. (2012). Androgen action and metabolism in prostate cancer. Molecular and Cellular Endocrinology, 360(1–2), 3–13. https://doi.org/10.1016/j.mce.2011.09.046. [Google Scholar] [PubMed] [CrossRef]
3. Nguyen-Nielsen, M., Borre, M. (2016). Diagnostic and therapeutic strategies for prostate cancer. Seminars in Nuclear Medicine, 46(6), 484–490. https://doi.org/10.1053/j.semnuclmed.2016.07.002. [Google Scholar] [PubMed] [CrossRef]
4. Zhou, L., Song, Z., Hu, J., Liu, L., Hou, Y. et al. (2021). ACSS3 represses prostate cancer progression through downregulating lipid droplet-associated protein PLIN3. Theranostics, 11(2), 841–860. https://doi.org/10.7150/thno.49384. [Google Scholar] [PubMed] [CrossRef]
5. Gupta, P., Serajuddin, M. (2021). Fish lipid against prostate cancer (PC-3) through apoptosis and cell cycle arrest. Nutrition and Cancer, 73(2), 300–306. https://doi.org/10.1080/01635581.2020.1743872. [Google Scholar] [PubMed] [CrossRef]
6. Shen, Z., Zhou, L., Zhang, C., Xu, J. (2020). Reduction of circular RNA Foxo3 promotes prostate cancer progression and chemoresistance to docetaxel. Cancer Letters, 468, 88–101. https://doi.org/10.1016/j.canlet.2019.10.006. [Google Scholar] [PubMed] [CrossRef]
7. Sun, L., Lü, J., Ding, S., Bi, D., Ding, K. et al. (2017). HCRP1 regulates proliferation, invasion, and drug resistance via EGFR signaling in prostate cancer. Biomedicine & Pharmacotherapy, 91, 202–207. https://doi.org/10.1016/j.biopha.2017.04.040. [Google Scholar] [PubMed] [CrossRef]
8. Duquet, A., Melotti, A., Mishra, S., Malerba, M., Seth, C. et al. (2014). A novel genome-wide in vivo screen for metastatic suppressors in human colon cancer identifies the positive WNT-TCF pathway modulators TMED3 and SOX12. EMBO Molecular Medicine, 6(7), 882–901. https://doi.org/10.15252/emmm.201303799. [Google Scholar] [PubMed] [CrossRef]
9. Jenne, N., Frey, K., Brugger, B., Wieland, F. T. (2002). Oligomeric state and stoichiometry of p24 proteins in the early secretory pathway. The Journal of Biological Chemistry, 277(48), 46504–46511. https://doi.org/10.1074/jbc.M206989200. [Google Scholar] [PubMed] [CrossRef]
10. Strating, J. R., Martens, GJ. (2009). The p24 family and selective transport processes at the ER-Golgi interface. Biology of the Cell, 101(9), 495–509. https://doi.org/10.1042/BC20080233. [Google Scholar] [PubMed] [CrossRef]
11. Zhang, X., Luo, Y., Li, Q. (2020). TMED3 promotes proliferation and migration in breast cancer cells by activating Wnt/β-catenin signaling. OncoTargets and Therapy, 13, 5819–5830. https://doi.org/10.2147/OTT.S250766. [Google Scholar] [PubMed] [CrossRef]
12. Zheng, H., Yang, Y., Han, J., Jiang, W. H., Chen, C. et al. (2016). TMED3 promotes hepatocellular carcinoma progression via IL-11/STAT3 signaling. Scientific Reports, 6, 37070. https://doi.org/10.1038/srep37070. [Google Scholar] [PubMed] [CrossRef]
13. Vainio, P., Mpindi, J. P., Kohonen, P., Fey, V., Mirtti, T. et al. (2012). High-throughput transcriptomic and RNAi analysis identifies AIM1, ERGIC1, TMED3 and TPX2 as potential drug targets in prostate cancer. PLoS One, 7(6), e39801. https://doi.org/10.1371/journal.pone.0039801. [Google Scholar] [PubMed] [CrossRef]
14. Khor, Y. S., Wong, P. F. (2024). MicroRNAs-associated with FOXO3 in cellular senescence and other stress responses. Biogerontology, 25(1), 23–51. https://doi.org/10.1007/s10522-023-10059-6. [Google Scholar] [PubMed] [CrossRef]
15. Vyavahare, S., Kumar, S., Smith, K., Mendhe, B., Zhong, R. et al. (2023). Inhibiting MicroRNA-141-3p improves musculoskeletal health in aged mice. Aging and Disease, 14(6), 2303–2316. https://doi.org/10.14336/AD.2023.0310-1. [Google Scholar] [PubMed] [CrossRef]
16. Liu, P., Kao, T. P., Huang, H. (2008). CDK1 promotes cell proliferation and survival via phosphorylation and inhibition of FOXO1 transcription factor. Oncogene, 27(34), 4733–4744. https://doi.org/10.1038/onc.2008.104. [Google Scholar] [PubMed] [CrossRef]
17. Shukla, S., Bhaskaran, N., Maclennan, G. T., Gupta, S. (2013). Deregulation of FoxO3a accelerates prostate cancer progression in TRAMP mice. Prostate, 73(14), 1507–1517. https://doi.org/10.1002/pros.22698. [Google Scholar] [PubMed] [CrossRef]
18. Liu, H., Yin, J., Wang, H., Jiang, G., Deng, M. et al. (2015). FOXO3a modulates WNT/β-catenin signaling and suppresses epithelial-to-mesenchymal transition in prostate cancer cells. Cellular Signalling, 27(3), 510–518. https://doi.org/10.1016/j.cellsig.2015.01.001. [Google Scholar] [PubMed] [CrossRef]
19. Kong, Z., Deng, T., Zhang, M., Zhao, Z., Liu, Y. et al. (2018). β-arrestin1-medieated inhibition of FOXO3a contributes to prostate cancer cell growth in vitro and in vivo. Cancer Science, 109(6), 1834–1842. https://doi.org/10.1111/cas.13619. [Google Scholar] [PubMed] [CrossRef]
20. Zhang, X., Hu, X., Zhang, Y., Liu, B., Pan, H. et al. (2023). Impaired autophagy-accelerated senescence of alveolar type II epithelial cells drives pulmonary fibrosis induced by single-walled carbon nanotubes. Journal of Nanobiotechnology, 21(1), 69. https://doi.org/10.1186/s12951-023-01821-6. [Google Scholar] [PubMed] [CrossRef]
21. Zhang, D., Sun, L., Zhang, J. (2021). TMED3 exerts a protumor function in non-small cell lung cancer by enhancing the Wnt/β-catenin pathway via regulation of AKT. Toxicology and Applied Pharmacology, 433, 115793. https://doi.org/10.1016/j.taap.2021.115793. [Google Scholar] [PubMed] [CrossRef]
22. Guo, X., Yin, X., Xu, Y., Li, L., Yuan, M. et al. (2023). TMED3 promotes the development of malignant melanoma by targeting CDCA8 and regulating PI3K/Akt pathway. Cell & Bioscience, 13(1), 65. https://doi.org/10.1186/s13578-023-01006-6. [Google Scholar] [PubMed] [CrossRef]
23. Dai, X., Liang, Z., Liu, L., Guo, K., Xu, S. et al. (2019). Silencing of MALAT1 inhibits migration and invasion by sponging miR-1-3p in prostate cancer cells. Molecular Medicine Reports, 20(4), 3499–3508. https://doi.org/10.3892/mmr.2019.10602. [Google Scholar] [PubMed] [CrossRef]
24. Eerola, S. K., Santio, N. M., Rinne, S., Kouvonen, P., Corthals, G. L. et al. (2019). Phosphorylation of NFATC1 at PIM1 target sites is essential for its ability to promote prostate cancer cell migration and invasion. Cell Communication and Signaling, 17(1), 148. https://doi.org/10.1186/s12964-019-0463-y. [Google Scholar] [PubMed] [CrossRef]
25. Jiang, C., Zhang, N., Hu, X., Wang, H. (2021). Tumor-associated exosomes promote lung cancer metastasis through multiple mechanisms. Molecular Cancer, 20(1), 117. https://doi.org/10.1186/s12943-021-01411-w. [Google Scholar] [PubMed] [CrossRef]
26. Ma, L., He, H., Jiang, K., Jiang, P., He, H. et al. (2020). FAM46C inhibits cell proliferation and cell cycle progression and promotes apoptosis through PTEN/AKT signaling pathway and is associated with chemosensitivity in prostate cancer. Sedentary Life and Nutrition, 12(7), 6352–6369. https://doi.org/10.18632/aging.103030. [Google Scholar] [PubMed] [CrossRef]
27. Townson, J. L., Naumov, G. N., Chambers, A. F. (2003). The role of apoptosis in tumor progression and metastasis. Current Molecular Medicine, 3(7), 631–642. https://doi.org/10.2174/1566524033479483. [Google Scholar] [PubMed] [CrossRef]
28. Pei, J., Zhang, S., Yang, X., Han, C., Pan, Y. et al. (2021). Long non-coding RNA RP11-283G6.5 confines breast cancer development through modulating miR-188-3p/TMED3/Wnt/β-catenin signalling. RNA Biology, 18(sup1), 287–302. https://doi.org/10.1080/15476286.2021.1941608. [Google Scholar] [PubMed] [CrossRef]
29. Shorning, B. Y., Dass, M. S., Smalley, M. J., Pearson, H. B. (2020). The PI3K-AKT-mTOR pathway and prostate cancer: At the crossroads of AR, MAPK, and WNT signaling. International Journal of Molecular Sciences, 21(12), 4507. https://doi.org/10.3390/ijms21124507. [Google Scholar] [PubMed] [CrossRef]
30. Du, W. W., Fang, L., Yang, W., Wu, N., Awan, F. M. et al. (2017). Induction of tumor apoptosis through a circular RNA enhancing Foxo3 activity. Cell Death and Differentiation, 24(2), 357–370. https://doi.org/10.1038/cdd.2016.133. [Google Scholar] [PubMed] [CrossRef]
31. Wang, Y., Lyu, Z., Qin, Y., Wang, X., Sun, L. et al. (2020). FOXO1 promotes tumor progression by increased M2 macrophage infiltration in esophageal squamous cell carcinoma. Theranostics, 10(25), 11535–11548. https://doi.org/10.7150/thno.45261. [Google Scholar] [PubMed] [CrossRef]
32. Watkins, S. K., Zhu, Z., Riboldi, E., Shafer-Weaver, K. A., Stagliano, K. E. et al. (2011). FOXO3 programs tumor-associated DCs to become tolerogenic in human and murine prostate cancer. The Journal of Clinical Investigation, 121(4), 1361–1372. https://doi.org/10.1172/JCI44325. [Google Scholar] [PubMed] [CrossRef]
33. Brunet, A., Bonni, A., Zigmond, M. J., Lin, M. Z., Juo, P. et al. (1999). Akt promotes cell survival by phosphorylating and inhibiting a Forkhead transcription factor. Cell, 96(6), 857–868. https://doi.org/10.1016/s0092-8674(00)80595-4. [Google Scholar] [PubMed] [CrossRef]
34. Hu, M. C., Lee, D. F., Xia, W., Golfman, L. S., Ou-Yang, F. et al. (2004). IkappaB kinase promotes tumorigenesis through inhibition of forkhead FOXO3a. Cell, 117(2), 225–237. https://doi.org/10.1016/s0092-8674(04)00302-2. [Google Scholar] [PubMed] [CrossRef]
35. Huang, H., Cheville, J. C., Pan, Y., Roche, P. C., Schmidt, L. J. et al. (2001). PTEN induces chemosensitivity in PTEN-mutated prostate cancer cells by suppression of Bcl-2 expression. The Journal of Biological Chemistry, 276(42), 38830–38836. https://doi.org/10.1074/jbc.M103632200. [Google Scholar] [PubMed] [CrossRef]
36. Boudjadi, S., Pandey, P. R., Chatterjee, B., Nguyen, T. H., Sun, W. et al. (2021). A fusion transcription factor-driven cancer progresses to a fusion-independent relapse via constitutive activation of a downstream transcriptional target. Cancer Research, 81(11), 2930–2942. https://doi.org/10.1158/0008-5472.CAN-20-1613. [Google Scholar] [PubMed] [CrossRef]
Supplementary Materials
Cite This Article
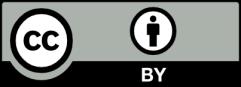
This work is licensed under a Creative Commons Attribution 4.0 International License , which permits unrestricted use, distribution, and reproduction in any medium, provided the original work is properly cited.