Open Access
ARTICLE
TonEBP expression is essential in the IL-1β–induced migration and invasion of human A549 lung cancer cells
Department of Physiology, School of Medicine, Chungnam National University, Daejeon, 35015, Korea
* Corresponding Author: SANG DO LEE. Email:
Oncology Research 2024, 32(1), 151-161. https://doi.org/10.32604/or.2023.030690
Received 20 April 2023; Accepted 19 July 2023; Issue published 15 November 2023
Abstract
Lung cancer has the highest mortality rate among all cancers, in part because it readily metastasizes. The tumor microenvironment, comprising blood vessels, fibroblasts, immune cells, and macrophages [including tumor-associated macrophages (TAMs)], is closely related to cancer cell growth, migration, and invasion. TAMs secrete several cytokines, including interleukin (IL)-1β, which participate in cancer migration and invasion. p21-activated kinase 1 (PAK1), an important signaling molecule, induces cell migration and invasion in several carcinomas. Tonicity-responsive enhancer-binding protein (TonEBP) is also known to participate in cancer cell growth, migration, and invasion. However, the mechanisms by which it increases lung cancer migration remain unclear. Therefore, in this study, we aimed to elucidate the mechanisms by which IL-1β and TonEBP affect lung cancer cell migration and invasion. We found that A549 cocultured-MΦ-secreted IL-1β induced A549 cell migration and invasion via the PAK1 pathway. TonEBP deficiency reduced A549 cell migration and invasion and increased responsiveness to IL-1β–induced migration and invasion. PAK1 phosphorylation, which was promoted by IL-1β, was reduced when TonEBP was depleted. These results suggest that TonEBP plays an important role in IL-1β induction and invasiveness of A549 cells via the PAK1 pathway. These findings could be valuable in identifying potential targets for lung cancer treatment.Graphic Abstract
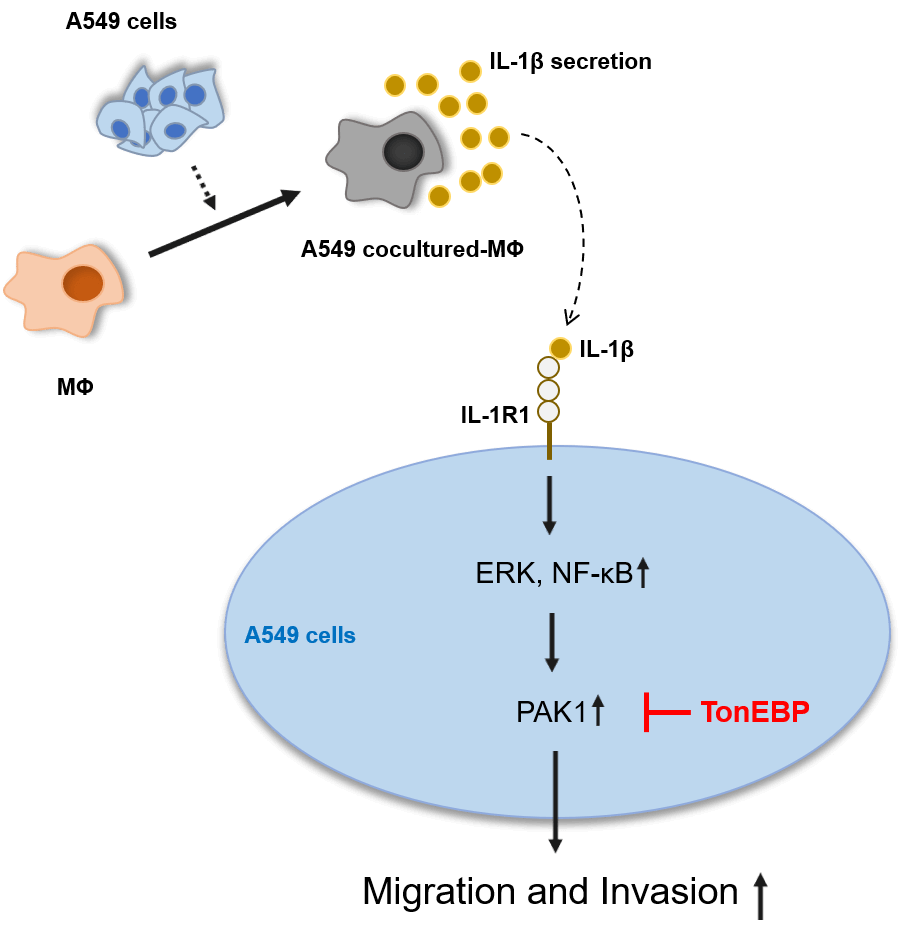
Keywords
Abbreviations
NSCLC | Non-small cell lung cancer |
TAMs | Tumor-associated macrophages |
TME | Tumor microenvironment |
MΦ | Macrophage |
IL-1β | Interleaukine-1 beta |
TonEBP | Tonicity-responsive enhancer-binding protein |
PAK1 | p21-activated kinase 1 |
JNK | c-Jun N-terminal kinase |
NF-κB | Nuclear factor kappa B |
Lung cancer has the highest mortality rate among all cancers [1–3], primarily because the early symptoms are insignificant, making diagnosis difficult, and because it rapidly develops drug resistance and readily metastasizes. Among the two types of lung cancer, non-small cell lung cancer (NSCLC) and small cell lung cancer [1,2], adenocarcinoma (an NSCLC), shows the highest mortality rate.
The tumor microenvironment (TME) is a major contributor of tumor progression or metastasis and consist of immune cells, blood vessels, fibroblasts, and macrophages that surround tumors [4–6]. In the TME, various cell types such as tumor-associated macrophages (TAMs), cancer associated fibroblasts (CAFs), and tumor endothelial cells (TECs) are found, and these cells induce cancer cell proliferation, migration, and angiogenesis [7]. It is well known that CAFs in the TME play an important role in forming tumors by reducing apoptosis and inducing proliferation and migration of cancer cells, whereas TECs are known to aggressively change cancer cells by supplying nutrients that induce tumor angiogenesis [7–9].
Cancer cells and macrophages (MΦ) share many cytokines and growth factors that promote cancer cell aggressiveness [10,11]. TAMs, which secrete several cytokines, chemokines, and growth factors [5,12], worsen cancer prognosis by inducing cancer cell migration, invasion, angiogenesis, and anticancer-drug resistance [13]. CD163, TGF-β, CCL2, and CCL5 are known as TAM biomarkers in lung cancer. TAMs constitute the predominant cellular component in the TME of lung cancer [14]. They not only act as immunosuppressive cells enabling immune evasion of NSCLC but also directly contribute to cancer cell proliferation, survival, invasion, and metastasis. TAMs induce tumor cell progression, resulting in poor prognosis for patients, and for NSCLC patients in particular [15].
The interleukin family (IL) influences metastasis and growth of lung cancer. It has been found that the expression of IL-10 correlates with the tumor diameter of NSCLC patients [16]. In addition, studies have shown that IL-6 and TNF-α promote metastasis of NSCLC by inducing epithelial-mesenchymal transition (EMT) [17]. The IL-1 family includes IL-1β, which induces cancer migration and invasion, promotes cancer growth and metastasis, and is secreted by TAMs, fibroblasts, immune cells, and cancer cells [18–20]. Immune-cell production of IL-1β has been widely studied [21,22]. IL-1β affects EMT, the basis of migration and invasion in several carcinomas [23–25]. In NSCLC, an IL 1β-treated group showed increased EMT along with high expression of the transcription factor SLUG, which is required for EMT [26,27]. In addition, high expression of IL-1β is associated with low survival rates in lung cancer patients [28,29]. In mice, IL-1R deficiency, which binds IL-1β, reduces prostate cancer cell growth and metastasis [30].
Cytokines play important roles in inducing cancer cell migration and invasion. Those that participate in migration and invasion-related signal transduction pathways include p21-activated kinase 1 (PAK1), extracellular signal-regulated kinase [31], c-Jun N-terminal kinase, p38, nuclear factor kappa B (NF-κB), and AKT serine/threonine kinase (AKT) [18,20,32–34]. PAK1, downstream of Rac1 and cdc42 in the Rho family, induces actin polymerization to regulate basic cell migration [31,35] and is associated with healthy cells as well as with cancer growth, migration, and invasion [35–37].
Tonicity-responsive enhancer-binding protein (TonEBP), also known as NFAT5, is a transcription factor in the Rel family of NF-κB and NFAT1-4 proteins [38–41]. It participates in regulating cellular homeostasis in relation to osmotic pressure, its expression is elevated under inflammation, and it is associated with cancer growth [41–43]. TonEBP participates in regulating cancer; clinical studies have revealed that high TonEBP expression increases mortality rates in NSCLC [39]. TonEBP induces hepatocellular carcinogenesis and is associated with liver cancer cell metastasis [42,43]. Although TonEBP is known to affect cancer migration in various carcinomas, the mechanisms by which it increases migration in lung cancer remain unclear. Therefore, in this study, we aimed to elucidate the mechanisms by which IL-1β and TonEBP affect cancer cell migration and invasion.
Phorbol 12-myristate 13-acetate (PMA), Hoechst 33342, and IPA3 were purchased from Sigma-Aldrich (St. Louis, MI); PD 98059, SP 600125, and IKK2 inhibitor IV were purchased from Calbiochem (San Diego, CA); antibodies against PAK1, phospho-PAK1, extracellular signal-regulated kinase (ERK), phospho-ERK, p38, phospho-p38, IκBα, pre-IL-1β, and glyceraldehyde 3-phosphate dehydrogenase (GAPDH) were purchased from Cell Signaling Technology (Danvers, MA); and secondary anti-rabbit antibodies were purchased from Invitrogen (Carlsbad, CA).
The human lung epithelial cell line A549 (CCL-185™) was purchased from ATCC (Manassas, VA, USA), and the human monocyte cell line THP-1 (40202) was purchased from Korean Cell Line Bank (Seoul, South Korea). A549 cells were cultured in RPMI 1640 medium (Welgene Inc., Daegu, South Korea) supplemented with 10% fetal bovine serum (GE Healthcare Life Sciences, Victoria, Australia), 50 U/mL penicillin, and 50 μg/mL streptomycin (Life Technologies, Carlsbad, CA), at 37°C in an incubator with 5% CO2. THP-1 cells were cultured in RPMI 1640 medium supplemented with 10% fetal bovine serum, as described above.
A549 cocultured-MΦ conditioned media
THP-1 cells were differentiated into macrophages by treatment with 30 nM PMA in independent wells in the upper Falcon® Permeable Support for 6-well plate with a 1.0-µm Transparent PET Membrane (Corning, NY, USA), and A549 cells were cultured separately. After 24 h, the macrophage-differentiated upper insert was transferred to the wells with A549 cells, followed by 24 h of incubation. The upper insert containing the A549 cocultured-MΦ was cultured in fresh culture medium for 24 h, and the culture supernatant was collected following centrifugation of the A549 cocultured-MΦ-conditioned medium. IL-1β levels (in pg/mL) in the culture media were determined using the Human IL-1β Quantikine enzyme-linked immunosorbent assay (ELISA) Kit (R&D Systems, Minneapolis, MN).
Small interfering RNA (siRNA) for TonEBP or negative control was purchased from Integrated DNA Technologies (pre-designed DsiRNA, Coralville, IA). A549 cells were transfected using Lipofectamine 2000 reagent (Invitrogen, Carlsbad, CA) according to the manufacturer’s instructions.
Cells were lysed using radioimmunoprecipitation buffer containing protease and phosphatase inhibitors. Total cell lysates were centrifuged at 12,000 × g for 15 min at 4°C, and the supernatants were collected. The protein concentration was determined using the SMART™ BCA Protein Assay Kit (iNtRON Biotechnology, Seongnam, South Korea). Proteins were separated via sodium dodecyl sulfate–polyacrylamide gel electrophoresis and then transferred to a nitrocellulose membrane. Nonspecific binding was performed via blocking with 5% non-fat milk and 5% bovine serum albumin in tris-buffered saline with Tween 20 (TBST; LPS solution, Daejeon, South Korea) for 30 min at room temperature. The membrane was incubated with primary antibodies in blocking solution overnight at 4°C, washed four times with TBST for 5 min each time, and then incubated with secondary antibodies. After washing with TBST, antibody-reactive proteins were detected using a chemiluminescence assay kit (Amersham Pharmacia Biotech, Freiburg, Germany).
For migration or invasion experiments, 4 × 104 cells/well were placed in a 24-well plate and treated with conditioned medium (CM) or IL-1β for 24 h. In the case of invasion, the cells were separated and coated with Matrigel (Corning, NY, USA), diluted in the medium at the same time. After 24 h, the cells were scraped with a micropipette tip, washed with Dulbecco’s phosphate-buffered saline, and the medium was replaced. Cell images were taken after 24 h using a Nikon Eclipse Ti microscope (100× magnification, Nikon, Tokyo, Japan).
For migration or invasion experiments, cells were split into 6-well plates and treated with CM or IL-1β for 24 h. Thereafter, the cells diluted with serum-free medium were split 1 × 104/well in an 8-μm-pore inserts chamber (Corning, NY, USA) of 24 wells. In the case of invasion, 50 µL of Matrigel diluted with serum-free medium was especially coated before splitting the cells. The bottom wells of the inserts were filled with 10% a lung medium and incubated for 24 h. After incubation, the insert was fixed with 4% paraformaldehyde and then DAPI-stained with Hoechst 33342. Images were captured using a Nikon Eclipse Ti microscope (40× magnification, Nikon, Tokyo, Japan) and analyzed using the ImageJ software.
Total RNA was isolated using TRIzol reagent (Life Technologies). Complementary DNA was synthesized from total RNA using the CellScript™ cDNA Master Mix (CellSafe, Yongin, South Korea). Relative RNA expression was detected via real-time PCR, using the Prism 7000 Sequence Detection System (Applied Biosystems, Foster City, CA) with TOPreal™ qPCR 2X PreMIX (Enzynomics, Daejeon, South Korea). The reaction conditions were as follows: 10 min at 95°C, followed by 40 cycles of 15 s at 95°C, 20 s at 60°C, and 30 s at 72°C. Primers (from Bioneer, Daejeon, South Korea) were as follows: for human GAPDH, forward, 5′-ACATCGCTCAGACACCATG-3′, and reverse, 5′-TG TAG TTG AGG TCA ATG AAG-3′; for human IL-1β, forward, 5′-ATGATGGCTTATTACAGTGGCAA-3′ and reverse, 5′-GTCGGAGATTCGTAGCTGGA-3′; for human PAK1 forward, 5′-CAACTCGGGACGTGGCTAC-3′, and reverse, 5′-CAGTATTCCGGGTCAAAGCAT-3′. mRNA levels, relative to those of GAPDH, were calculated according to the comparative 2−ΔΔCq method.
A549 cells were incubated with macrophage CM in 2 mL of medium for 12 h. The medium was changed after 12 h to obtain IL-1β secreted by pure A549 cells. IL-1β levels (in pg/mL) in the cell culture media were determined using the Human IL-1β Quantikine ELISA Kit (R&D Systems, Minneapolis, MN).
Data are presented as the mean ± SEM. Statistical significance was determined using Student’s t-test and one-way analysis of variance multiple comparison test using GraphPad Prism version 8.0 (GraphPad Software, Inc., USA).
A549 cocultured-MΦ-secreted IL-1β enhanced A549 cell migration and invasion
IL-1β is secreted by macrophages and is known to be associated with migration and invasion of cancer cells. We confirmed that IL-1β was actually secreted in vitro (data not shown). We investigated whether IL-1β was secreted from A549 cocultured-MΦ and whether it induced migration and invasion in A549 cells. ELISA confirmed IL-1β secretion by A549 cocultured-MΦ (Fig. 1A). pre-IL-1β expression was higher in A549 cocultured-MΦ than in THP-1 monocytes (Figs. 1B and 1C). A549 cells treated with IL-1β showed concentration-dependent increases in cell migration and invasion (Figs. 2A–2D). To determine whether IL-1β affects the A549 cocultured-MΦ–induced increase in migration, we performed migration and invasion experiments by treating cells with an IL-1 receptor antagonist (Figs. 2E–2H). Under cotreatment with A549 cocultured-MΦ and the IL-1 receptor antagonist, migration and invasion were lower than when A549 cocultured-MΦ were applied alone. This suggests that IL-1β plays a key role in the A549 cocultured-MΦ–induced increase in migration and invasion.
Figure 1: IL-1β secretion and expression in A549 cocultured-MΦ (A) IL-1β levels were determined via ELISA. (B) IL-1β and GAPDH expression were determined from whole-cell lysates. (C) IL-1β mRNA expression was analyzed via quantitative RT-PCR. Data are presented as the mean ± SEM of three independent experiments (***p < 0.001, compared with monocytes).
Figure 2: IL-1β secretion by A549 cocultured-MΦ promotes cancer cell migration and invasion. (A–D) A549 cells were treated with IL-1β. (E–H) A549 cells were incubated with A549 cocultured-MΦ-conditioned media in the presence or absence of IL-1RA for 24 h. Wound healing and transwell migration assays were conducted to determine the effects of IL-1β on cancer cell migration and invasion. Data are presented as the mean ± SEM of three independent experiments (*p < 0.05, **p < 0.01, ***p < 0.001, compared with 0 ng/mL and control; NI: non-induced).
ERK, p38, and NF-κB inhibitors reduced IL-1β–induced migration and invasion
The MAPK and NF-κB signaling pathways are known to induce IL-1β expression, which in turn promotes cancer cell migration and invasion. We verified this by treating A549 cells with IL-1β (Fig. 3A); this treatment induced ERK and p38 phosphorylation and reduced IκBα expression. To verify that these pathways affect cancer cell migration and invasion, we treated the cells with MAPK and IKK2 inhibitors (Figs. 3B and 3C); treatment with ERK, p38, and IKK2 inhibitors significantly reduced cell migration. Cell invasion was reduced significantly following treatment with ERK and IKK2 inhibitors but non-significantly following treatment with p38 inhibitor.
Figure 3: Effects of MAPK and NF-κB inhibitors on IL-1β–induced cancer cell migration and invasion. (A) p-p38/t-p38, p-ERK/t-ERK, and IκBα expression levels were determined using whole-cell lysates. (B, C) A549 cells were pretreated with ERK, p38 and IKK2 inhibitors for 1 h and then treated with IL-1β for 24 h. The effects of MAPK and NF-κB inhibitors on cancer cell migration and invasion were determined using a transwell migration assay. Data are presented as the mean ± SEM of three independent experiments (***p < 0.001, compared with the -).
PAK1 inhibitors reduced IL-1β–induced cancer cell migration and invasion
We investigated the phosphorylation of PAK1, a signaling molecule that induces cancer cell migration. We examined whether PAK1 signaling participates in the induction of cancer cell migration and invasion by IL-1β. We found that IL-1β treatment increased p-PAK1 expression relative to the control (Figs. 4A and 4B). Treatment with IPA3, a PAK1-phosphorylation blocker, significantly reduced cancer cell migration and invasion (Figs. 4C–4E). Many papers have reported that PAK1 induces migration in cancer cells by regulating MAPK and NF-κB. However, other previous reports indicated that ERK2 regulates PAK1 [44]. To determine whether PAK1 signaling is related to MAPK and NF-κB, we investigated p-PAK1 expression following treatment with MAPK and IKK2 inhibitors; treatment with ERK, p38, and IKK2 inhibitors reduced p-PAK1 expression relative to the control (Fig. 4F). This suggests that IL-1β induces cancer cell migration and invasion via the PAK1 pathway, downstream of ERK, p38, and NF-κB signaling.
Figure 4: Inhibitory effects of IPA3 on IL-1β–induced migration and invasion of A549 cells. p-PAK1/t-PAK1 expression levels were determined using whole-cell lysates. (A, B) Western blot results. (C–E) A549 cells were incubated with IL-1β in the presence or absence of IPA3 for 24 h. The effects of IPA3 on cancer cell migration and invasion were determined via wound healing and transwell migration assays. (F) A549 cells were pretreated with ERK, p38 and IKK2 inhibitors for 1 h and then treated with IL-1β for 24 h. Data are presented as the mean ± SEM of three independent experiments (*p < 0.05, compared with the con and -; con: control).
TonEBP deficiency suppressed A549 cell migration and invasion
TonEBP plays important roles in stress responses, osmotic regulation, and cancer, affecting cancer cell migration. We examined the association between TonEBP and IL-1β, both closely associated with cancer cell migration and invasion. TonEBP depletion via siRNA reduced cancer cell migration and invasion relative to the control (Figs. 5A–5D).
Figure 5: Effects of TonEBP depletion on cancer cell migration and invasion. A549 cells were transfected with siTonEBP or siNC for 24 h and then treated with IL-1β for 24 h. (A–D) Migration and Invasion were quantified via wound healing and transwell migration assays. Data are presented as the mean ± SEM of three independent experiments (*p < 0.05, **p < 0.01, ***p < 0.001, compared with the siNC treatment; NC: negative control).
We then investigated how TonEBP regulates cell migration and invasion. To determine whether TonEBP affects IL-1β-activated ERK, p38, and NF-κB, we investigated ERK and p38 phosphorylation and IκBα expression following TonEBP depletion; we found that TonEBP depletion did not affect total or phosphorylated ERK, p38, and IκBα levels (Fig. 6A), but it reduced the protein and mRNA expression of PAK1, a signaling molecule downstream of MAPK and NF-κB, relative to the control (Figs. 6B and 6C). These results suggest that although TonEBP regulates PAK1 expression, it is independent of ERK, p38, and NF-κB signaling. Here, treatment with IL-1β did not directly increase TonEBP expression; however, depletion of TonEBP increased responsiveness to IL-1β–induced migration and invasion. In addition, TonEBP deficiency also affected the expression level of PAK1, indicating that the expression level of TonEBP is involved in IL-1β–induced migration and invasion as well as in the PAK1 signaling pathway.
Figure 6: Expression of p-PAK1 and T-PAK1 on TonEBP deficiency. (A) TonEBP, p-p38/t-p38, p-ERK/t-ERK, and IκBα expression levels were determined using whole-cell lysates. A549 cells were transfected with siTonEBP or siNC for 48 h and then treated with IL-1β for 1 h. (B) PAK1 mRNA expression was analyzed via quantitative RT-PCR. (C) p-PAK1 and T-PAK1 expression was determined using whole-cell lysates. Data are presented as the mean ± SEM of three independent experiments (**p < 0.01, ***p < 0.001, compared with the siNC treatment; NC: negative control).
Lung cancer is the cancer with the highest mortality rate worldwide. The high mortality rate of lung cancer can be attributed to difficulties in obtaining an early diagnosis and a high metastasis rate [1–3]. In general, the key to cancer metastasis is migration and invasion, and the TME plays an important role in these processes. For example, TAMs with high CD5L expression have been shown to be associated with poor prognosis in patients with papillary lung adenocarcinoma [45], and subtypes of high-grade serous ovarian cancer macrophages have been revealed to be associated with the prognosis of tumor extracellular matrix signatures [46]. Moreover, analysis of the TME in metastatic breast cancer identified several tumor-promoting genes in bone marrow cells and confirmed diversity in CAFs, endothelial cells, and mural cells [47]. It has been reported that TAMs create a type of cancer microenvironment that induces cancer metastasis and increases the migration and invasion of cancer cells [10].
IL-1β and PAK1 are known to increase migration and invasion in various cancer cells, including lung cancer, which is consistent with our results. TonEBP has been reported to be related to migration and invasion in liver cancer cells; however, it is not known in other cancer cells, including lung cancer, and no association with IL-1β or PAK1 has been reported. Our results show that TonEBP plays an important role in IL-1β–induced cancer cell migration and invasion and that some of these effects proceed via the regulation of PAK1 expression in A549 cancer cells (Fig. 7).
Figure 7: Schematic diagram of the effects of IL-1β and TonEBP expression on cell migration and invasion in A549 cells.
The MAPK and NF-κB signaling pathways activate IL-1β–induced cancer cell migration and invasion, and inhibition of IL-1β–induced migration and invasion by an inhibitor or RNAi reduces cancer cell migration and invasion [48]. It has been reported that SCLC (Small cell lung cancer) and SLC (Squamous cell carcinoma) patient tissues have higher IL-1β expression than normal tissues [49]. We confirmed that migration and invasion induced by inhibitors of MAPK and NF-κB were reduced in the investigated lung cancer cell lines. This indicates that IL-1β induces cancer cell migration and invasion via the ERK and NF-κB signaling pathways.
PAK1 is known to be phosphorylated because of the binding of cdc42/rac1, a small GTPase protein, and the signal transduction of cdc42/rac1/PAK1 has been studied in tumors as well [50]. In esophageal squamous cell carcinoma, pharmacological inhibition of PAK1 reduces cancer cell migration and invasion as well as matrix metalloproteinase (MMP)-2 and MMP-9 expression [51]. In hepatocellular carcinoma, PAK1 is overexpressed together with Snail; further, PAK1 deficiency reduces cancer cell proliferation, migration, and invasion and induces apoptosis [37]. Here, we found that PAK1 plays a key role in the IL-1β–induced migration and invasion of lung cancer cells. PAK1 phosphorylation induced by IL-1β was reduced by inhibition of ERK and NF-κB. This suggests that IL-1β–induced ERK and NF-κB signaling induces cancer cell migration and invasion by regulating PAK1 phosphorylation. Previous studies have shown that A549 cells as well as various types of lung cancer cells induce migration and invasion [52,53]. This study has certain limitations. For instance, A549 cells harbor a k-RAS mutation and cannot be used to represent lung cancer cells with EGF mutations. Even in the case of THP-1 cells, artificially created conditions via in vitro co-culture with A549 cells cannot represent TAMs. To this end, it is necessary to isolate TAMs from lung cancer and conduct further research.
As a transcriptional regulator, TonEBP regulates various genes involved in specific tissues or diseases. TonEBP directly inhibits HO-1, a stress-inducing protein, in macrophages [54] and regulates inflammation-related TLR-induced genes by interacting with NF-κB [55]. TonEBP is essential in regulating osmotic pressure under cyclooxygenase-2–induced hyperosmotic conditions in cancer [56]. In addition, studies revealed that TonEBP promotes neuroinflammation and cognitive impairment via the upregulation of LCN2 in mice in a diabetes model [57]. It has been shown that TonEBP-p65 activates NF-κB by forming a complex with p300, a transcriptional coactivator. This suggests that TonEBP not only regulates genes directly but also indirectly [39]. Here, we found that TonEBP regulated PAK1. IL-1β-activated MAPK and NF-κB signaling were not affected by TonEBP deficiency, although it reduced the expression of PAK1, a downstream signaling molecule. Modulating the expression of TonEBP in A549 cells did not directly mediate IL-1β but altered the responsiveness of IL-1β to affect cancer cell migration and invasion. This suggests that TonEBP directly regulates PAK1 via the IL-1β–induced migration and invasion signaling pathways. To investigate the regulation of PAK1 expression by TonEBP, after depleting TonEBP, the expression of Rac1 and cdc42, which function upstream of PAK1, was examined; however, there was no significant difference (data not shown). TonEBP expression is associated with survival rate in lung cancer patients [39]. However, inhibitors of TonEBP have not yet been developed. Through this study, it was revealed that expression of TonEBP increases IL-1β reactivity. Therefore, future studies will investigate the inhibition effect of TonEBP expression on tumor size and metastasis using RNAi-nanoparticles in animal experiments. Animal experiments are expected to show the possibility of developing effective anticancer drugs using the regulation of TonEBP expression. Also, PAK1 reportedly affects the survival of PAK1-positive patients with NSCLC [58]. In an in vivo experiment, an inhibitor of PAK1 reduced the tumor size in a murine lung cancer model [59]. However, to the best of our knowledge, no clinical study has directly related lung cancer metastasis with TonEBP and PAK1 in patients with lung cancer, and this is an issue that warrants further investigation. Furthermore, the association between TonEBP and PAK1 is worth highlighting.
Acknowledgement: We would like to thank Editage (www.editage.co.kr) for editing and reviewing this manuscript for English language.
Funding Statement: This research was supported by Basic Science Research Program through the National Research Foundation of Korea (NRF) funded by the Ministry of Education (NRF-2014R1A6A1029617).
Author Contributions: SHJ, KTH, KSJ, and CHN performed the experiments. SHJ and KTH analyzed the data. SHJ wrote the manuscript. LSD designed this study and manuscript. All authors read and approved the final manuscript.
Availability of Data and Materials: The datasets used and/or analyzed during the current study are available from the corresponding author upon reasonable request.
Ethics Approval: Not applicable.
Conflicts of Interest: The authors declare that they have no conflicts of interest to report regarding the present study.
References
1. Wang, Q. Z., Xu, W., Habib, N., Xu, R. (2009). Potential uses of microRNA in lung cancer diagnosis, prognosis, and therapy. Current Cancer Drug Targets, 9(4), 572–594. [Google Scholar] [PubMed]
2. Wang, G., Reed, E., Li, Q. (2004). Molecular basis of cellular response to cisplatin chemotherapy in non-small cell lung cancer (Review). Oncology Reports, 12(5), 955–965. [Google Scholar] [PubMed]
3. Sosa Iglesias, V., Giuranno, L., Dubois, L. J., Theys, J., Vooijs, M. (2018). Drug resistance in non-small cell lung cancer: A potential for NOTCH targeting? Frontiers in Oncology, 8, 267. [Google Scholar] [PubMed]
4. Allinen, M., Beroukhim, R., Cai, L., Brennan, C., Lahti-Domenici, J. et al. (2004). Molecular characterization of the tumor microenvironment in breast cancer. Cancer Cell, 6(1), 17–32. [Google Scholar] [PubMed]
5. Emon, B., Bauer, J., Jain, Y., Jung, B., Saif, T. (2018). Biophysics of tumor microenvironment and cancer metastasis—A mini review. Computational and Structural Biotechnology Journal, 16, 279–287. [Google Scholar] [PubMed]
6. Laplane, L., Duluc, D., Larmonier, N., Pradeu, T., Bikfalvi, A. et al. (2018). The multiple layers of the tumor environment. Trends in Cancer, 4(12), 802–809. [Google Scholar] [PubMed]
7. Baghban, R., Roshangar, L., Jahanban-Esfahlan, R., Seidi, K., Ebrahimi-Kalan, A. et al. (2020). Tumor microenvironment complexity and therapeutic implications at a glance. Cell Communication and Signaling, 18, 1–19. [Google Scholar]
8. Seidi, K., Jahanban-Esfahlan, R., Zarghami, N. J. T. et al. (2017). Tumor rim cells: From resistance to vascular targeting agents to complete tumor ablation. Tumor Biology, 39(3), 1010428317691001. [Google Scholar] [PubMed]
9. Lee, S. W., Kwak, H. S., Kang, M. H., Park, Y. Y., Jeong, G. S. (2018). Fibroblast-associated tumour microenvironment induces vascular structure-networked tumouroid. Scientific Reports, 8(1), 2365. [Google Scholar] [PubMed]
10. Quatromoni, J. G., Eruslanov, E. (2012). Tumor-associated macrophages: Function, phenotype, and link to prognosis in human lung cancer. American Journal of Translational Research, 4(4), 376–389. [Google Scholar] [PubMed]
11. Schmall, A., Al-Tamari, H. M., Herold, S., Kampschulte, M., Weigert, A. et al. (2015). Macrophage and cancer cell cross-talk via CCR2 and CX3CR1 is a fundamental mechanism driving lung cancer. American Journal of Respiratory and Critical Care Medicine, 191(4), 437–447. [Google Scholar] [PubMed]
12. Wang, M., Zhao, J., Zhang, L., Wei, F., Lian, Y. et al. (2017). Role of tumor microenvironment in tumorigenesis. Journal of Cancer, 8(5), 761–773. [Google Scholar] [PubMed]
13. Condeelis, J., Pollard, J. W. (2006). Macrophages: Obligate partners for tumor cell migration, invasion, and metastasis. Cell, 124(2), 263–266. [Google Scholar] [PubMed]
14. Jayasingam, S. D., Citartan, M., Thang, T. H., Mat Zin, A. A., Ang, K. C. et al. (2020). Evaluating the polarization of tumor-associated macrophages into M1 and M2 phenotypes in human cancer tissue: Technicalities and challenges in routine clinical practice. Frontiers in Oncology, 9, 1512. [Google Scholar] [PubMed]
15. Sedighzadeh, S. S., Khoshbin, A. P., Razi, S., Keshavarz-Fathi, M., Rezaei, N. J. T. L. C. R. (2021). A narrative review of tumor-associated macrophages in lung cancer: Regulation of macrophage polarization and therapeutic implications. Translational Lung Cancer Research, 10(4), 1889–1916. [Google Scholar] [PubMed]
16. Vahl, J. M., Friedrich, J., Mittler, S., Trump, S., Heim, L. et al. (2017). Interleukin-10-regulated tumour tolerance in non-small cell lung cancer. British Journal of Cancer, 117(11), 1644–1655. [Google Scholar] [PubMed]
17. Shang, G. S., Liu, L., Qin, Y. W. J. O. l. (2017). IL-6 and TNF-α promote metastasis of lung cancer by inducing epithelial-mesenchymal transition. Oncology Letters, 13(6), 4657–4660. [Google Scholar] [PubMed]
18. Lin, C. C., Kuo, C. T., Cheng, C. Y., Wu, C. Y., Lee, C. W. et al. (2009). IL-1β promotes A549 cell migration via MAPKs/AP-1- and NF-κB-dependent matrix metalloproteinase-9 expression. Cell Signal, 21(11), 1652–1662. [Google Scholar] [PubMed]
19. Tseng, H. C., Lee, I. T., Lin, C. C., Chi, P. L., Cheng, S. E. et al. (2013). IL-1β promotes corneal epithelial cell migration by increasing MMP-9 expression through NF-κB- and AP-1-dependent pathways. PLoS One, 8(3), e57955. [Google Scholar] [PubMed]
20. Cheng, C. Y., Hsieh, H. L., Sun, C. C., Lin, C. C., Luo, S. F. et al. (2009). IL-1β induces urokinase-plasminogen activator expression and cell migration through PKC α, JNK1/2, and NF-κB in A549 cells. Journal of Cellular Physiology, 219(1), 183–193. [Google Scholar] [PubMed]
21. Mantovani, A., Barajon, I., Garlanda, C. (2018). IL-1 and IL-1 regulatory pathways in cancer progression and therapy. Immunological Reviews, 281(1), 57–61. [Google Scholar] [PubMed]
22. Bent, R., Moll, L., Grabbe, S., Bros, M. J. I. J. O. M. S. (2018). Interleukin-1 beta–A friend or foe in malignancies? International Journal of Molecular Sciences, 19(8), 2155. [Google Scholar] [PubMed]
23. Petrella, B. L., Armstrong, D. A., Vincenti, M. P. J. C. L. (2012). Interleukin-1 beta and transforming growth factor-beta 3 cooperate to activate matrix metalloproteinase expression and invasiveness in A549 lung adenocarcinoma cells. Cancer Letters, 325(2), 220–226. [Google Scholar] [PubMed]
24. Masola, V., Carraro, A., Granata, S., Signorini, L., Bellin, G. et al. (2019). In vitro effects of interleukin (IL)-1 beta inhibition on the epithelial-to-mesenchymal transition (EMT) of renal tubular and hepatic stellate cells. Journal of Translational Medicine, 17(1), 12. [Google Scholar] [PubMed]
25. Jiang, J., Tang, Y. L., Liang, X. H. (2011). EMT: A new vision of hypoxia promoting cancer progression. Cancer Biology & Therapy, 11(8), 714–723. [Google Scholar]
26. Huang, J., Lan, X., Wang, T., Lu, H., Cao, M. et al. (2020). Targeting the IL-1β/EHD1/TUBB3 axis overcomes resistance to EGFR-TKI in NSCLC. Oncogene, 39(8), 1739–1755. [Google Scholar] [PubMed]
27. Rebe, C., Ghiringhelli, F. (2020). Interleukin-1β and cancer. Cancers, 12(7), 1791. [Google Scholar] [PubMed]
28. McLoed, A. G., Sherrill, T. P., Cheng, D. S., Han, W., Saxon, J. A. et al. (2016). Neutrophil-derived IL-1β impairs the efficacy of NF-κB inhibitors against lung cancer. Cell Reports, 16(1), 120–132. [Google Scholar] [PubMed]
29. Barrera, L., Montes-Servín, E., Hernandez-Martinez, J. M., Orozco-Morales, M., Montes-Servín, E. et al. (2018). Levels of peripheral blood polymorphonuclear myeloid-derived suppressor cells and selected cytokines are potentially prognostic of disease progression for patients with non-small cell lung cancer. Cancer Immunology, Immunotherapy, 67, 1393–1406. [Google Scholar] [PubMed]
30. Shahriari, K., Shen, F., Worrede-Mahdi, A., Liu, Q., Gong, Y. et al. (2017). Cooperation among heterogeneous prostate cancer cells in the bone metastatic niche. Oncogene, 36(20), 2846–2856. [Google Scholar] [PubMed]
31. Mierke, C. T., Puder, S., Aermes, C., Fischer, T., Kunschmann, T. (2020). Effect of PAK inhibition on cell mechanics depends on Rac1. Frontiers in Cell and Developmental Biology, 8, 13. [Google Scholar] [PubMed]
32. Huang, Q., Lan, F., Wang, X., Yu, Y., Ouyang, X. et al. (2014). IL-1β-induced activation of p38 promotes metastasis in gastric adenocarcinoma via upregulation of AP-1/c-fos, MMP2 and MMP9. Molecular Cancer, 13(1), 1–15. [Google Scholar]
33. Wang, B., Shi, L., Sun, X., Wang, L., Wang, X. et al. (2016). Production of CCL20 from lung cancer cells induces the cell migration and proliferation through PI3K pathway. Journal of Cellular and Molecular Medicine, 20(5), 920–929. [Google Scholar] [PubMed]
34. Jung, J. S., Jung, K., Kim, D. H., Kim, H. S. (2012). Selective inhibition of MMP-9 gene expression by mangiferin in PMA-stimulated human astroglioma cells: Involvement of PI3K/Akt and MAPK signaling pathways. Pharmacological Research, 66(1), 95–103. [Google Scholar] [PubMed]
35. Kichina, J. V., Goc, A., Al-Husein, B., Somanath, P. R., Kandel, E. S. J. E. O. O. T. T. (2010). PAK1 as a therapeutic target. Expert Opinion on Therapeutic Targets, 14(7), 703–725. [Google Scholar] [PubMed]
36. Zhou, W., Jubb, A. M., Lyle, K., Xiao, Q., Ong, C. C. et al. (2014). PAK1 mediates pancreatic cancer cell migration and resistance to MET inhibition. The Journal of Pathology, 234(4), 502–513. [Google Scholar] [PubMed]
37. Cao, F., Yin, L. X. (2020). PAK1 promotes proliferation, migration and invasion of hepatocellular carcinoma by facilitating EMT via directly up-regulating Snail. Genomics, 112(1), 694–702. [Google Scholar] [PubMed]
38. Halterman, J. A., Kwon, H. M., Wamhoff, B. R. (2012). Tonicity-independent regulation of the osmosensitive transcription factor TonEBP (NFAT5). American Journal of Physiology-Cell Physiology, 302(1), C1–C8. [Google Scholar] [PubMed]
39. Lee, H. H., Sanada, S., An, S. M., Ye, B. J., Lee, J. H. et al. (2016). LPS-induced NFκB enhanceosome requires TonEBP/NFAT5 without DNA binding. Scientific Reports, 6(1), 1–12. [Google Scholar]
40. Woo, S. K., Lee, S. D., Na, K. Y., Park, W. K., Kwon, H. M. et al. (2002). TonEBP/NFAT5 stimulates transcription of HSP70 in response to hypertonicity. Molecular and Cellular Biology, 22(16), 5753–5760. [Google Scholar] [PubMed]
41. Choi, S. Y., Lee-Kwon, W., Kwon, H. M. (2020). The evolving role of TonEBP as an immunometabolic stress protein. Nature Reviews Nephrology, 16(6), 352–364. [Google Scholar] [PubMed]
42. Lee, J. H., Suh, J.h, Choi, S. Y., Kang, H. J., Lee, G. R. et al. (2017). TonEBP promotes hepatocellular carcinoma and is associated with poor prognosis. Cancer Research, 77, 4726. [Google Scholar]
43. Lee, J. H., Suh, J. H., Choi, S. Y., Kang, H. J., Lee, H. H. et al. (2019). Tonicity-responsive enhancer-binding protein promotes hepatocellular carcinogenesis, recurrence and metastasis. Hepatology, 68, 347–358. [Google Scholar]
44. Sundberg-Smith, L. J., Doherty, J. T., Mack, C. P., Taylor, J. M. (2005). Adhesion stimulates direct PAK1/ERK2 association and leads to ERK-dependent PAK1 Thr212 phosphorylation. Journal of Biological Chemistry, 280(3), 2055–2064. [Google Scholar] [PubMed]
45. Sanchez-Moral, L., Paul, T., Martori, C., Font-Diaz, J., Sanjurjo, L. et al. (2023). Macrophage CD5L is a target for cancer immunotherapy. eBioMedicine, 91, 104555. [Google Scholar] [PubMed]
46. Puttock, E. H., Tyler, E. J., Manni, M., Maniati, E., Butterworth, C. et al. (2023). Extracellular matrix educates an immunoregulatory tumor macrophage phenotype found in ovarian cancer metastasis. Nature Communications, 14(1), 2514. [Google Scholar] [PubMed]
47. Zou, Y., Ye, F., Kong, Y., Hu, X., Deng, X. et al. (2023). The single-cell landscape of intratumoral heterogeneity and the immunosuppressive microenvironment in liver and brain metastases of breast cancer. Advanced Science, 10(5), e2203699. [Google Scholar] [PubMed]
48. Zhang, W., Borcherding, N., Kolb, R. J. T. M. (2020). IL-1 signaling in tumor microenvironment. Advances in Experimental Medicine and Biology, 1240, 1–23. [Google Scholar] [PubMed]
49. Kong, H., Wang, Y., Zeng, X., Wang, Z., Wang, H. et al. (2015). Differential expression of inflammasomes in lung cancer cell lines and tissues. Tumor Biology, 36(10), 7501–7513. [Google Scholar] [PubMed]
50. Maldonado, M. D. M., Dharmawardhane, S. (2018). Targeting Rac and Cdc42 GTPases in cancer. Cancer Research, 78(12), 3101–3111. [Google Scholar] [PubMed]
51. Chen, L., Bi, S., Hou, J., Zhao, Z., Wang, C. et al. (2019). Targeting p21-activated kinase 1 inhibits growth and metastasis via Raf1/MEK1/ERK signaling in esophageal squamous cell carcinoma cells. Cell Communication and Signaling, 17(1), 31. [Google Scholar] [PubMed]
52. Singh, T., Katiyar, S. K. (2013). Honokiol inhibits non-small cell lung cancer cell migration by targeting PGE2-mediated activation of β-catenin signaling. PLoS One, 8(4), e60749. [Google Scholar] [PubMed]
53. Chen, P., Wu, Q., Feng, J., Yan, L., Sun, Y. et al. (2020). Erianin, a novel dibenzyl compound in Dendrobium extract, inhibits lung cancer cell growth and migration via calcium/calmodulin-dependent ferroptosis. Signal Transduction and Targeted Therapy, 5(1), 51. [Google Scholar] [PubMed]
54. Yoo, E. J., Lee, H. H., Ye, B. J., Lee, J. H., Lee, C. Y. et al. (2019). TonEBP suppresses the HO-1 gene by blocking recruitment of Nrf2 to its promoter. Frontiers in Immunology, 10, 850. [Google Scholar] [PubMed]
55. Hwang, H. S., Lee, M. H., Kim, H. A. J. S. R. (2021). The TLR-2/TonEBP signaling pathway regulates 29-kDa fibronectin fragment-dependent expression of matrix metalloproteinases. Scientific Reports, 11(1), 1–15. [Google Scholar]
56. Choi, H., Chaiyamongkol, W., Doolittle, A. C., Johnson, Z. I., Gogate, S. S. et al. (2018). COX-2 expression mediated by calcium-TonEBP signaling axis under hyperosmotic conditions serves osmoprotective function in nucleus pulposus cells. Journal of Biological Chemistry, 293(23), 8969–8981. [Google Scholar] [PubMed]
57. Jeong, E., Lee, J., Shin, H. J., Lee, J. Y., Kim, K. E. et al. (2021). Tonicity-responsive enhancer-binding protein promotes diabetic neuroinflammation and cognitive impairment via upregulation of lipocalin-2. Journal of Neuroinflammation, 18(1), 1–18. [Google Scholar]
58. Chung, J. H., Kim, T., Kang, Y. J., Yoon, S. H., Kim, Y. S. et al. (2020). PAK1 as a potential therapeutic target in male smokers with EGFR-mutant non-small cell lung cancer. Molecules, 25(23), 5588. [Google Scholar] [PubMed]
59. Ito, M., Codony-Servat, C., Codony-Servat, J., Lligé, D., Chaib, I. et al. (2019). Targeting PKCι-PAK1 signaling pathways in EGFR and KRAS mutant adenocarcinoma and lung squamous cell carcinoma. Cell Communication and Signaling, 17, 1–11. [Google Scholar]
Cite This Article
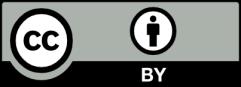
This work is licensed under a Creative Commons Attribution 4.0 International License , which permits unrestricted use, distribution, and reproduction in any medium, provided the original work is properly cited.