Open Access
REVIEW
Recent updates on nano-phyto-formulations based therapeutic intervention for cancer treatment
1 Department of Pharmaceutics, School of Pharmaceutical Sciences, Delhi Pharmaceutical Sciences and Research University (DPSRU), Pushp Vihar, New Delhi,
110017, India
2 Department of Pharmaceutical Sciences, Gurugram University, Haryana, 122003, India
3 Department of Life Sciences, Sharda School of Basic Sciences and Research, Sharda University, Greater Noida, Uttar Pradesh, 201310, India
4 Department of Biotechnology, Graphic Era (Deemed to be University), Dehradun, Uttarakhand, 248002, India
5 Department of Pharmacology, Shobhaben Pratapbhai Patel School of Pharmacy & Technology Management, SVKM’s NMIMS, Vile Parle (West), Mumbai,
400056, India
6 Department of Bio-Sciences and Technology, Maharishi Markandeshwar Engineering College, Maharishi Markandeshwar (Deemed to be University), Mullana,
Ambala, 133207, India
* Corresponding Authors: Madhu Gupta, ; Hardeep Singh Tuli,
Oncology Research 2024, 32(1), 19-47. https://doi.org/10.32604/or.2023.042228
Received 23 May 2023; Accepted 27 July 2023; Issue published 15 November 2023
Abstract
Cancer is a leading cause of death globally, with limited treatment options and several limitations. Chemotherapeutic agents often result in toxicity which long-term conventional treatment. Phytochemicals are natural constituents that are more effective in treating various diseases with less toxicity than the chemotherapeutic agents providing alternative therapeutic approaches to minimize the resistance. These phytoconstituents act in several ways and deliver optimum effectiveness against cancer. Nevertheless, the effectiveness of phyto-formulations in the management of cancers may be constrained due to challenges related to inadequate solubility, bioavailability, and stability. Nanotechnology presents a promising avenue for transforming current cancer treatment methods through the incorporation of phytochemicals into nanosystems, which possess a range of advantageous characteristics such as biocompatibility, targeted and sustained release capabilities, and enhanced protective effects. This holds significant potential for future advancements in cancer management. Herein, this review aims to provide intensive literature on diverse nanocarriers, highlighting their applications as cargos for phytocompounds in cancer. Moreover, it offers an overview of the current advancements in the respective field, emphasizing the characteristics that contribute to favourable outcomes in both in vitro and in vivo settings. Lastly, clinical development and regulatory concerns are also discussed to check on the transformation of the concept as a promising strategy for combination therapy of phytochemicals and chemotherapeutics that could lead to cancer management in the future.Graphic Abstract
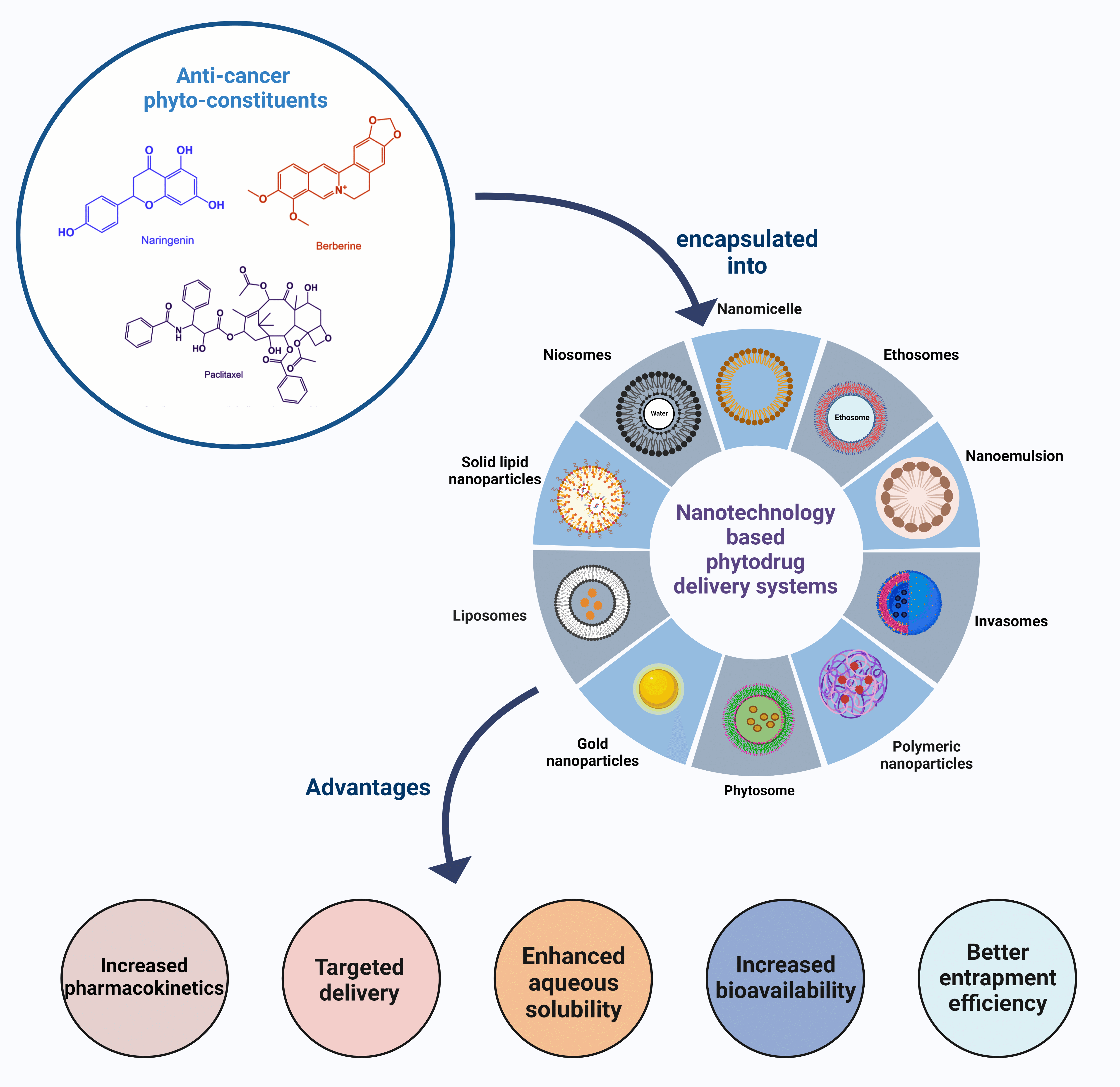
Keywords
Cancer was and still is one of the dreadful diseases from ancient times. Though ages have witnessed many advances in cancer therapy, the rising cases indicate the call for much deep understanding and newer therapeutic moieties or remolding of the existing therapy to control the prevalence of the disease. International Agency for Research on Cancer (IARC) has conducted a survey in 185 countries on all age groups, sex categories, and 36 types of cancers to make available a global cancer burden database based on cancer incidents and cancer mortalities [1]. This data is alarming enough (Fig. 1) to look for new treatments that can outshine conventional treatments. Conventional therapy includes surgery, chemotherapeutic anticancer drug delivery, or radiation therapy. Cancer cells exhibit a high rate of proliferation, leading to their expansion and concurrent infiltration of adjacent cells. Conventional therapy is associated with affecting/destroying healthy cells during treatment, which can also lead to lethal effects [2].
Figure 1: Estimated number of new cases in 2020 in the world for both sexes [9].
Nanotechnology is quickly acquiring worldwide consideration as a crucial part of biomedical research explicitly aimed at cancer theranostics. The unique properties of nano-formulations include a tailorable surface and a high surface area-to-volume ratio. This system facilitates efficient adsorbing and encapsulating of the therapeutic agent, including phytochemicals, for site-specific drug delivery or passive delivery. Additionally, these nanoformulations condense the possibility of systemic toxicity and enhance the bioavailability with programmed release to the target site. Some common examples of such carrier systems are liposomes, polymeric nanoparticles (NPs), and polymeric micelles [3]. Nowadays, healthcare professionals are looking up to the traditional medicinal system to redesign the current therapeutics by combining the knowledge of the herbal with technology. Herbal medicine, or phytomedicine, uses bioactives derived from plants/herbs to promote wellbeing [4–6]. Severe synthetic medicines-related adverse effects and poor therapeutic choices for acute diseases make plant-based medications popular worldwide. Many clinical trials are underway to check out the chemotherapeutic potential of plant-based chemicals [7,8]. Natural plant resource phytochemicals can be used alone or in combination with anticancer drugs for better cancer theranostic over the conventional approach.
Numerous phytochemicals have shown prominent anticancer activity in preclinical models, including Curcumin, irinotecan, marijuana, and epipodophyllotoxin. Secondary metabolites like terpenes, alkaloids, terpenoids, flavonols, phenols, etc., are a vital source of anticancer compounds undergoing clinical trials [10]. The secondary metabolites help in the regulation of natural processes within the body. These mechanisms encompass the inhibition of overexpressed proteins, enzymes, amino acids, and hormones. Phytochemicals additionally expedite the synthesis of defensive enzymes. Also, they regulate different pathways, proving their role in relative oxygen generation [11]. Clinical results are still in the research phase, and it is not easy to find an optimal combination treatment [12].
Despite the encouraging potential of phytochemicals as an anticancer agent, several concerns still need to be pondered. The primary issues associated with phytochemicals are their low solubility, susceptibility to first-pass metabolism, narrow therapeutic window, and lack of specificity. Low solubility hampers the characteristic of permeability and ultimately affects bioavailability. Phyto-formulations given via the oral route may go through the first-pass effect and can get degraded before their therapeutic action. Apart from these pharmacological concerns, another concern associated with phytochemicals is being natural components they may be recognized as supplements and may be taken up by normal healthy tissue [13]. In addition, another hurdle in the effective use of phytochemicals is the chance of the development of resistance via multiple pathways. Indeed, nanotechnology can help to overcome the pitfalls of conventional phytochemicals-based therapy. Still, detailed research, including a clinical trial, is the call of time to make the phytochemical-based nano-therapy a success [14,15]. The present review focuses on nanotechnology-based phyto-formulations and their application in cancer treatment. Also, a brief of clinical trial status and future perspective of phytochemical-based nanotherapeutics is discussed.
Phytochemicals for Cancer Therapy
Progressive cell division and the ability of the cells to spread to other bodily areas are the primary characteristics of cancer. Various factors are mainly responsible for the cause of cancer, including epigenetic modifications in the genes, environmental factors like UV radiation, smoking, exposure to harmful chemicals, etc. Epidemiological studies revealed that incidences of cancers are increasing worldwide along with an increase in the problem of drug resistance. This emphasizes researchers’ interest in developing strategies related to cancer treatment [16,17]. Phytochemical-based foods or treatments have taken a massive lead over the past few decades. Researchers are keen to approach a unique treatment method for diseases like neurodegeneration, obesity, and cancer. Plant-based food, including vegetables, fruits, and whole grains, contribute to positive health outcomes because they are rich in diverse phytochemicals of great importance to human health [18]. Today, research in the field of cancer is mainly transferred from synthetic molecules to phytoconstituents (Table 1) because of their easy availability, economical, less side/toxic effects, and combination therapy. One of the main drawbacks associated with anticancer chemotherapy regimens is the emergence of resistance that occurs over the course of ongoing treatment. Phytoconstituents play the most significant role in combating chemo-resistance by modulating targets like various signaling events and many regulators involved in drug transport, cell survival, epithelial-mesenchymal transition, apoptosis, etc. The phenomenon of chemo-resistance is observed when there is an excessive expression of efflux pumps, namely MDR1, p-gp, LRP, and BCRP. Phenolic phytochemicals, including genistein, EGCG, quercetin, emodin, and resveratrol, have been found to enhance the cytotoxic potential of various cytotoxic drugs such as paclitaxel, docetaxel, gemcitabine, 5-FU, vinblastine, vincristine, cisplatin, doxorubicin, TRAIL, temozolomide, and sorafenib. This enhancement is achieved through the modulation of multiple signalling pathways and the targeting of molecules that confer resistance to these drugs [19]. However, recent research has been primarily dedicated to investigating the utilisation of formulations containing phytochemicals in combination with conventional anticancer medications. The aim is to restore the sensitivity to chemotherapy in cases where resistance has developed due to prolonged use of these chemotherapeutic agents. Therefore, these combination therapies improve healing efficacy and result in favorable treatment outcomes [20]. Thus, this section mainly discusses the role of essential phytochemicals in cancer treatment.
One of the largest groups of phytochemicals in the plant kingdom is polyphenols. They are bioactive compounds containing multiple phenolic groups in their structure, mainly classified as natural, synthetic, or semisynthetic compounds [21]. Polyphenols are a huge source of antioxidants, and this feature contributes to their mechanism of action as they scavenge free radicals or reactive oxygen species (ROS) and lower their levels in the human body [22,23]. Several studies have reported that various plant-derived natural polyphenols can protect normal cells directly or indirectly from the induction of neoplastic transformation due to xenobiotics and carcinogenic factors, thereby decreasing the risk of developing cancer [24]. Previous reports have established that in prostate cancer, polyphenolic compounds act as a chemopreventive agent due to their antioxidant or pro-oxidation activity, which is mainly contributed by reducing ROS generation, increasing enzyme activity specifically for antioxidation, and lastly by inducing cytotoxic effects.
Moreover, polyphenolic compounds also modulate androgen receptors by inhibiting their function or expression. In addition, polyphenolic compounds activate various signaling pathways in prostate cancer, like PI3K, Akt, ERK1/2, FOXO, GSK-3β, RTK, etc., ultimately suppressing cancer [25,26]. Polyphenols induce cell cycle arrest by downregulating various proteins, including cdc25, CDK2 and CDK4, cyclin E, and cyclin D1, and upregulating the proteins that code for tumor suppressor genes such as p21, p53, etc. They also induce apoptosis by activating caspase -3, -9, cyt-c, or Bax proteins [27].
Resveratrol, chemically known as 3, 5, 4′-trihydroxy-trans-stilbene, is a natural polyphenol in the stilbene family. Familiar plant sources of resveratrol include peanuts, almonds, berries-raspberries, mulberries, blueberries, grapes, beans, etc. [28]. Researchers have reported that resveratrol inhibits skin cancer development and can delay its onset. It protects DNA from ROS [29]. For example, resveratrol protects DNA from free radical generation and scavenges ROS, superoxides, and hydroxyls produced in cancerous cells—a cascade of events related to tumor initiation [30]. According to Pereria and colleagues, resveratrol lessens pancreatic acinar cell necrosis and early intracellular trypsin activation. In addition to this, resveratrol also inhibits NF-kB signaling leading to anti-inflammatory effects after oral administration [31]. Zhang and colleagues stated that the co-encapsulated delivery of resveratrol and docetaxel nanoliposome combination exhibited higher cytotoxicity and increased the activity caspase-3, leading to cell death in prostate cancer. Moreover, this nanoliposome combination also decreases the growth of tumors in PC3-bearing Balb/c nude mice analyzed by a change in cell proliferation and apoptosis parameters [32]. Therefore, having such a combination of conventional drugs along with phytochemicals could serve great potential in synergistically fighting cancer.
Curcumin, obtained from Curcuma longa (known as turmeric), is a yellow-colored pigment mainly used as a traditional spice and food coloring agent [33,34]. Curcumin has a unique role in protecting biomembranes against peroxidative damage [35]. Several studies have reported that Curcumin (12 g/day for three months) activates apoptotic pathways against various cancer cell lines, including kidney, colorectal, prostate, pancreatic, and breast, showing antiproliferative activity [33]. Curcumin at a dose of 0.1–3 mg/kg body weight was shown to decrease the enzyme, telomerase reverse transcriptase, and decreased Bcl-2 expression. According to earlier studies, Curcumin’s low stability and insolubility in water have been blamed for reducing its bioavailability. Therefore, nanoencapsulation of Curcumin emerged as an effective strategy to enhance its therapeutic efficacy and bioavailability in combination with conventional chemotherapeutic agents [36]. With some selected phytochemical combinations (resveratrol, quercetin, epigallocatechin-3-gallate, and piperine), Curcumin exerts synergistic effects in cancer treatment to improve its clinical efficacy [37]. Fig. 2 represents a molecular mechanism of anticancer action of Curcumin.
Figure 2: Molecular mechanism of anti-cancer action of curcumin [46].
Naringenin is an essential anticancer polyphenol. It mainly occurs in citrus fruits like lemons, oranges, tomatoes, etc. Naringenin generally induces cytotoxicity and apoptotic effects, thereby preventing the proliferation of different cancerous cells. Naringenin also helps downregulate the levels of pro-inflammatory mediators such as ICAM-1, COX-2, TNF-α, etc. Despite being a valuable phytochemical to target cancer, its in vivo bioavailability is very low due to its hydrophobic nature. Therefore, to overcome this implication and to enhance its bioavailability, the phytochemical in a delivery system at a nanoscale range was formulated [38]. Akhter et al. reported that naringenin-loaded PLGA polymeric NPs increased the cytotoxicity in pancreatic cell lines compared to only naringenin [39]. Another study also reported the beneficial effects of the phytochemical nano-based formulation; Yildirim et al. have established that intelligent polymeric NPs carrying naringenin (SPNPs) improve bioavailability and increase therapeutic effects in breast cancer. Moreover, SPNPs positively affect cell cycle arrest and apoptosis induction in breast cancer cells [40].
Quercetin belongs to the family of flavonoids. It is an excellent antioxidant by affecting ROS, glutathione, enzyme activity, and regulation of various signal transduction pathways, including AMPK, MAPK, and NRFB. Quercetin exhibits anticancer potential by inducing (a) cell cycle arrest G2/M phase in various cancer cell lines like U937, 232B4, SKOV3, etc.; (b) intrinsic apoptotic pathway; (c) autophagy and (d) inhibitory effects on angiogenesis [41]. Lakshmi et al. have reported the anticancer therapeutic implication of quercetin-mediated gold nanoclusters in lung cancer cells and established that nanoclusters have more significant and better cytotoxicity toward cancerous cells. Moreover, it also abolishes ROS generation stating antioxidant activity [42]. The Elsayed et al. [43] have documented the positive effects observed in vivo of quercetin encapsulated chitosan functionalized copper oxide nanoparticles (NPs) in breast cancer. These effects were attributed to the induction of apoptosis, mediated by an upregulation of the p53 gene, ultimately resulting in the death of mammary cancer cells. In addition, it concurrently downregulates the PCNA gene, resulting in a decrease in the rate of proliferation of cancer cells.
Kaempferol is an aglycone flavonoid yellow-colored compound abundantly found in various plant parts, including fruits, leaves, seeds, etc. It exhibits anticancer potential by triggering cell death, arrests in the cell cycle at phase G2/M, and downregulating the PI3K/AKT signaling pathway. Moreover, it also possesses antioxidant activity by reducing ROS metabolism, disrupting molecular mechanisms of proteins associated with cancer-related expression, including Nrf2 and Keap1 [44]. Several studies have reported the beneficial roles of kaempferol in targeting cancer. Govindaraju et al. investigated and reported that gold nanoclusters of kaempferol are cytotoxic to lung cancer cells characterized by nuclear damage. Moreover, kaempferol gold nanoclusters also inhibited the proliferation and migration of cancerous lung cells [45].
Aghazadeh et al. [47] reported that kaempferol-based nanostructured lipid carriers (KNLC) moderately inhibit the proliferation of breast cancer cells. In addition, combining KNLC with paclitaxel shows a synergistic effect leading to the strengthening of apoptosis and arrest in the progression of the cancer cell cycle at the sub-G1 phase.
Myricetin is a flavonoid mainly found in teas, berries, wines, etc. The anticancer activity of myricetin is mainly due to a reduction in MMP-2/9 activity. Reduction in mTOR activation induces apoptosis and downregulates MDR-1 [48]. Khorsandi et al. found that myricetin-loaded solid lipid NPs diminish colony formation in colorectal cancer cells. Moreover, it also elevates the expression levels of Bax and AIF while decreasing the levels of Bcl-2. It also helps in decreasing MMP [49].
Rutin is a unique antioxidant flavonoid in cereals, vegetables, and fruits. It serves as a promising natural phytochemical in the prevention of cancer by following molecular mechanisms; (a) modulation of cancer signaling pathways like MAPK, PI3K/Akt, JNK, etc., (b) inducing apoptosis by stimulating NF-kB pathway and cell cycle arrest in G2 and G1 phase by modulating GSTP1 and Cyp1A1 polymorphism, (c) reducing MMP [50]. Saleemi et al. have shown that zinc oxide NPs with bioflavonoid rutin possess anticancer effects against breast cancer cells by having higher antiproliferative activity than pure rutin. The primary molecular mechanism behind cytotoxicity is the release of intracellular zinc ions and ROS species production [51]. Additionally, head and neck cancer cells were said to resist the anticancer actions of rutin nanocrystals. In addition to this, it also decreases mRNA expression of Bcl-2 and activates a mitochondrial-dependent apoptotic pathway in cancerous cells [52].
Epicatechin is a flavonoid prominently found in black and green tea consumed globally. It possesses anti-tumour and chemopreventive properties due to its oligomeric structure. Epicatechin modulates various cancer pathways, including inhibition of the HGF/Met signaling pathway in breast cancer cells. Moreover, it also suppresses MMPs and other downregulating extracellular scaffolds, resulting in antiangiogenic activity [53]. Perez-Ruiz et al. have demonstrated the beneficial effect of encapsulating epicatechin with lecithin-chitosan NPs against breast cancer cells by inhibiting cell proliferation and encapsulating epicatechin as NPs increase the cytotoxic activity of epicatechin itself [54].
Phenolic compounds have shown significant effects as cytotoxic phytochemicals against cancer, including induction of apoptosis and antiproliferative activity, and target various aspects of cancer (metastasis, proliferation, differentiation, and angiogenesis). Benzoic acid and phenolic acid are the major phenolics well-versed in different in vitro and in vivo models [55,56]. The chemical structures of various anticancer phyto-constituents are represented in Fig. 3.
Figure 3: Chemical structures of various anticancer polyphenols.
Alkaloids are naturally occurring essential compounds mainly of plant-based origin and are found particularly in specific families of flower-bearing plants. Alkaloids contain a cyclic structure with at least one essential nitrogen atom [57]. Various alkaloids (Fig. 4) are well-versed and studied worldwide as potential chemotherapeutic agents, including vinblastine, vincristine, camptothecin, piperine, berberine, etc. Vinblastine (Indole alkaloid) exhibits cytotoxic activity against several cancer cell lines by activating a cascade of proteins in the apoptotic signaling pathway, thereby inducing apoptosis, necroptosis, and autophagy [58]. Vinblastine is characterized as an autophagy maturation inhibitor; it enhances apoptosis in the human colon (LS174T) and human hepatocarcinoma (HepG2) cell lines synergistically in combination with nano liposomal C6-ceramide leading to the accumulation of autophagic vacuole and reducing autophagy maturation of the cells. Another indole alkaloid, Arctigenin, targets the senescence of cells in gall-bladder cancer by remarkably reducing the expression of EGFR levels through rapidly accelerated fibrosarcoma (RAF)-mitogen-activated protein kinase (MEK)-extracellular-regulated kinase (ERK) signaling pathway. It also induces cell death and arrests cell-cycle progression at G1/G0 phase by downregulating the levels of EGFR [59].
Figure 4: Chemical structures of various anticancer alkaloids
Various isoquinoline alkaloids possess antiproliferative activity by autophagy-influenced apoptosis mediated through the ATG5 gene. Berberine, an isoquinoline alkaloid, reduces colon tumor development by inhibiting the cyclooxygenase-2 (COX-2) enzyme. It exhibits anti-tumor activity against EAC cells by inhibiting the biosynthesis of RNA, DNA, and protein in vitro [60]. According to Wang et al., it has been suggested that berberine demonstrates an immunomodulatory impact, augments the cytotoxic properties of NK92-MI cells, and hinders tumour immune evasion through the reduction of PD-L1 expression [61]. Pyrrole alkaloids also exhibit anticancer activity by activating apoptosis, autophagy, and cell necrosis. One of the pyrrole alkaloids (BMA-155CI) was reported to have anticancer potential by modulating apoptosis and autophagy by increasing the expression of various proteins levels, including p65, NF-kB, LC3B, Bax, and Beclin-1 in HepG-2 cell lines [60]. Phenanthroindolizidine alkaloids refer to a class of alkaloids that possess a phenanthrene ring fused with a saturated indolizine ring. Examples of such alkaloids include antofine, tylocrebrine, tylophorine, and tylophorinine. Antofine is a promising phytochemical with potential anti-cancer properties, as it has been observed to induce cell-cycle arrest specifically at the G0/G1 phase. Additionally, it has been found to inhibit the expression of cyclin-dependent kinases (CDKs) and cyclins, specifically cyclins E and D1.
Moreover, antofine also inhibits β-catenin/Tcf transcriptional activity in human colon cancer cells (HCT 116), promoting TNF-α-induced apoptosis [60,62]. Another essential class of organic compounds is steroidal alkaloids (SAs) like solanidine, conanine, etc. These are defined as secondary metabolites that play a significant role in the defense mechanism of plants, with no role in growth, reproduction, and development. The prime mechanism of action of SAs is the activation of apoptotic cell death in a particular cancer cell line, which includes briofilin, which enhances the expression of Bax protein levels, suppresses levels of caspase-3 and Bcl-2 along with segmentation of poly (ADP-ribose) polymerase-1 (PARP-1) in HeLa cells. The biomolecular mechanism underlying the SAs antiproliferative activity might be due to the inhibition of proteins like AKT and MMP-2/9 or by targeting various cell signaling pathways that permits the development of cancer cells [57,63].
Essential oils (EOs) and terpenoids (Fig. 5) are multifunctional phytochemicals with a complex structure of plant origin, which have been used for the past several decades because of their biological importance in the treatment and prevention of various diseases. Terpenoids are divided into a variety of categories according on the quantity of isoprene units they contain [64,65]. Thymol, a monoterpene, have been reported to exhibit anti-cancer effect in various type of cancers cell lines like HL-60, and PC3 human prostate cancer cells [66,67]. Research related to the therapeutic efficacy of EOs in anticancer therapy is relatively new [68]. Recently, studies have reported that enantiomer (+)-citronellal EOs obtained from the plant Corymbia citriodora and Cymbopogon nardus, is also act as a potential microtubule-disrupting agent for the treatment strategy related to cancer [69–71]. Rajiv Gandhi and colleagues reported that chitosan loaded essential oil nanoparticles possess greater cytotoxicity in A549 human lung cancer cell lines compared to simple essential oil treatment [72]. Paclitaxel, a mitotic inhibitor agent, which was originally derived from the bark of the tree Taxus brevifolia acts as a potential anticancer agent by inducing mitotic arrest via targeting the cytoskeleton component tubulin, thereby activating the mitotic checkpoints and subsequently leading to apoptosis [65]. Another study from Najjari and colleagues stated that essential-oil loaded nanostructured lipid carriers obtained from the gums of Pistacia atlantica Desf. decreases cell viability of SKBR3 breast cancerous cells by inducing cell arrest and apoptosis [73]. Thymoquinone, an EO isolated from Nigella sativa has also been discovered to show promising anticancer activity by various mechanisms [74]. A number of studies have been done in order developed an effective novel thymoquinone based phyto-nanophytoformulation, out of which some of the liposomal and polymeric nanoformulation are FDA approved [75–77].
Figure 5: Chemical structures of anti-cancer essential oils and terpenoids.
Influence of Nanotechnology on Phytoconstituents
Phytochemicals generally reach the target site inefficiently due to their low levels of solubility, poor penetration through biological membranes, target specificity, and stability, leading to poor bioavailability in the body [108,109]. This is the main challenge regarding translating phytochemicals’ therapeutic activity to clinical settings [109]. Based on their structural configurations, phytochemicals can be broadly divided into the following classes: alkaloids, terpenoids, flavonoids, and polyphenolic compounds. These various substances, either as substrates, cofactors, or inhibitors of enzymatic reactions, exhibit rich anticancer and cytotoxic activities like phenolic acids (polyphenols); pomolic acid, ursolic acid, oleanolic acid, fomitellic acids, boswellic acids, avicins (triterpenoids); kaempherol, myricetin, quercetin, rutin (flavonoids); and matrine and sanguinarine (alkaloids) as discussed above [110].
The abundance of nanotechnology has a significant impact on phytochemical delivery in many different ways. They have influenced the nanocarrier system from outside as a ligand or delivered to the target site in encapsulated form alone or with synthetic therapeutic agents. Nanosized delivery systems can be organic delivery systems like liposomes and polymeric NPs or inorganic delivery systems like silver, gold, and copper. Phytochemicals could form NPs as a bio-friendly capping agent in green chemistry. Such NPs proved more biocompatible, stable, less toxic, and highly efficient with anti-oxidative properties [108]. In green synthesized nanotechnology, when phytochemicals are entangled or enclosed in the silver NPs have been proven effective for anticancer properties, like the methanolic extract of Vitex negundo L., Acalypha indica, Andrographis echioides, Premna serratifolia leaves, Erythrina indica, and Annona squamosa seed extract. Phytochemical-based metallic NPs impart their anticancer activity by disrupting the lysosome and cell membrane integrity, blebbing, signal transduction, stimulation of signaling pathways, oxidation of lipid and proteins with reactive oxygen species, enhanced mitochondrial activity, with and shrinkage of a nucleus that leads to apoptosis [111]. Anticancer results were analyzed and proved on human hepatoma, Dalton’s lymphoma cells, HeLa cells, and breast cancer cells (MCF-7) with these phytochemicals [110]. Medicinal plants and their components are used in the green synthesis of NPs prominently with silver and gold ions due to their flexible and reliable binding properties to thiol and amine groups, enabling them for surface plasmon resonance (SPR) against cancer [109]. In metallic NPs, iron oxide NPs with seaweed (Sargassum muticum), titanium dioxide NPs, cerium oxide NPs, gold-platinum (Au-Pt), and silver–selenium (Ag-Se) bimetallic NPs also showed anticancer activities with quercetin and gallic acid [111]. In a recent study conducted by Pushparaj et al., silver nanoparticles were synthesised using an aqueous extract derived from the leaves of Solanum melongema. The resulting nanoparticles exhibited remarkable efficacy in inhibiting the growth of human MDA-MB-231 breast cancer cells, thus demonstrating promising anticancer activity. Additionally, it demonstrated antibacterial efficacy against various strains including E. Coli, Pseudomonas aeruginosa, and Shigella flexneri etc. This study demonstrates that the utilisation of green synthesis methods for the production of metallic nanoparticles holds promise as a potential strategy for cancer treatment [112].
Such NPs act more efficiently against MCF-7 breast cancer, colon cancer, hepatocellular carcinoma (HepG2 cells), and lung cancer when they have A. leptopus, Cassia tora, Gymnema sylvestre leaf extracts, and Moringa oleifera flower aqueous extract, respectively. Apart from NPs, single-walled CNTs (SWCNTs) also represent promising carriers for phenolic acids and Curcumin [2]. For topical delivery, transferosomes transport phytoconstituents like Curcuma longa extract, capsaicin, vincristine, and colchicine in a sustained release pattern with more improved bioavailability as they deformed the subcutaneous layer of the epidermis, thereby giving deeper penetration [111]. Another advancement in anticancer chemotherapy is the combination of phytoconstituents with cytotoxic agents that the National Cancer Institute (USA) encouraged to combat multidrug resistance (MDR) undesirable side effects by using the potential antitumor activities of plant extracts.
Various Types of Phyto-Based Nanoformulations
Liposome is a microscopic lipid vesicle consisting of an inner aqueous core and an outer bulk separated by a thin lipid bilayer. Liposomes are estimated to range from 10 nm to 1 m or more significant. The inner aqueous core facilitates the targeted delivery of vaccines, enzymes, proteins, and drugs to the intended site. Stealth liposomes are superior to transferosomes in site-specific targeting and delivering drugs across rigid barriers. Besides their biocompatibility, biodegradability, and amphiphilicity, liposomes can encapsulate polar and nonpolar therapeutics [113]. A mucoadhesive polymer can be applied to the surface of liposomes to deliver targeted drugs, or liposomes can be ligated to facilitate drug delivery. The work by Shu et al. aimed to treat myelogenous leukemia by employing liposomes laden with betulinic acid to target HepG2 cells. Mannosyl erythritol lipid-A (MEL-A) was added to the surface of liposomes during their creation in order to improve transfection effectiveness and make it easier for DNA to bind to liposomes. Surface-modified betulinic acid liposomes with MEL-A augment the cross-way permeation of these liposomes into the cells. This ultimately paves the way for faster cell apoptosis. In addition to enhanced anticancer activity, the G1 phase blockage was also responsible [114].
Likewise, curcumin nanoliposomal delivery shows advanced antitumor potential. Curcumin nanoliposome formulations (PG-LipCUR and LipCUR) actively eliminated breast cancer cells (TUBO and 4T1). Compared to free CUR, liposomal curcumin has a longer half-life based on the pharmacokinetic study. Because of the fusogenic properties of the lipids used, in the biodistribution study in mice, both nano liposomal formulations showed more significant tumor accumulation than pure curcumin [115]. Differential nano-formulation approaches have emerged to address these challenges. As a result of these studies, Greil et al. investigated the efficacy of increasing liposomal curcumin doses (Lipocurc TM) in metastatic cancer patients. During phase I trials, over 32 patients were treated with liposomal curcumin, with a dose of 300 mg/m2 liposomal curcumin over 6 h reported as the most tolerated dose by patients [116]. These lipocurcumin formulations were proven harmless, and ceramide generated upon pretreatment significantly alleviated cancer markers through apoptotic and cell-cycle arrest phenomena.
Further, in contrast to the parent curcumin formulation, the liposomal formulation showed a significantly higher dose-effect profile with minimal harm to the patients. However, LipocurcTM contains curcumin polyphenols, which include DMPC (2:0-1, 2-dimyristoyl-sn-glycero-3-phosphocholine) and DMPG (2:0-1, 2-dimyristoyl-sn-glycero-3-phosphorylglycerol), which protect against cancer. Patients with cancer experienced a significant increase in curcumin plasma levels after receiving the liposomal carrier intravenously. Consequently, the excretion rate of the drug and half-life are also lower than those of physically well-being individuals upon dose withdrawal. In a phase-I study using liposomal curcumin in metastatic tumor patients, they obtained steady plasma concentrations with a substantial but brief reduction in tumor markers, including CEA (Carcinoembryonic antigen), PSA (prostate-specific antigen), and CA 19–9 (cancer antigen). Additionally, the sphingosine kinase inhibitory effect of liposomal curcumin implies that it may be used alone or in combination with other cancer treatments to lessen the severity and frequency of cancer recurrences [117]. AbouSamra et al. formulated rutin loaded liposomes for the treatment of hepatocellular carcinoma. It was observed that developed formulation effectively enhanced the solubility and biological activity of rutin. Moreover, to prepare the desirable liposomes, β-sitosterol was used as an alternative to cholesterol and thus, termed it as “Phyto-stereosomes”. This study provides evidence supporting the potential therapeutic efficacy of nano-encapsulated Rutin within this novel vesicular nanoformulation designed to promote its action against hepatocellular carcinoma [118].
An innovative drug delivery system, niosomes use nonionic surfactants to deliver therapeutics. Surfactant molecules are two-fold in structure, which encourages them to form a bilayer structure. Two surfactant layers are formed by hydrophilic and hydrophobic ends arranged in external and internal locations. In a unique carrier, two kinds of drugs are encapsulated by this lamellar morphology. Several factors include surfactants, cholesterol levels, critical packing parameters, drugs used, and shape niosomes are need to be optimized for efficient drug delivery. For drug delivery, these systems can be designed to be administered orally, parenterally, topically, and so on. Although liposomes resemble niosomes in structure, niosomes are more stable and cost-effective than liposomes. Unlike ions, niosomes remain in the bloodstream for quite some time, making them an excellent drug-delivery vehicle [119]. Zare-Zardini et al. evaluated the in vitro test on Ginsenoside Rh2- loaded niosomes and prepared two formulations: 5% DOTAP-loaded niosomal formulation and DOTAP-free niosomal formulation. Additionally, cholesterol and Span 60 were included in niosomal formulations, and DOTAP was used as cationic lipid. However, DOTAP-loaded nano-niosomal formulations containing Ginsenoside Rh2 had average size, PDI, zeta potential, and encapsulation efficiency of 93.5 ± 2.1 nm, 0.203 ± 0.01, + 4.65 ± 0.65 mV, and 98.32% ± 2.4%, respectively. It was found that niosomal vesicles have a round shape and smooth surfaces. Niosome release of Ginsenoside Rh2 followed a biphasic pattern. It was demonstrated that adding DOTAP to niosomal formulations enhanced cellular uptake compared to DOTAP-free niosomal formulations. Furthermore, it also improved cellular uptake and cytotoxic activity in PC3 cells [120]. Barani and team designed and evaluated Carum-loaded Niosomes targeting breast cancer. A chemical compound known as thymoquinone (TQ) is present in the Carum carvil (C. carvil) seeds. TQ has many applications in medicine, including cancer therapy. Although TQ is hydrophobic, it is poorly soluble, permeable, and bioavailable in biological mediums. Hence, it was designed to minimize these drawbacks by combining Ergosterol (herbal lipid) with Carum carvil extract (Carum) and non-ionic surfactants. However, two formulations of noisome containing TQ and Carum was prepared and evaluated. A niosome loaded with TQ and Carum was showed an entrapment efficacy such as 92.32% ± 2.32% and 86.25% ± 1.85, respectively. As compared with free TQ, both formulations provided a controlled release. Loaded niosomes exhibit more significant anticancer activity against MCF-7 cancer cells, according to the results of the MTT experiment and a flow cytometric study. Interestingly, Nio/TQ, Nio/Carum, and TQ formulations showed arrest in the G2/M cell cycle and a decline in the migration of MCF7 cells. TQ and Carum-loaded niosomes illustrated appreciable encapsulating power for poorly soluble phytoconstituents and could be utilized as a carrier to enhance site-specific drug targeting in breast cancer treatment [121].
Ethosomes are elastic NPs based on phospholipids. The ethosomal system primarily comprises phospholipids, water, and high ethanol concentrations (20%–45%). Among the benefits of ethanol is that it enhances skin penetration and is a rich permeation enhancer [122,123]. Since ethosomes have improved skin permeability, they can be used as drug nanocarriers to treat skin cancer. The ethanol composition may penetrate deeper into the skin because it provides the vesicles with the characteristics of soft elastic nature. Due to its ability to affect the two-layered structure of stratum corneum, ethanol may enhance drug penetration in phospholipid vesicles [124]. Moreover, recovering the fluidity of the lipid membrane of the skin. A unique characteristic of ethosomes is that they act as a shield from the outer environment, simultaneously helping move the drug across the different layers of skin and timely releasing the drug [125–127]. In this way, skin cancer treatment was expected to benefit from effective drug delivery. Furthermore, Lin et al. investigated the effects of ethosomes enclosing evodiamine (EVO) and Berberine chloride (BBR) [128]. It aimed to develop a novel method of single-step injection for topical anti-melanoma therapy. Propylene glycol (PG) cholesterol and soybean lecithin (SPC) cholesterol are formed in different amounts. Ethosome particle size increases as SPC (180 mg) increases (227 ± 6 nm). However, EVO and BBR were entrapped more than 90% efficiently by the ethosomes formulation. The in vitro findings verified that the formulation (Ethosome/BBR-EVO) reached the epidermis layer of the skin. They also enhance penetration as well as drug delivery. Overall, the formulation was effective in drug delivery against skin cancer [128]. According to the studies discussed above, PTX (Paclitaxel) was found extended drug release to the target when loaded into ethosome [129]. In addition, curcumin-loaded ethosomes were compared with PTX-loaded ethosomes developed in-house. Curcumin-loaded ethosome deposited more than 60% of the drug on the skin, while PTX-loaded ethosome deposited less than 60% [130,131]. The release profile of CUR gel and PTX gel was 12 and 16 h, respectively. Nevertheless, the prepared ethosomal gel needed to be studied pre-clinically and clinically. It can be concluded that ethosome is an effective system for vesicular drug delivery. In this way, the drug is loaded more efficiently into the skin and the permeability of the drug is increased. Furthermore, ethosomes have a high zeta potential, which indicates independent repulsion, thereby preventing aggregation or fusion due to electrostatic interactions.
A micelle is a nanoscale material formed from the aggregation of amphiphilic molecules in an aqueous environment above the critical micellar concentration. In most cases, micelles consist of an amphiphilic block copolymer and a core-shell structure. Nanomicelles possess unique properties, potentially holding water-soluble and insoluble solutes [132]. The nanocarriers were highly influential in loading chemotherapeutics explicitly formulated to target ovarian cancer sites [133,134]. A micelle with a size between 10 and 100 nm is more porous, more likely to be endocytosed by ovarian cancer cells, and less likely to target normal cells [135]. Among nano micelles, remarkable properties are in vivo stability, enhanced biocompatibility, sustained plasma circulation, perforation of inflammation, and incorporation of hydrophobic chemotherapeutics. Previous reports described using immunotherapy against melanoma based on lipid-based Trp2 peptide vaccines by Lu and his team. In tumors, suppressive immune microenvironments hinder vaccine therapies. Using curcumin (CUR) as a vaccine enhancer will remodel the tumor microenvironment to enhance its effectiveness. CUR-PEG (polyethylene glycol), a curcumin micelle, was administered intravenously to treat the tumor. Vaccine treatment and CUR-PEG treatment combined had a synergistic antitumor effect in B16F10 mice compared to their personal effects. According to in vivo data, the combination therapy significantly increased CTL (Cytotoxic T lymphocyte) responses, found 41.0 percent more or less than 5% for specific killing, and IFN-γ production resulted in a 7-fold increase. The combination therapy, including MDSCs and Treg cells, IL-6, and CCL2, significantly decreased several immunosuppressive factors. There was also a significant increase in pro-inflammatory cytokines, including TNF and IFN (interferons (IFNs) and tumor necrosis factor (TNF), as well as CD8+ T cells, as a result of the combination therapy. Results showed that tumors were switching from M2 to M1 phenotypes. CUR-PEG and vaccine significantly decrease the STAT3 activity (76%). Lu et al. suggested that CUR-PEG might be promising for improving immune therapy for advanced melanoma [136]. Patra and the team formulated a blend of polymeric micelles utilizing quercetin (QCT) to target different cancer types. Indeed, QCT, a flavonoid, is an excellent source of antioxidants that exhibit cancer-protective and anti-proliferative properties. However, due to their water-insoluble nature, they are less active. Therefore, the mixed polymeric micelles (MPMs) method and their physicochemical properties were examined. These MPMs demonstrated anticancer activity in ovarian (SKOV-3 and NCI/ADR, epithelial and multi-drug resistant cell lines, respectively) and breast cancer (MCF-7 and MDA-MB-231). According to in vitro experiments, MPMs released QCT more slowly than free QCT. When compared with pure QCT, QCT had a markedly improved solubility. Due to their low critical micelle concentration, MPMs have high stability in aqueous media. In all cancer cell lines, the concentration that inhibited 50% growth (IC50) was significantly lower for both micellar preparations than free QCT. There is potential for further development of MPMs containing QCT, which may be effective for treating cancer types ranging from cancerous to non-cancerous [137].
Fig. 6 provides an illustration of above discussed vesicular systems for the delivery of phytocostituents.
Figure 6: Illustration representing various vesicular systems for the delivery of phyto-constituents
Polymeric NPs are 1 to 100-nm-sized particles typically loaded with active compounds entrapped inside or surface-adsorbed onto the polymer core. NPs are classified as either nanocapsules or nanospheres, depending on their morphology. Several diseases can be treated with polymeric NPs for targeted drug delivery. As drug carriers, polymeric NPs have several advantages. They can control the drug release from the reservoir, protect drugs and other molecules from the environment, and improve their bioavailability and therapeutic index [113,114,116,138]. An herbal-based nano-formulation delivers targeted drug delivery to minimize the side effects of anti-cancer drugs and conventional drug delivery systems. Hence, A. absinthium extract-loaded polymeric NPs (NVA-AA) were formulated and evaluated against breast cancer (MCF-7 and MDA MB-231), also focused on a protein that targets cytotoxicity [139].
Three monomers were co-polymerized to create poly-meric NPs in the preparation, namely N-vinylpyrrolidone-acrylic acid (NIPAAM), N-vinylpyrrolidone (VP) and acrylic acid (AA). Synthesized NPs had a size of 131.4 and 19.7 nm, and the PDI was 0.1 ± 0.03 at 25 degree Celsius. As a result of TEM analysis (Transmission electron microscopy), the synthesized NPs had a spherical shape and were highly monodispersed, having an average size of 110 nm × 12.6 nm. However, the IR spectra showed broad, intense peaks at 3280.35 and 1636.78 cm−1. As a result, it confirmed the polymerization of NIPAAM with VP and AA. Against MCF-7 and MDA MB-232, whole plant extract exhibited more significant cytotoxicity than different parts of the plant (with lower IC50 values of 307.16 × 20.4 g/mL and 338.55 × 15.7 g/mL, respectively). To conduct further research, the formulation found an entrapment efficiency of 84.8% and a loading capacity of 21.2%. LCMS/MS and STRING analysis identified the protein targets and their interactions, validated for using qPCR and BLI. The LCMS/MS analysis demonstrated that the cytotoxicity was caused by modifying protein molecules implicated in vesicular transport, apoptosis, proliferation, and metastasis. Additionally, vesicular trafficking and apoptosis networking are connected by UBA52 in MCF-7 and TIAL1, PPP1CC in MDA MB-231 cells, producing effective results against breast cancer [139]. Fang, and team designed charge-reversing NPs that could effectively target the cells’ mitochondria while responsive to different pH environments. A comparison was made between vitamin B6-oligomeric hyaluronic acid-di-thiodipropionic acid-berberine/curcumin (B6-oHASS-Ber/CUR) and vitamin B6-oligomeric hyaluronic acid-di-thiodipropionic acid-berberine/curcumin (oHA-SS-Ber/CUR). The lipophilic cation Berberine (Ber) was conjugated with oligomeric hyaluronic acid (oHA-SS-Ber) through disulfide bonds. As a result of conjugating B6 with oHA, B6-oHA-SS-Ber was synthesized using the dialysis method and produced two types of Cur-loaded NPs (CUR-NPs). In tumor tissue acidic microenvironments, B6 pKa permits its charge to move from a negative to a positive one, making it more effective at targeting mitochondria. In transmission electron microscopy (TEM), B6-oHA-SS-Ber/Cur micelles could self-assemble in water and form spherical NPs with a hydrodynamic diameter of 172.9 ± 13 nm. Studies comparing oHA-SS-Ber/Cur micelles with B6-oHA-SS-Ber/Cur micelles showed that B6-oHA-SS-Ber/Cur micelles showed better cytotoxicity, lysosome escape, mitochondrial distribution, and cellular uptake. Micelles containing B6-oHA-SS-Ber/Cur exhibited effective effects on tumour growth in vivo [140].
Solid lipid nanoparticles (SLNs)
As alternatives to the traditional colloidal delivery systems, the SLNs are promising in providing a blend of NPs and liposomal systems while being relatively non-toxic. Biocompatible lipids are used in SLN to provide a highly tolerable safety profile. Drug and gene delivery can be enhanced by integrating SLNs with hydrophilic, lipophilic drugs, proteins, and nucleic acids [141]. Akanda et al. designed transferrin (Tf)-conjugated solid lipid NPs (Tf-SLNs) loaded with curcumin for active prostate cancer cell targeting. Cancer mortality from prostate cancer is one of the leading causes of death in men worldwide and one of the most challenging to treat. However, in vitro unloaded Tf-SLNs demonstrated less cytotoxicity, while Tf-Cur-SLNs exhibited effective anti-proliferative action. Furthermore, Tf-Cur-SLNs showed a significant increase in cellular uptake (p < 0.05/=0.01) compared to unconjugated SLNs. While compared to Cur-SLNs and Cur solution, bioconjugated Tf-Cur-SLNs also improved in primary and late apoptotic populations. In contrast to the control group, the Tf-Cur-SLNs significantly reduced the size of tumors in mice bearing prostate cancer (392.64 mm3 after 4 weeks, p < 0.001). SLNs bio-conjugated with bioactive molecules could potentially cure cancer in vivo, based on the findings of this study [142]. In another study, researchers developed Silibinin loaded (SLNs) for targeting lung cancer. According to the results, the developed formulation indicated that they are suitable for inhalation. The Silibinin loaded- SLNs were effective against A549 cells, based on in vitro cellular efficacy. Inhalable SLNs that could be given to the lungs without complication were successfully introduced with this method [143]. Farhadi et. al., fabricated SLNs loaded with chrysin and subsequently functionalized the nanoparticles by conjugating them with folate-bound chitosan. The prepared demonstrated inhibitory effects on PANC, MCF-7, A2780, and HepG2 cell lines, which are known to be malignant. Furthermore, the prepared formulation exhibited the ability to scavenge free radicals, while also demonstrating the capability to inhibit angiogenesis [144]. Therefore, it can be regarded as a potentially auspicious candidate for preclinical and clinical investigations in the context of pancreatic cancer.
Gold NPs have the potential to act as drug carriers in recognition of their optical, SPR, and tunable properties. They have an extensively broader size range available from 1 to 150 nm. Gold NPs are easily adjustable due to their negative charge and can efficiently hold ligands, drugs and other biomasses. Furthermore, gold NPs are practically safer, biocompatible non-reactant materials that are becoming preferable as drug-carriers [145,146]. Lee interestingly encapsulated gold NPs in chitosan and designated spherical, star and rod shapes to these NPs for understanding the effects of different shapes on cytotoxic potential and cellular uptake of gold NPs. The reduction of gold salts into gold nanospheres was achieved through green tea extract. To prepare gold nanostars, as-prepared nanosphere solutions were used as seed solutions. A conventional method was used to synthesize gold nanorods. According to FTIR studies, the −OH functional groups are formed when polyphenols are oxidized to C=O while Au salts are reduced to AuNPs. The presence of C=O functional groups in nanospheres has demonstrated oxidation of –OH functional groups during synthesis, as demonstrated by their findings. A UV-visible spectrophotometry study revealed that all three gold NP types (nanospheres, nanostars, and nanorods) have characteristic surface plasmon resonance bands, with nanospheres showing 537 nm, nanostars 600–800 nm and nanorods 797 nm, based on zeta potential of 190.7 nm, nanostars 123.9 nm, and nanorods 33.23 nm. Accordingly, the increase in zeta potentials for the nanospheres and nanostars indicates successful capping with chitosan.
Additionally, the nanorods’ zeta potential was increased, suggesting that their surfaces were capped with chitosan. The lattice structures in all three shapes were visible in high-resolution transmission electron microscopy images, indicating that the NPs are crystalline. Typical particle dimensions of nanospheres were 8.7 nm × 1.7 nm, nanostars were 99.0 nm × 47.0 nm, and nanorods were 60.4 nm × 16.4 nm. Among nanorods, nanostars, and nanospheres, nanorods had the highest cytotoxicity. The human hepatocyte carcinoma cells (HepG2) have displayed varied cellular update capacities of gold NPs ranging from the highest uptake by nanospheres to the slightly lower uptake by nanorods to the lowest being with the nanostars [147]. This study has provided a different perspective on selecting the appropriate shapes of gold NPs for achieving better therapeutic profiles of the loaded drugs. Lupeng Li and colleagues developed gold NPs of Marsdenia tenacissima (MT) for targeting liver cancer HepG2 cells. For thousands of years, Marsdenia tenacissima has been used in Chinese medicine to treat respiratory problems, asthma, and rheumatism. The FTIR analysis shows that the MT-AuNPs show peaks at 3331.43 and 1637.27 cm−1, corresponding to the O–H and C=C stretching modes. MT-AuNPs at 59.62 × 4.37 g were found to be cytotoxic after 24 h. The migration assay of MT-AuNPs confirmed the induction of apoptosis, changes in MMP and generation of ROS. Up-regulation of apoptotic proteins (Bax, caspase-9 and caspase-3) confirmed the apoptosis phenomenon, and further this was also affirmed by the down-regulation of anti-apoptotic proteins (Bcl-2 and Bcl-XL). The anti-cancer profile of MT-AuNPs against liver cancer was established through this study [97]. Fig. 7 represents advantages of nano-encapsulated phyto-nanoformulations in treatment of cancer.
Figure 7: Advantages of nano-encapsulated phyto-nanoformulations in treatment of cancer.
Clinical Development, and Regulatory Concerns Associated with Anticancer Phytoconstituent’s Herbal Nanoformulations
Various medicinal plants in clinical trials show positive results for their anticancer efficacy. Two approaches are recommended to the sponsors to prove that variations in different batches will have no effect on the clinical response of the botanical medicinal product. The initial approach involves the identification of batch effects on clinical endpoints through the utilisation of multiple-batch analyses. This method allows for the assessment of potential heterogeneity in clinical outcomes, such as subgroup analysis. The second approach, on the other hand, demonstrates that clinical response is not influenced by dosage. It investigates the effectiveness of various doses in comparison to placebo or control, and determines whether they are either superior to placebo/control or non-inferior to active treatment. This demonstrated that as long as batches were regulated within the bounds of specification, the hereditary heterogeneity of chemical components could not effect final clinical outcomes. These days, combinatorial therapy is used with phytocompounds in conjugation with other chemopreventive agents against single drugs to combat the side effects or achieve higher sensitivity or synergistic or additive effects. Multiple-herb preparations (e.g., Shen qu) are also becoming part of the botanical combination drug. The beta error (wrong negative finding) increases with the number of botanicals evaluated in a factorial trial. A five-arm trial (or six if a placebo group is included) comparing ABCDE against ABCD, ABCE, ABDE, and BCDE was presented in the introduction to the proposed updated Combination regulations (21CFR300.50). The granting of waivers by the Agency is permissible under the proposed combination rule in cases where it is impractical to conduct a comprehensive analysis of all constituent elements. If a combination contains multiple active substances without the deliberate intention to combine them, it would not typically be classified as a fixed dose combination under the proposed Fixed-Combination regulations. Additionally, the revised 21 CFR 300.60 suggests that a waiver may be granted under the proposed rule in such cases.
So, it becomes an imperative research area for pharmaceutical formulators and biomedical scientists to integrate green nanotechnology into cancer science for the formulation of superior plant-based antineoplastic agents. Many FDA-approved phytoconstituents with their clinical trials are listed below in Tables 2 and 3.
Any regulatory agency viz. The food and Drug Administration (FDA) and European Medicines Agency (EMA) approves any drug for marketing authorization when it shows significant benefits, statistically outweigh the risk results through all phases of its clinical trials. Although plant-based compounds are considered safer and less toxic than synthetic compounds, but to avoid any unregulated use against different diseases much evidence of their side effects must be reported [148]. Regulation of herbal constituents is challenging as herbal drug’s medicinal constituent profiles generally vary when the same plant species grown in different seasons, processing, growing/cultivation conditions (e.g., soil, light, temperature, humidity, and time of harvesting), differences in plant genetics that leads to variation in chemical composition and biopharmacological activity of botanical raw materials and ultimately standardization of quality, safety and efficacy affected due to lack of data. Therefore, conventional chemistry, manufacture and control (CMC) approaches used for quality control (QC) of small molecules are sometimes not sufficient. It is recommended that sponsors of INDs (Investigational New Drugs) should establish and execute Good Agricultural and Collection Practises (GACP) in accordance with the WHO Guidelines on GACP20 or the EMA Guideline on GACP for Starting Materials of Herbal Origin21. These practices should be implemented at the grower or farm levels to provide guidance for the production of botanical raw materials in specific geographical regions [149]. To ensure batch-to-batch consistency in herbal anticancer drug’s a “totality-of-the-evidence” approach should be adopted that includes chemical identification, fingerprint analysis, quantification of active or chemical constituents, and a biological assay with nonclinical studies and clinical effectiveness showing no interaction with the clinical batches [149].
Another challenge is harmonizing the marketing authorization of herbal anticancer drug among different regulatory agencies. For this specific efforts are taken by the FDA by seeking responses in questionnaires against their guidelines. Now, it is the need of the hour that for standardized and high-quality production of plants with a uniform metabolite profile, we have to focus on biotechnical approaches and genetic studies that declare safe or unsafe once and for all. Next challenge is that unfortunately, all the developed and approved phytoconstituent-based pre-parations are intravenously or subcutaneously administered. The oral route is the most convenient and preferred, so in this connection, research is required to overcome the solubility, acidic and enzymatic degradation, and first-pass effect. The next big challenge is optimization of dose and dosage regimen when a combination of anticancer moieties (chemotherapeutic agents and phytochemicals) are used. The Food and Drug Administration (FDA) has made changes to its regulatory policies in order to streamline the process of developing botanical drugs. This includes the introduction of a proposal for herbal IND applications to be deemed “safe to proceed” (STP) for phase 1 or 2 clinical studies. The basis for this determination is the prior human experience of these botanical drugs, either as traditional medicines (such as Traditional Chinese Medicine or Ayurveda) or through evidence of their marketing experience (such as dietary supplements). This evidence includes factors such as sales volume and reported adverse events. The implementation of a flexible regulatory approach offers the botanical industries various incentives to promote the initiation of early-phase trials for botanical products, without the requirement of additional purification of drug substances or identification of active constituents. Furthermore, this approach allows for the utilisation of existing human data as a substitute for or delay of animal toxicology studies, potentially leading to a reduction in the overall timeline for the development of botanical drugs. In order to acknowledge the intricate nature of botanical drugs and the inherent uncertainty surrounding their active constituent(s), the Food and Drug Administration (FDA) typically adheres to the Botanical Drug Development Guidance for Industry, with particular emphasis on section VI entitled “Investigational New Drug Applications for Phase 3 Clinical Studies.” This approach serves to both endorse botanicals and offer supplementary recommendations for conducting phase 3 clinical trials. After phase 3 trials, changing growth sites or adding new growing sites makes NDA filing more challenging. Prior to submitting an NDA, the FDA advises the sponsor to take into account the prospect of qualifying as many growth sites as possible. However, we hope that with the evolution of new techniques and research scientists will overcome all the challenges to treat resistant cancer in the future.
Challenges of Herbal Nanoformulations
The prevalent cancer treatments have certain limitations like plasticity, which leads to phenotyping switching and then ultimately multi-drug-resistance (MDR) by tumors, intra-tumoral heterogenicity, tumor sites targeting of the therapy, understanding of tumor microenvironment, and insufficient drug circulation times. Besides these, these therapies are associated with cancer drug toxicity, heart problems, and low white blood cell counts.
Nanotechnology is a more advanced and immensely beneficial system than conventional therapeutics approaches. Nanotechnology involves diagnosis and therapeutics strategies in applying structures, characterization, design, devices, production, and systems at the nanometer scale. Nanocarriers have various advantages like small size (10–1000 nm), surface charge, porosity, elasticity, co-delivery of drugs and phytochemicals, enhanced solubility, prolonged systemic circulation, entrapment of lipophilic and hydrophilic drugs, easy penetration through the various biological membranes, protection of the drugs from external physiological damage by encapsulating, enhanced stability during storage by protecting the phytochemicals from oxidation and degradation, resulted into long-term effects, target-specific drug delivery thereby lowering toxicity and increasing the efficacy of the drug/phytochemicals [161]. Nano-delivery systems improve the pharmacokinetics parameters like solubility, half-life, clearance rate, and biodistribution of drug/drugs, thereby creating a therapeutic index. Small-sized NPs (less than 100 nm) are characterized by high drug loading capacity and deep penetration power into tumor tissue more effectively through enhanced vascular permeation than large-sized NPs [110]. Not only size but shape also affects as disc-shaped NPs (nanodiscs) more effectively bind to melanoma cells for more extended periods than spheroid NPs [111].
Further, breast cancer cells more effectively absorb rod-shaped NPs than spherical NPs [2]. At the same time, nanodiamonds are more effective in treating brain tumors, as they remain in the tumor longer, increasing their effectiveness [190]. The enhanced permeability and retention (EPR) effect was achieved via passive targeting. Nanocarriers are leaked through blood vessels near the tumor and retained for extended periods due to poor lymphatic drainage. If the encapsulated phytochemicals are not appropriately released sustainably or controlled, then such delivery systems are useless. For active targeting, nanocarriers are decorated with antibody fragments, peptides, growth factors, and monoclonal antibodies to recognize the overexpressed receptors or antigens by biophysical interactions. MDR is the main problem of cancer therapy that can be overcome by using nanotechnology to deliver phytochemicals.
Antibody-drug conjugates (ADCs) in the nanometer range with small-molecule cytotoxins chemically linked to an antibody that could be an excellent phytochemical carrier. Generally, biocompatible, biodegradable, and amphiphilic polymeric materials are chosen for nanocarriers that can facilitate the encapsulation of polar and nonpolar therapeutics. Some carrier systems are solid particulate types, while others are vesicle types. The particulate type solid lipid NP is widely used within the 10 to 1000 nm size range in biomedical fields. Dendrimer, again a nano- star-shaped polymeric carrier system, quickly accumulates various types of molecules as they have a central core and interior and exterior branches like a tree with multifunctional surface groups. They can also carry biomolecules (proteins and amino acids), drug molecules, and phytochemicals. Gold NPs have unique properties due to the moieties of gold and the NP system. Gold facilitates bonding with amine and thiol groups, preferred for ligand attaching in targeting, while the nano-particulate form provides a large surface area and high stability.
Depending upon the type of polymer, we can decorate it with various conjugated molecules with improved biocompatibility, less toxicity, and controlled and sustained drug release. In magnetic NPs, the magnetic field can be manipulated as controlled externally by varying the precursor concentration. As they generally agglomerate, which can be overcome by silica, polymer, or surfactant molecule’s coating that ultimately enhances dispersibility. The therapeutic efficiency of magnetic NPs in cancer cells is enhanced by lipid coating and targeted thermo-chemotherapy to folate receptors, which remain overexpressed. The carbon nanotubes (CNTs) made of rolled graphene sheets easily accommodate the therapeutic molecules as they have a narrow diameter, larger surface area, and broader length. With surface modification via oxygen-containing functional groups, ligand-targeting is possible [2].
The collaborative research conducted between traditional herbal treatments and nanotechnology, has yielded promising pharmaceutical therapies that hold potential for enhancing human health in the near future. Hence, the anticipated impact of incorporating natural products and herbal remedies alongside nanocarriers is expected to enhance the significance of current drug delivery systems in terms of their effectiveness and value.
While herbal nanoformulation presents certain challenges as discussed in previous section, it also provides an avenue for novel research opportunities [191]. The accumulation of evidence is necessary to support the notion that nanotechnology, specifically phytofabricated nano-carriers, may offer a significant solution to various challenges associated with herbal nanoformulations. Moreover, the global scale-up of these nanoformulations to enhance productivity poses a significant challenge. The tools for assessing the biological efficacy of nanoformulations are currently in the process of development in order to establish a comprehensive framework for evaluating the performance of nanoformulations. This represents a significant challenge that must be addressed in order to effectively navigate the path towards successful implementation of nanoformulations.
Natural substances derived from plants have long been acknowledged as an essential source of anti-cancer medications. During the past 25 years, over 65% of new anticancer medications have come from natural sources. These naturally derived chemicals were produced in vast quantities, and their novel equivalents were created by chemical synthesis (either partial or total). The majority of these chemicals have limited bioavailability and low solubility. Additionally, the non-specificity of chemotherapy has long caused damage to the patient’s normal proliferating tissues, leaving them immunodeficient and with long-term adverse effects.
Regarding this, the emergence of nanotechnology in cancer therapies have shown promise in overcoming the drawbacks of traditional therapeutic regimens, which included barriers of instability, low aqueous solubility, high toxicity, poor absorption, low specificity, and multidrug resistance. The novel delivery systems based on nanotechnology have various benefits, including water solubility, decreased toxicity, biocompatibility, and the ability to have their surfaces modified for other purposes. Better therapeutic response and long-term survival observed in patients who received NPs have further demonstrated this. The potential for incorporating naturally occurring chemicals into nanotechnology-based combination medication formulations that target the tumor microenvironment is enormous. Studies targeted at synthesizing more tumor-targeted nanotherapeutic delivery systems with high-quality and yield cytotoxic compounds drawn from natural resources may improve the general treatment of cancer. It has been determined that combining traditional chemotherapy and plant-based drugs to display synergistic activity can result in superior treatment outcomes. It is extremely challenging for scientists to formulate nanocarriers for phytochemicals combined with chemotherapeutic medicines and optimize the dosage.
Additionally, it is challenging to coordinate their pharmacokinetics and biodistribution to keep a synergistic ratio of drugs at the tumor site. Additionally, despite phyto-nanoformulations showing promising anticancer results in preclinical research, they have not yet been developed for use in clinical settings. However, nanocarriers may exhibit some level of harm in humans based on commercial or therapeutic possibilities, even if this is unproven. Additionally, therapeutically viable phytochemical-based anticancer medicines might be made possible by effective formulation targeting methodologies, evaluation of the targeting effectiveness of NPs, and compliance with international standards for their toxicological and biocompatibility. However, scientists are confident that nanoformulations based on phytochemicals will undoubtedly find a position in the arsenal to cure cancer shortly.
Acknowledgement: The authors are thankful to Delhi Pharmaceutical Sciences and Research University, New Delhi, India, for providing necessary facilities.
Funding Statement: The authors received no specific funding for this study.
Author Contributions: The authors confirm contribution to the paper as follows: Conceptualization: Abhishek Wahi, Madhu Gupta; Writing–Review & Editing: Abhishek Wahi, Mamta Bishnoi, Neha Raina, Meghna Amrita Singh, Piyush Verma, Ginpreet Kaur, Hardeep Singh Tuli, Piyush Kumar Gupta; Data Curation: Neha Raina; Artwork: Abhishek Wahi, Neha Raina; Supervision: Piyush Kumar Gupta, Madhu Gupta; Visualization, Project Administration, Funding Acquisition: Madhu Gupta. All authors reviewed the results and approved the final version of the manuscript.
Availability of Data and Materials: Not applicable.
Ethics Approval: Not applicable.
Conflicts of Interest: The authors declare that they have no conflicts of interest to report regarding the present study.
References
1. Fares, J., Fares, M. Y., Khachfe, H. H., Salhab, H. A., Fares, Y. (2020). Molecular principles of metastasis: A hallmark of cancer revisited. Signal Transduction and Targeted Therapy, 5(1), 1–17. https://doi.org/10.1038/s41392-020-0134-x [Google Scholar] [PubMed] [CrossRef]
2. More, M. P., Pardeshi, S. R., Pardeshi, C. V., Sonawane, G. A., Shinde, M. N. et al. (2021). Recent advances in phytochemical-based nano-formulation for drug-resistant cancer. Medicine in Drug Discovery, 10(45), 100082. https://doi.org/10.1016/j.medidd.2021.100082 [Google Scholar] [CrossRef]
3. Ashrafizadeh, M., Zarrabi, A., Hashemi, F., Zabolian, A., Saleki, H. et al. (2020). Polychemotherapy with curcumin and doxorubicin via biological nanoplatforms: Enhancing antitumor activity. Pharmaceutics, 12(11), 1084. https://doi.org/10.3390/pharmaceutics12111084 [Google Scholar] [PubMed] [CrossRef]
4. Agarwal, N., Majee, C., Chakraborthy, G. (2012). Natural herbs as anticancer drugs. International Journal of PharmTech Research, 4(3), 1142–1153. [Google Scholar]
5. Bisht, D., Kumar, D., Kumar, D., Dua, K., Chellappan, D. K. (2021). Phytochemistry and pharmacological activity of the genus artemisia. Archives of Pharmacal Research, 44(5), 439–474. https://doi.org/10.1007/s12272-021-01328-4 [Google Scholar] [PubMed] [CrossRef]
6. Ashique, S., Afzal, O., Hussain, A., Zeyaullah, M., Altamimi, M. A. et al. (2023). It’s all about plant derived natural phytoconstituents and phytonanomedicine to control skin cancer. Journal of Drug Delivery Science and Technology, 84(3), 104495. https://doi.org/10.1016/j.jddst.2023.104495 [Google Scholar] [CrossRef]
7. Chavda, V. P., Patel, A. B., Mistry, K. J., Suthar, S. F., Wu, Z. -X. et al. (2022). Nano-drug delivery systems entrapping natural bioactive compounds for cancer: Recent progress and future challenges. Frontiers in Oncology, 12, 867655. https://www.frontiersin.org/articles/10.3389/fonc.2022.867655 [Google Scholar] [PubMed]
8. Devan, A. R., Nair, B., Nath, L. R. (2023). Translational phytomedicines against cancer: Promise and hurdles. Advanced Pharmaceutical Bulletin, 13(2), 210–215. https://doi.org/10.34172/apb.2023.023 [Google Scholar] [PubMed] [CrossRef]
9. International Agency for Research on Cancer. Estimated number of new cases in 2020, world, both sexes, all ages. World Health Organisation. https://gco.iarc.fr/today/online-analysis-pie?v=2020&mode=cancer&mode_population=continents&population=900&populations=900&key=total&sex=0&cancer=39&type=0&statistic=5&prevalence=0&population_group=0&ages_group%5B%5D=0&ages_group%5B%5D=17&nb_items=7&group [Google Scholar]
10. Choudhari, A. S., Mandave, P. C., Deshpande, M., Ranjekar, P., Prakash, O. (2020). Phytochemicals in cancer treatment: From preclinical studies to clinical practice. Frontiers in Pharmacology, 10, 1614. https://doi.org/10.3389/fphar.2019.01614 [Google Scholar] [PubMed] [CrossRef]
11. Shukla, S., Mehta, A. (2015). Anticancer potential of medicinal plants and their phytochemicals: A review. Brazilian Journal of Botany, 38(2), 199–210. https://doi.org/10.1007/s40415-015-0135-0 [Google Scholar] [CrossRef]
12. Abdou, P., Wang, Z., Chen, Q., Chan, A., Zhou, D. R. et al. (2020). Advances in engineering local drug delivery systems for cancer immunotherapy. WIREs Nanomedicine and Nanobiotechnology, 12(5), e1632. https://doi.org/10.1002/wnan.1632 [Google Scholar] [PubMed] [CrossRef]
13. Kulkarni, A. D., Belgamwar, V. S. (2019). Influence of novel carrier Soluplus® on aqueous stability, oral bioavailability, and anticancer activity of morin hydrate. Drying Technology, 37(9), 1143–1161. https://doi.org/10.1080/07373937.2018.1488261 [Google Scholar] [CrossRef]
14. Kulkarni, A. D., Belgamwar, V. S. (2017). Inclusion complex of chrysin with sulfobutyl ether-β-cyclodextrin (Captisol®Preparation, characterization, molecular modelling and in vitro anticancer activity. Journal of Molecular Structure, 1128, 563–571. https://doi.org/10.1016/j.molstruc.2016.09.025 [Google Scholar] [CrossRef]
15. Senapati, S., Mahanta, A. K., Kumar, S., Maiti, P. (2018). Controlled drug delivery vehicles for cancer treatment and their performance. Signal Transduction and Targeted Therapy, 3(1), 7. https://doi.org/10.1038/s41392-017-0004-3 [Google Scholar] [PubMed] [CrossRef]
16. Ranjan, A., Ramachandran, S., Gupta, N., Kaushik, I., Wright, S. et al. (2019). Role of phytochemicals in cancer prevention. International Journal of Molecular Sciences, 20(20), 1–17. https://doi.org/10.3390/ijms20204981 [Google Scholar] [PubMed] [CrossRef]
17. Joshi, B., Juyal, V., Sah, A., Verma, P., Mukhija, M. (2021). Review on documented medicinal plants used for the treatment of cancer. Current Traditional Medicine, 7(2), 100371. https://doi.org/10.2174/2215083807666211011125110 [Google Scholar] [CrossRef]
18. Chikara, S., Nagaprashantha, L. D., Singhal, J., Horne, D., Awasthi, S. et al. (2018). Oxidative stress and dietary phytochemicals: Role in cancer chemoprevention and treatment. Cancer Letters, 413(5), 122–134. https://doi.org/10.1016/j.canlet.2017.11.002 [Google Scholar] [PubMed] [CrossRef]
19. Ghobadi, N., Asoodeh, A. (2023). Co-administration of curcumin with other phytochemicals improves anticancer activity by regulating multiple molecular targets. Phytotherapy Research, 37(4), 1688–1702. https://doi.org/10.1002/ptr.7794 [Google Scholar] [PubMed] [CrossRef]
20. George, B. P., Chandran, R., Abrahamse, H. (2021). Role of phytochemicals in cancer chemoprevention: Insights. Antioxidants, 10(9), 1455. https://doi.org/10.3390/antiox10091455 [Google Scholar] [PubMed] [CrossRef]
21. Fraga, C. G., Croft, K. D., Kennedy, D. O., Tomás-Barberán, F. A. (2019). The effects of polyphenols and other bioactives on human health. Food & Function, 10(2), 514–528. https://doi.org/10.1039/C8FO01997E [Google Scholar] [PubMed] [CrossRef]
22. Russo, G. L., Tedesco, I., Spagnuolo, C., Russo, M. (2017). Antioxidant polyphenols in cancer treatment: Friend, foe or foil? Seminars in Cancer Biology, 46(Suppl. 1), 1–13. https://doi.org/10.1016/j.semcancer.2017.05.005 [Google Scholar] [PubMed] [CrossRef]
23. Luca, S. V., Macovei, I., Bujor, A., Miron, A., Skalicka-Woźniak, K. et al. (2020). Bioactivity of dietary polyphenols: The role of metabolites. Critical Reviews in Food Science and Nutrition, 60(4), 626–659. https://doi.org/10.1080/10408398.2018.1546669 [Google Scholar] [PubMed] [CrossRef]
24. Gorzynik, M., Bastian, P., Cappello, F., Kuban-Jankowska, A., Gammazza, A. et al. (2018). Potential health benefits of olive oil and plant polyphenols. International Journal of Molecular Sciences, 19(3), 686. https://doi.org/10.3390/ijms19030686 [Google Scholar] [PubMed] [CrossRef]
25. Pejčić, T., Tosti, T., Džamić, Z., Gašić, U., Vuksanović, A. et al. (2019). The polyphenols as potential agents in prevention and therapy of prostate diseases. Molecules, 24(21), 3982. https://doi.org/10.3390/molecules24213982 [Google Scholar] [PubMed] [CrossRef]
26. Miyata, Y., Shida, Y., Hakariya, T., Sakai, H. (2019). Anti-cancer effects of green tea polyphenols against prostate cancer. Molecules, 24(1), 193. https://doi.org/10.3390/molecules24010193 [Google Scholar] [PubMed] [CrossRef]
27. Costea, T., Nagy, P., Ganea, C., Szöllősi, J., Mocanu, M. M. (2019). Molecular mechanisms and bioavailability of polyphenols in prostate cancer. International Journal of Molecular Sciences, 20(5), 1062. https://doi.org/10.3390/ijms20051062 [Google Scholar] [PubMed] [CrossRef]
28. Rauf, A., Imran, M., Butt, M. S., Nadeem, M., Peters, D. G. et al. (2018). Resveratrol as an anti-cancer agent: A review. Critical Reviews in Food Science and Nutrition, 58(9), 1428–1447. https://doi.org/10.1080/10408398.2016.1263597 [Google Scholar] [PubMed] [CrossRef]
29. Lin, M. H., Hung, C. F., Sung, H. C., Yang, S. C., Yu, H. P. et al. (2021). The bioactivities of resveratrol and its naturally occurring derivatives on skin. Journal of Food and Drug Analysis, 29(1), 15–38. https://doi.org/10.38212/2224-6614.1151 [Google Scholar] [PubMed] [CrossRef]
30. Montané, X., Kowalczyk, O., Reig-Vano, B., Bajek, A., Roszkowski, K. et al. (2020). Current perspectives of the applications of polyphenols and flavonoids in cancer therapy. Molecules, 25(15), 3342. https://doi.org/10.3390/molecules25153342 [Google Scholar] [PubMed] [CrossRef]
31. Pereira, T. M. C., Carvalho, G. R., Luaces-Regueira, M., Bugallo-Casal, A., Iglesias-Mejuto, A. et al. (2022). Nanomolar resveratrol reduces early alterations of pancreatitis and pancreatic cancer in pancreatic acinar cells. Phytomedicine Plus, 2(3), 100301. https://doi.org/10.1016/j.phyplu.2022.100301 [Google Scholar] [CrossRef]
32. Zhang, L., Lin, Z., Chen, Y., Gao, D., Wang, P. et al. (2022). Co-delivery of docetaxel and resveratrol by liposomes synergistically boosts antitumor efficiency against prostate cancer. European Journal of Pharmaceutical Sciences, 174, 106199. https://doi.org/10.1016/j.ejps.2022.106199 [Google Scholar] [PubMed] [CrossRef]
33. Giordano, A., Tommonaro, G. (2019). Curcumin and cancer. Nutrients, 11(10), 2376. https://doi.org/10.3390/nu11102376 [Google Scholar] [PubMed] [CrossRef]
34. Mortezaee, K., Salehi, E., Mirtavoos-Mahyari, H., Motevaseli, E., Najafi, M. et al. (2019). Mechanisms of apoptosis modulation by curcumin: Implications for cancer therapy. Journal of Cellular Physiology, 234(8), 12537–12550. https://doi.org/10.1002/jcp.28122 [Google Scholar] [PubMed] [CrossRef]
35. Kumari, A., Raina, N., Wahi, A., Goh, K. W., Sharma, P. et al. (2022). Wound-healing effects of curcumin and its nanoformulations: A comprehensive review. Pharmaceutics, 14(11), 2288. https://doi.org/10.3390/pharmaceutics14112288 [Google Scholar] [PubMed] [CrossRef]
36. de Souza Ferreira, S. B., Bruschi, M. L. (2019). Improving the bioavailability of curcumin: Is micro/nanoencapsulation the key? Therapeutic Delivery, 10(2), 83–86. https://doi.org/10.4155/tde-2018-0075 [Google Scholar] [PubMed] [CrossRef]
37. Rudzińska, A., Juchaniuk, P., Oberda, J., Wiśniewska, J., Wojdan, W. et al. (2023). Phytochemicals in cancer treatment and cancer prevention-review on epidemiological data and clinical trials. Nutrients, 15(8), 1896. https://doi.org/10.3390/nu15081896 [Google Scholar] [PubMed] [CrossRef]
38. Bhia, M., Motallebi, M., Abadi, B., Zarepour, A., Pereira-Silva, M. et al. (2021). Naringenin nano-delivery systems and their therapeutic applications. Pharmaceutics, 13(2), 291. https://doi.org/10.3390/pharmaceutics13020291 [Google Scholar] [PubMed] [CrossRef]
39. Akhter, M. H., Kumar, S., Nomani, S. (2020). Sonication tailored enhance cytotoxicity of naringenin nanoparticle in pancreatic cancer: Design, optimization, and in vitro studies. Drug Development and Industrial Pharmacy, 46(4), 659–672. https://doi.org/10.1080/03639045.2020.1747485 [Google Scholar] [PubMed] [CrossRef]
40. Yıldırım, M., Acet, Ö., Yetkin, D., Acet, B. Ö., Karakoc, V. et al. (2022). Anti-cancer activity of naringenin loaded smart polymeric nanoparticles in breast cancer. Journal of Drug Delivery Science and Technology, 74(24), 103552. https://doi.org/10.1016/j.jddst.2022.103552 [Google Scholar] [CrossRef]
41. Tang, S. M., Deng, X. T., Zhou, J., Li, Q. P., Ge, X. X. et al. (2020). Pharmacological basis and new insights of quercetin action in respect to its anti-cancer effects. Biomedicine & Pharmacotherapy, 121, 109604. https://doi.org/10.1016/j.biopha.2019.109604 [Google Scholar] [PubMed] [CrossRef]
42. Lakshmi, B. A., Kim, S. (2019). Quercetin mediated gold nanoclusters explored as a dual functional nanomaterial in anticancer and bio-imaging disciplines. Colloids and Surfaces B: Biointerfaces, 178, 230–237. https://doi.org/10.1016/j.colsurfb.2019.02.054 [Google Scholar] [PubMed] [CrossRef]
43. Elsayed, A. M., Sherif, N. M., Hassan, N. S., Althobaiti, F., Hanafy, N. A. N. et al. (2021). Novel quercetin encapsulated chitosan functionalized copper oxide nanoparticles as anti-breast cancer agent via regulating p53 in rat model. International Journal of Biological Macromolecules, 185(7), 134–152. https://doi.org/10.1016/j.ijbiomac.2021.06.085 [Google Scholar] [PubMed] [CrossRef]
44. Imran, M., Salehi, B., Sharifi-Rad, J., Aslam Gondal, T., Saeed, F. et al. (2019). Kaempferol: A key emphasis to its anticancer potential. Molecules, 24(12), 2277. https://doi.org/10.3390/molecules24122277 [Google Scholar] [PubMed] [CrossRef]
45. Govindaraju, S., Roshini, A., Lee, M. H., Yun, K. (2019). Kaempferol conjugated gold nanoclusters enabled efficient for anticancer therapeutics to A549 lung cancer cells. International Journal of Nanomedicine, 14, 5147–5157. https://doi.org/10.2147/IJN [Google Scholar] [CrossRef]
46. Hassan, F., Rehman, M. S., Khan, M. S., Ali, M. A., Javed, A. et al. (2019). Curcumin as an alternative epigenetic modulator: Mechanism of action and potential effects. Frontiers in Genetics, 10, 514. https://doi.org/10.3389/fgene.2019.00514 [Google Scholar] [PubMed] [CrossRef]
47. Aghazadeh, T., Bakhtiari, N., Rad, I. A., Ramezani, F. (2021). Formulation of kaempferol in nanostructured lipid carriers (NLCsA delivery platform to sensitization of MDA-MB468 breast cancer cells to paclitaxel. Biointerface Research in Applied Chemistry, 11(6), 14591–14601. https://doi.org/10.33263/BRIAC116.1459114601 [Google Scholar] [CrossRef]
48. Imran, M., Saeed, F., Hussain, G., Imran, A., Mehmood, Z. et al. (2021). Myricetin: A comprehensive review on its biological potentials. Food Science & Nutrition, 9(10), 5854–5868. https://doi.org/10.1002/fsn3.2513 [Google Scholar] [PubMed] [CrossRef]
49. Alidadi, H., Ashtari, A., Samimi, A., Karami, M. A., Khorsandi, L. (2022). Myricetin loaded in solid lipid nanoparticles induces apoptosis in the HT-29 colorectal cancer cells via mitochondrial dysfunction. Molecular Biology Reports, 49(9), 8537–8545. https://doi.org/10.1007/s11033-022-07683-9 [Google Scholar] [PubMed] [CrossRef]
50. Satari, A., Ghasemi, S., Habtemariam, S., Asgharian, S., Lorigooini, Z. (2021). Rutin: A flavonoid as an effective sensitizer for anticancer therapy; Insights into multifaceted mechanisms and applicability for combination therapy. Evidence-Based Complementary and Alternative Medicine, 2021(1), 9913179. https://doi.org/10.1155/2021/9913179 [Google Scholar] [PubMed] [CrossRef]
51. Saleemi, M. A., Alallam, B., Yong, Y. K., Lim, V. (2022). Synthesis of zinc oxide nanoparticles with bioflavonoid rutin: Characterisation, antioxidant and antimicrobial activities and in vivo cytotoxic effects on Artemia nauplii. Antioxidants, 11(10), 1853. https://doi.org/10.3390/antiox11101853 [Google Scholar] [PubMed] [CrossRef]
52. Bohlouli, S., Jafarmadar Gharehbagh, F., Dalir Abdolahinia, E., Kouhsoltani, M., Ebrahimi, G. et al. (2021). Preparation, characterization, and evaluation of rutin nanocrystals as an anticancer agent against head and neck squamous cell carcinoma cell line. Journal of Nanomaterials, 2021(3), 9980451. https://doi.org/10.1155/2021/9980451 [Google Scholar] [CrossRef]
53. Prakash, M., Basavaraj, B. V., Chidambara Murthy, K. N. (2019). Biological functions of epicatechin: Plant cell to human cell health. Journal of Functional Foods, 52(4), 14–24. https://doi.org/10.1016/j.jff.2018.10.021 [Google Scholar] [CrossRef]
54. Perez-Ruiz, A. G., Ganem, A., Olivares-Corichi, I. M., García-Sánchez, J. R. (2018). Lecithin-chitosan–TPGS nanoparticles as nanocarriers of (−)-epicatechin enhanced its anticancer activity in breast cancer cells. RSC Advances, 8(61), 34773–34782. https://doi.org/10.1039/C8RA06327C [Google Scholar] [PubMed] [CrossRef]
55. Abotaleb, M., Liskova, A., Kubatka, P., Büsselberg, D. (2020). Therapeutic potential of plant phenolic acids in the treatment of cancer. Biomolecules, 10(2), 221. https://doi.org/10.3390/biom10020221 [Google Scholar] [PubMed] [CrossRef]
56. Wahle, K. W. J., Brown, I., Rotondo, D., Heys, S. D. (2010). Plant phenolics in the prevention and treatment of cancer BT—bio-farms for nutraceuticals: Functional food and safety control by biosensors. In: Giardi, M. T., Rea, G., Berra, B. (Eds.Bio-farms for nutraceuticals, pp. 36–51. Boston, MA, USA: Springer. https://doi.org/10.1007/978-1-4419-7347-4_4 [Google Scholar] [CrossRef]
57. Dey, P., Kundu, A., Chakraborty, H. J., Kar, B., Choi, W. S. et al. (2019). Therapeutic value of steroidal alkaloids in cancer: Current trends and future perspectives. International Journal of Cancer, 145(7), 1731–1744. https://doi.org/10.1002/ijc.31965 [Google Scholar] [PubMed] [CrossRef]
58. Liu, C., Yang, S., Wang, K., Bao, X., Liu, Y. et al. (2019). Alkaloids from traditional Chinese medicine against hepatocellular carcinoma. Biomedicine & Pharmacotherapy, 120(2), 109543. https://doi.org/10.1016/j.biopha.2019.109543 [Google Scholar] [PubMed] [CrossRef]
59. Shabgah, A. G., Suksatan, W., Achmad, M. H., Bokov, D. O., Abdelbasset, W. K. et al. (2021). Arctigenin, an anti-tumor agent; A cutting-edge topic and up-to-the-minute approach in cancer treatment. European Journal of Pharmacology, 909, 174419. https://doi.org/10.1016/j.ejphar.2021.174419 [Google Scholar] [PubMed] [CrossRef]
60. Mondal, A., Gandhi, A., Fimognari, C., Atanasov, A. G., Bishayee, A. (2019). Alkaloids for cancer prevention and therapy: Current progress and future perspectives. European Journal of Pharmacology, 858(1–12), 172472. https://doi.org/10.1016/j.ejphar.2019.172472 [Google Scholar] [PubMed] [CrossRef]
61. Wang, K., Gu, C., Yu, G., Lin, J., Wang, Z. et al. (2022). Berberine enhances the anti-hepatocellular carcinoma effect of NK92-MI cells through inhibiting IFN-gamma-mediated PD-L1 expression. Liver Research, 6(3), 167–174. https://doi.org/10.1016/j.livres.2022.08.003 [Google Scholar] [CrossRef]
62. Omran, Z., Guise, C. P., Chen, L., Rauch, C., Abdalla, A. N. et al. (2021). Design, synthesis and in vitro biological evaluation of antofine and tylophorine prodrugs as hypoxia-targeted anticancer agents. Molecules, 26(11), 3327. https://doi.org/10.3390/molecules26113327 [Google Scholar] [PubMed] [CrossRef]
63. Jiang, Q. W., Chen, M. W., Cheng, K. J., Yu, P. Z., Wei, X. et al. (2016). Therapeutic potential of steroidal alkaloids in cancer and other diseases. Medicinal Research Reviews, 36(1), 119–143. https://doi.org/10.1002/med.21346 [Google Scholar] [PubMed] [CrossRef]
64. El-Baba, C., Baassiri, A., Kiriako, G., Dia, B., Fadlallah, S. et al. (2021). Terpenoids’ anti-cancer effects: Focus on autophagy. Apoptosis, 26(9), 491–511. https://doi.org/10.1007/s10495-021-01684-y [Google Scholar] [PubMed] [CrossRef]
65. Huang, M., Lu, J. J., Huang, M. Q., Bao, J. L., Chen, X. P. et al. (2012). Terpenoids: Natural products for cancer therapy. Expert Opinion on Investigational Drugs, 21(12), 1801–1818. https://doi.org/10.1517/13543784.2012.727395 [Google Scholar] [PubMed] [CrossRef]
66. Chang, H. T., Hsu, S. S., Chou, C. T., Cheng, J. S., Wang, J. L. et al. (2011). Effect of thymol on Ca2+ homeostasis and viability in MG63 human osteosarcoma cells. Pharmacology, 88(3–4), 201–212. https://doi.org/10.1159/000331864 [Google Scholar] [PubMed] [CrossRef]
67. Deb, D. D., Parimala, G., Devi, S. S., Chakraborty, T. (2011). Effect of thymol on peripheral blood mononuclear cell PBMC and acute promyelotic cancer cell line HL-60. Chemico-Biological Interactions, 193(1), 97–106. https://doi.org/10.1016/j.cbi.2011.05.009 [Google Scholar] [PubMed] [CrossRef]
68. Ishfaq, M. P., Shukla, A., Beraiya, S., Tripathi, S., Mishra, K. S. (2018). Biochemical and pharmacological applications of essential oils in human health especially in cancer prevention. Anti-Cancer Agents in Medicinal Chemistry, 18(13), 1815–1827. https://doi.org/10.2174/1871520618666181002130240 [Google Scholar] [PubMed] [CrossRef]
69. Blowman, K., Magalhães, M., Lemos, M. F. L., Cabral, C., Pires, I. M. (2018). Anticancer properties of essential oils and other natural products. Evidence-Based Complementary and Alternative Medicine, 2018(7), 3149362. https://doi.org/10.1155/2018/3149362 [Google Scholar] [PubMed] [CrossRef]
70. Bakrania, A. K., Variya, B. C., Patel, S. S. (2016). Novel targets for paclitaxel nano formulations: Hopes and hypes in triple negative breast cancer. Pharmacological Research, 111(12), 577–591. https://doi.org/10.1016/j.phrs.2016.07.023 [Google Scholar] [PubMed] [CrossRef]
71. Mutlu-Agardan, N. B., Sarisozen, C., Torchilin, V. P. (2020). Cytotoxicity of novel redox sensitive PEG(2000)-S-S-PTX micelles against drug-resistant ovarian and breast cancer cells. Pharmaceutical Research, 37(3), 65. https://doi.org/10.1007/s11095-020-2759-4 [Google Scholar] [PubMed] [CrossRef]
72. Rajivgandhi, G., Saravanan, K., Ramachandran, G., Li, J. L., Yin, L. et al. (2020). Enhanced anti-cancer activity of chitosan loaded Morinda citrifolia essential oil against A549 human lung cancer cells. International Journal of Biological Macromolecules, 164(226), 4010–4021. https://doi.org/10.1016/j.ijbiomac.2020.08.169 [Google Scholar] [PubMed] [CrossRef]
73. Najjari, N., Sari, S., Saffari, M., Kelidari, H., Nokhodchi, A. (2022). Formulation optimization and characterization of Pistacia atlantica Desf. essential oil-loaded nanostructured lipid carriers on the proliferation of human breast cancer cell line SKBR3 (in vitro studies). Journal of Herbal Medicine, 36(2), 100600. https://doi.org/10.1016/j.hermed.2022.100600 [Google Scholar] [CrossRef]
74. Ballout, F., Habli, Z., Rahal, O. N., Fatfat, M., Gali-Muhtasib, H. (2018). Thymoquinone-based nanotechnology for cancer therapy: Promises and challenges. Drug Discovery Today, 23(5), 1089–1098. https://doi.org/10.1016/j.drudis.2018.01.043 [Google Scholar] [PubMed] [CrossRef]
75. Gaspani, S., Milani, B. (2013). Access to liposomal generic formulations: Beyond AmBisome and Doxil/Caelyx. Generics and Biosimilars Initiative Journal, 2(2), 60–62. https://doi.org/10.5639/gabij.2013.0202.022 [Google Scholar] [CrossRef]
76. Swenson, C. E., Haemmerich, D., Maul, D. H., Knox, B., Ehrhart, N. et al. (2015). Increased duration of heating boosts local drug deposition during radiofrequency ablation in combination with thermally sensitive liposomes (ThermoDox) in a porcine model. PLoS One, 10(10), e0139752. https://doi.org/10.1371/journal.pone.0139752 [Google Scholar] [PubMed] [CrossRef]
77. Ke, W., Zha, Z., Mukerabigwi, J. F., Chen, W., Wang, Y. et al. (2017). Matrix metalloproteinase-responsive multifunctional peptide-linked amphiphilic block copolymers for intelligent systemic anticancer drug delivery. Bioconjugate Chemistry, 28(8), 2190–2198. https://doi.org/10.1021/acs.bioconjchem.7b00330 [Google Scholar] [PubMed] [CrossRef]
78. Rautray, S., Panikar, S., Amutha, T., Rajananthini, A. U. (2018). Anticancer activity of Adiantum capillus veneris and Pteris quadriureta L. in human breast cancer cell lines. Molecular Biology Reports, 45(6), 1897–1911. https://doi.org/10.1007/s11033-018-4337-y [Google Scholar] [PubMed] [CrossRef]
79. Vijayakumar, S., Malaikozhundan, B., Saravanakumar, K., Durán-Lara, E. F., Wang, M. H. et al. (2019). Garlic clove extract assisted silver nanoparticle—antibacterial, antibiofilm, antihelminthic, anti-inflammatory, anticancer and ecotoxicity assessment. Journal of Photochemistry and Photobiology B: Biology, 198, 111558. https://doi.org/10.1016/j.jphotobiol.2019.111558 [Google Scholar] [PubMed] [CrossRef]
80. Qian, L., Su, W., Wang, Y., Dang, M., Zhang, W. et al. (2019). Synthesis and characterization of gold nanoparticles from aqueous leaf extract of Alternanthera sessilis and its anticancer activity on cervical cancer cells (HeLa). Artificial Cells, Nanomedicine, and Biotechnology, 47(1), 1173–1180. https://doi.org/10.1080/21691401.2018.1549064 [Google Scholar] [PubMed] [CrossRef]
81. Baghbani-Arani, F., Movagharnia, R., Sharifian, A., Salehi, S., Shandiz, S. A. S. (2017). Photo-catalytic, anti-bacterial, and anti-cancer properties of phyto-mediated synthesis of silver nanoparticles from Artemisia tournefortiana Rchb extract. Journal of Photochemistry and Photobiology B: Biology, 173, 640–649. https://doi.org/10.1016/j.jphotobiol.2017.07.003 [Google Scholar] [PubMed] [CrossRef]
82. Rajput, S., Kumar, D., Agrawal, V. (2020). Green synthesis of silver nanoparticles using Indian Belladonna extract and their potential antioxidant, anti-inflammatory, anticancer and larvicidal activities. Plant Cell Reports, 39(7), 921–939. https://doi.org/10.1007/s00299-020-02539-7 [Google Scholar] [PubMed] [CrossRef]
83. Vijayan, R., Joseph, S., Mathew, B. (2019). Anticancer, antimicrobial, antioxidant, and catalytic activities of green-synthesized silver and gold nanoparticles using Bauhinia purpurea leaf extract. Bioprocess and Biosystems Engineering, 42(2), 305–319. https://doi.org/10.1007/s00449-018-2035-8 [Google Scholar] [PubMed] [CrossRef]
84. Singh, H., Du, J., Yi, T. H. (2017). Green and rapid synthesis of silver nanoparticles using Borago officinalis leaf extract: Anticancer and antibacterial activities. Artificial Cells, Nanomedicine, and Biotechnology, 45(7), 1310–1316. https://doi.org/10.1080/21691401.2016.1228663 [Google Scholar] [PubMed] [CrossRef]
85. Yadav, A., Mendhulkar, V. D. (2018). Antiproliferative activity of Camellia sinensis mediated silver nanoparticles on three different human cancer cell lines. Journal of Cancer Research and Therapeutics, 14(6), 1316–1324. https://doi.org/10.4103/jcrt.JCRT_575_16 [Google Scholar] [PubMed] [CrossRef]
86. Azhar, N. A., Ghozali, S. Z., Abu Bakar, S. A., Lim, V., Ahmad, N. H. (2020). Suppressing growth, migration, and invasion of human hepatocellular carcinoma HepG2 cells by Catharanthus roseus‐silver nanoparticles. Toxicology In Vitro: An International Journal Published in Association with BIBRA, 67(2), 104910. https://doi.org/10.1016/j.tiv.2020.104910 [Google Scholar] [PubMed] [CrossRef]
87. Li, F., Song, L., Yang, X., Huang, Z., Mou, X. et al. (2020). Anticancer and genotoxicity effect of (Clausena lansium (Lour.) Skeels) Peel ZnONPs on neuroblastoma (SH-SY5Y) cells through the modulation of autophagy mechanism. Journal of Photochemistry and Photobiology B: Biology, 203(9), 111748. https://doi.org/10.1016/j.jphotobiol.2019.111748 [Google Scholar] [PubMed] [CrossRef]
88. Liu, R., Pei, Q., Shou, T., Zhang, W., Hu, J. et al. (2019). Apoptotic effect of green synthesized gold nanoparticles from Curcuma wenyujin extract against human renal cell carcinoma A498 cells. International Journal of Nanomedicine, 14, 4091–4103. https://doi.org/10.2147/IJN.S203222 [Google Scholar] [PubMed] [CrossRef]
89. Erdogan, O., Abbak, M., Demirbolat, G. M., Birtekocak, F., Aksel, M. et al. (2019). Green synthesis of silver nanoparticles via Cynara scolymus leaf extracts: The characterization, anticancer potential with photodynamic therapy in MCF7 cells. PLoS One, 14(6), e0216496. https://doi.org/10.1371/journal.pone.0216496 [Google Scholar] [PubMed] [CrossRef]
90. Selim, Y. A., Azb, M. A., Ragab, I., El-Azim, H. M., Abd El-Azim, M. (2020). Green synthesis of zinc oxide nanoparticles using aqueous extract of Deverra tortuosa and their cytotoxic activities. Scientific Reports, 10(1), 3445. https://doi.org/10.1038/s41598-020-60541-1 [Google Scholar] [PubMed] [CrossRef]
91. Mohanta, Y. K., Panda, S. K., Jayabalan, R., Sharma, N., Bastia, A. K. et al. (2017). Antimicrobial, antioxidant and cytotoxic activity of silver nanoparticles synthesized by leaf extract of Erythrina suberosa (Roxb.). Frontiers in Molecular Biosciences, 4, 14. https://doi.org/10.3389/fmolb.2017.00014 [Google Scholar] [PubMed] [CrossRef]
92. Kanjikar, A. P., Hugar, A. L., Londonkar, R. L. (2018). Characterization of phyto-nanoparticles from Ficus krishnae for their antibacterial and anticancer activities. Drug Development and Industrial Pharmacy, 44(3), 377–384. https://doi.org/10.1080/03639045.2017.1386205 [Google Scholar] [PubMed] [CrossRef]
93. Lu, L., Zhuang, Z., Fan, M., Liu, B., Yang, Y. et al. (2022). Green formulation of Ag nanoparticles by Hibiscus rosa-sinensis: Introducing a navel chemotherapeutic drug for the treatment of liver cancer. Arabian Journal of Chemistry, 15(2), 103602. https://doi.org/10.1016/j.arabjc.2021.103602 [Google Scholar] [CrossRef]
94. Ramkumar, R., Balasubramani, G., Raja, R. K., Raja, M., Govindan, R. et al. (2017). Lantana camara Linn root extract-mediated gold nanoparticles and their in vitro antioxidant and cytotoxic potentials. Artificial Cells, Nanomedicine, and Biotechnology, 45(4), 748–757. https://doi.org/10.1080/21691401.2016.1276923 [Google Scholar] [PubMed] [CrossRef]
95. Iqbal, M. J., Ali, S., Rashid, U., Kamran, M., Malik, M. F. et al. (2018). Biosynthesis of silver nanoparticles from leaf extract of Litchi chinensis and its dynamic biological impact on microbial cells and human cancer cell lines. Cellular and Molecular Biology, 64(13), 42–47. https://doi.org/10.14715/cmb/2018.64.13.9 [Google Scholar] [CrossRef]
96. Vimalraj, S., Ashokkumar, T., Saravanan, S. (2018). Biogenic gold nanoparticles synthesis mediated by Mangifera indica seed aqueous extracts exhibits antibacterial, anticancer and anti-angiogenic properties. Biomedicine & Pharmacotherapy, 105(2), 440–448. https://doi.org/10.1016/j.biopha.2018.05.151 [Google Scholar] [PubMed] [CrossRef]
97. Li, L., Zhang, W., Desikan Seshadri, V. D., Cao, G. (2019). Synthesis and characterization of gold nanoparticles from Marsdenia tenacissima and its anticancer activity of liver cancer HepG2 cells. Artificial Cells, Nanomedicine, and Biotechnology, 47(1), 3029–3036. https://doi.org/10.1080/21691401.2019.1642902 [Google Scholar] [PubMed] [CrossRef]
98. Ali, H., Al-Khalifa, A. R., Aouf, A., Boukhebti, H., Farouk, A. (2020). Effect of nanoencapsulation on volatile constituents, and antioxidant and anticancer activities of Algerian Origanum glandulosum Desf. essential oil. Scientific Reports, 10(1), 2812. https://doi.org/10.1038/s41598-020-59686-w [Google Scholar] [PubMed] [CrossRef]
99. Oves, M., Aslam, M., Rauf, M. A., Qayyum, S., Qari, H. A. et al. (2018). Antimicrobial and anticancer activities of silver nanoparticles synthesized from the root hair extract of Phoenix dactylifera. Materials Science and Engineering: C, 89(4), 429–443. https://doi.org/10.1016/j.msec.2018.03.035 [Google Scholar] [PubMed] [CrossRef]
100. Hajebi, S., Tabrizi, M. H., Moghaddam, M. N., Shahraki, F., Yadamani, S. (2019). Rapeseed flower pollen bio-green synthesized silver nanoparticles: A promising antioxidant, anticancer and antiangiogenic compound. JBIC Journal of Biological Inorganic Chemistry, 24(3), 395–404. https://doi.org/10.1007/s00775-019-01655-4 [Google Scholar] [PubMed] [CrossRef]
101. Iqbal, J., Abbasi, B. A., Ahmad, R., Mahmoodi, M., Munir, A. et al. (2020). Phytogenic synthesis of nickel oxide nanoparticles (NiO) using fresh leaves extract of Rhamnus triquetra (Wall.) and investigation of its multiple in vitro biological potentials. Biomedicines, 8(5), 117. https://doi.org/10.3390/biomedicines8050117 [Google Scholar] [PubMed] [CrossRef]
102. Sisubalan, N., Ramkumar, V. S., Pugazhendhi, A., Karthikeyan, C., Indira, K. et al. (2018). ROS-mediated cytotoxic activity of ZnO and CeO2 nanoparticles synthesized using the Rubia cordifolia L. leaf extract on MG-63 human osteosarcoma cell lines. Environmental Science and Pollution Research, 25(11), 10482–10492. https://doi.org/10.1007/s11356-017-0003-5 [Google Scholar] [PubMed] [CrossRef]
103. Zhang, K., Liu, X., Samuel Ravi, S. O. A., Ramachandran, A., Aziz Ibrahim, I. A. et al. (2019). Synthesis of silver nanoparticles (AgNPs) from leaf extract of Salvia miltiorrhiza and its anticancer potential in human prostate cancer LNCaP cell lines. Artificial Cells, Nanomedicine, and Biotechnology, 47(1), 2846–2854. https://doi.org/10.1080/21691401.2019.1638792 [Google Scholar] [PubMed] [CrossRef]
104. Wu, F., Zhu, J., Li, G., Wang, J., Veeraraghavan, V. P. et al. (2019). Biologically synthesized green gold nanoparticles from Siberian ginseng induce growth-inhibitory effect on melanoma cells (B16). Artificial Cells, Nanomedicine, and Biotechnology, 47(1), 3297–3305. https://doi.org/10.1080/21691401.2019.1647224 [Google Scholar] [PubMed] [CrossRef]
105. Zhang, P., Wang, P., Yan, L., Liu, L. (2018). Synthesis of gold nanoparticles with Solanum xanthocarpum extract and their in vitro anticancer potential on nasopharyngeal carcinoma cells. International Journal of Nanomedicine, 13, 7047–7059. https://doi.org/10.2147/IJN.S180138 [Google Scholar] [PubMed] [CrossRef]
106. Yun, Z., Chinnathambi, A., Alharbi, S. A., Jin, Z. (2020). Biosynthesis of gold nanoparticles using Vetex negundo and evaluation of pro-apoptotic effect on human gastric cancer cell lines. Journal of Photochemistry and Photobiology B: Biology, 203, 111749. https://doi.org/10.1016/j.jphotobiol.2019.111749 [Google Scholar] [PubMed] [CrossRef]
107. Baharara, J., Ramezani, T., Hosseini, N., Mousavi, M. (2018). Silver nanoparticles synthesized coating with Zataria multiflora leaves extract induced apoptosis in HeLa cells through p53 activation. Iranian Journal of Pharmaceutical Research, 17(2), 627–639, https://pubmed.ncbi.nlm.nih.gov/29881420 [Google Scholar] [PubMed]
108. Cao, Y., Xie, Y., Liu, L., Xiao, A., Li, Y. et al. (2017). Influence of phytochemicals on the biocompatibility of inorganic nanoparticles: A state-of-the-art review. Phytochemistry Reviews, 16(3), 555–563. https://doi.org/10.1007/s11101-017-9490-8 [Google Scholar] [CrossRef]
109. Khaiwa, N., Maarouf, N. R., Darwish, M. H., Alhamad, D. W. M., Sebastian, A. et al. (2021). Camptothecin’s journey from discovery to WHO essential medicine: Fifty years of promise. European Journal of Medicinal Chemistry, 223(Suppl 3), 113639. https://doi.org/10.1016/j.ejmech.2021.113639 [Google Scholar] [PubMed] [CrossRef]
110. Alharbi, W. S., Almughem, F. A., Almehmady, A. M., Jarallah, S. J., Alsharif, W. K. et al. (2021). Phytosomes as an emerging nanotechnology platform for the topical delivery of bioactive phytochemicals. Pharmaceutics, 13(9), 1475. https://doi.org/10.3390/pharmaceutics13091475 [Google Scholar] [PubMed] [CrossRef]
111. Rao, P. V., Nallappan, D., Madhavi, K., Rahman, S., Jun Wei, L. et al. (2016). Phytochemicals and biogenic metallic nanoparticles as anticancer agents. Oxidative Medicine and Cellular Longevity, 2016(5), 3685671. https://doi.org/10.1155/2016/3685671 [Google Scholar] [PubMed] [CrossRef]
112. Pushparaj, K., Balasubramanian, B., Kandasamy, Y., Arumugam, V. A., Kaliannan, D. et al. (2023). Green synthesis, characterization of silver nanoparticles using aqueous leaf extracts of Solanum melongena and in vitro evaluation of antibacterial, pesticidal and anticancer activity in human MDA-MB-231 breast cancer cell lines. Journal of King Saud University-Science, 35(5), 102663. https://doi.org/10.1016/j.jksus.2023.102663 [Google Scholar] [CrossRef]
113. Sajid, M., Cameotra, S. S., Ahmad Khan, M. S., Ahmad, I. (2019). Chapter 23-nanoparticle-based delivery of phytomedicines: Challenges and opportunities. In: Ahmad Khan, M. S., Ahmad, I., Chattopadhyay, D. (Eds.New look to phytomedicine, pp. 597–623. Academic Press. https://doi.org/10.1016/B978-0-12-814619-4.00024-0 [Google Scholar] [CrossRef]
114. Shu, Q., Wu, J., Chen, Q. (2019). Synthesis, characterization of liposomes modified with biosurfactant MEL—A loading betulinic acid and its anticancer effect in HepG2 cell. Molecules, 24(21), 3939. https://doi.org/10.3390/molecules24213939 [Google Scholar] [PubMed] [CrossRef]
115. Karimi, M., Gheybi, F., Zamani, P., Mashreghi, M., Golmohammadzadeh, S. et al. (2020). Preparation and characterization of stable nanoliposomal formulations of curcumin with high loading efficacy: In vitro and in vivo anti-tumor study. International Journal of Pharmaceutics, 580, 119211. [Google Scholar] [PubMed]
116. Greil, R., Greil-Ressler, S., Weiss, L., Schönlieb, C., Magnes, T. et al. (2018). A phase 1 dose-escalation study on the safety, tolerability and activity of liposomal curcumin (Lipocurc(TM)) in patients with locally advanced or metastatic cancer. Cancer Chemotherapy and Pharmacology, 82(4), 695–706. https://doi.org/10.1007/s00280-018-3654-0 [Google Scholar] [PubMed] [CrossRef]
117. Bolger, G. T., Licollari, A., Tan, A., Greil, R., Vcelar, B. et al. (2019). Pharmacokinetics of liposomal curcumin (LipocurcTM) infusion: Effect of co-medication in cancer patients and comparison with healthy individuals. Cancer Chemotherapy and Pharmacology, 83(2), 265–275. https://doi.org/10.1007/s00280-018-3730-5 [Google Scholar] [PubMed] [CrossRef]
118. AbouSamra, M. M., Afifi, S. M., Galal, A. F., Kamel, R. (2023). Rutin-loaded phyto-sterosomes as a potential approach for the treatment of hepatocellular carcinoma: In vitro and in vivo studies. Journal of Drug Delivery Science and Technology, 79, 104015. https://doi.org/10.1016/j.jddst.2022.104015 [Google Scholar] [CrossRef]
119. Khoee, S., Yaghoobian, M. (2017). Chapter 6-niosomes: A novel approach in modern drug delivery systems. In: Andronescu, E., Grumezescu, A. M. (Eds.Micro and nano technologies, pp. 207–237. Elsevier. https://doi.org/10.1016/B978-0-323-46143-6.00006-3 [Google Scholar] [CrossRef]
120. Zare-Zardini, H., Alemi, A., Taheri-Kafrani, A., Hosseini, S. A., Soltaninejad, H. et al. (2020). Assessment of a new ginsenoside Rh2 nanoniosomal formulation for enhanced antitumor efficacy on prostate cancer: An in vitro study. Drug Design, Development and Therapy, 14, 3315–3324. https://doi.org/10.2147/DDDT.S261027 [Google Scholar] [PubMed] [CrossRef]
121. Barani, M., Mirzaei, M., Torkzadeh-Mahani, M., Adeli-Sardou, M. (2019). Evaluation of carum-loaded niosomes on breast cancer cells: Physicochemical properties, in vitro cytotoxicity, flow cytometric, DNA fragmentation and cell migration assay. Scientific Reports, 9(1), 7139. https://doi.org/10.1038/s41598-019-43755-w [Google Scholar] [PubMed] [CrossRef]
122. Verma, P., Pathak, K. (2010). Therapeutic and cosmeceutical potential of ethosomes: An overview. Journal of Advanced Pharmaceutical Technology & Research, 1(3), 274–282. https://doi.org/10.4103/0110-5558.72415 [Google Scholar] [PubMed] [CrossRef]
123. Nasr, S., Rady, M., Gomaa, I., Syrovets, T., Simmet, T. et al. (2019). Ethosomes and lipid-coated chitosan nanocarriers for skin delivery of a chlorophyll derivative: A potential treatment of squamous cell carcinoma by photodynamic therapy. International Journal of Pharmaceutics, 568(5), 118528. https://doi.org/10.1016/j.ijpharm.2019.118528 [Google Scholar] [PubMed] [CrossRef]
124. Hua, S. (2015). Lipid-based nano-delivery systems for skin delivery of drugs and bioactives. Frontiers in Pharmacology. https://www.frontiersin.org/articles/10.3389/fphar.2015.00219 [Google Scholar]
125. Moolakkadath, T., Aqil, M., Ahad, A., Imam, S. S., Praveen, A. et al. (2019). Fisetin loaded binary ethosomes for management of skin cancer by dermal application on UV exposed mice. International Journal of Pharmaceutics, 560, 78–91. https://doi.org/10.1016/j.ijpharm.2019.01.067 [Google Scholar] [PubMed] [CrossRef]
126. Garg, V., Singh, H., Bimbrawh, S., Singh, S. K., Gulati, M. et al. (2017). Ethosomes and transfersomes: Principles, perspectives and practices. Current Drug Delivery, 14(5), 613–633. https://doi.org/10.2174/1567201813666160520114436 [Google Scholar] [PubMed] [CrossRef]
127. Eskolaky, E. B., Ardjmand, M., Akbarzadeh, A. (2015). Evaluation of anti-cancer properties of pegylated ethosomal paclitaxel on human melanoma cell line SKMEL-3. Tropical Journal of Pharmaceutical Research, 14(8), 1421–1425. https://doi.org/10.4314/tjpr.v14i8.14 [Google Scholar] [CrossRef]
128. Lin, H., Lin, L., Choi, Y., Michniak-Kohn, B. (2020). Development and in vitro evaluation of co-loaded berberine chloride and evodiamine ethosomes for treatment of melanoma. International Journal of Pharmaceutics, 581, 119278. https://doi.org/10.1016/j.ijpharm.2020.119278 [Google Scholar] [PubMed] [CrossRef]
129. Kollipara, R. K., Tallapaneni, V., Sanapalli, B. K. R., Kumar, G. V., Karri, V. V. S. R. (2019). Curcumin loaded ethosomal vesicular drug delivery system for the treatment of melanoma skin cancer. Research Journal of Pharmacy and Technology, 12(4), 1783–1792. https://doi.org/10.5958/0974-360X.2019.00298.1 [Google Scholar] [CrossRef]
130. Khan, N. R., Wong, T. W. (2018). 5-Fluorouracil ethosomes-skin deposition and melanoma permeation synergism with microwave. Artificial Cells, Nanomedicine, and Biotechnology, 46(sup1), 568–577. https://doi.org/10.1080/21691401.2018.1431650 [Google Scholar] [PubMed] [CrossRef]
131. Din, F. U., Aman, W., Ullah, I., Qureshi, O. S., Mustapha, O. et al. (2017). Effective use of nanocarriers as drug delivery systems for the treatment of selected tumors. International Journal of Nanomedicine, 12, 7291. https://doi.org/10.2147/IJN.S146315 [Google Scholar] [PubMed] [CrossRef]
132. Pantshwa, J. M., Kondiah, P. P. D., Choonara, Y. E., Marimuthu, T., Pillay, V. (2020). Nanodrug delivery systems for the treatment of ovarian cancer. Cancers, 12(1), 213. https://doi.org/10.3390/cancers12010213 [Google Scholar] [PubMed] [CrossRef]
133. Yu, F., Jiang, F., Tang, X., Wang, B. (2018). N-octyl-N-arginine-chitosan micelles for gambogic acid intravenous delivery: Characterization, cell uptake, pharmacokinetics, and biodistribution. Drug Development and Industrial Pharmacy, 44(4), 615–623. https://doi.org/10.1080/03639045.2017.1405973 [Google Scholar] [PubMed] [CrossRef]
134. Fathi, M., Majidi, S., Zangabad, P. S., Barar, J., Erfan-Niya, H. (2018). Chitosan-based multifunctional nanomedicines and theranostics for targeted therapy of cancer. Medicinal Research Reviews, 38(6), 2110–2136. https://doi.org/10.1002/med.21506 [Google Scholar] [PubMed] [CrossRef]
135. Ghosh, B., Biswas, S. (2021). Polymeric micelles in cancer therapy: State of the art. Journal of Controlled Release, 332(6), 127–147. https://doi.org/10.1016/j.jconrel.2021.02.016 [Google Scholar] [PubMed] [CrossRef]
136. Lu, Y., Miao, L., Wang, Y., Xu, Z., Zhao, Y. et al. (2016). Curcumin micelles remodel tumor microenvironment and enhance vaccine activity in an advanced melanoma model. Molecular Therapy, 24(2), 364–374. https://doi.org/10.1038/mt.2015.165 [Google Scholar] [PubMed] [CrossRef]
137. Patra, A., Satpathy, S., Shenoy, A. K., Bush, J. A., Kazi, M. et al. (2018). Formulation and evaluation of mixed polymeric micelles of quercetin for treatment of breast, ovarian, and multidrug resistant cancers. International Journal of Nanomedicine, 13, 2869. [Google Scholar] [PubMed]
138. Gera, M., Sharma, N., Ghosh, M., Huynh, D. L., Lee, S. J. et al. (2017). Nanoformulations of curcumin: An emerging paradigm for improved remedial application. Oncotarget, 8(39), 66680–66698. https://doi.org/10.18632/oncotarget.19164 [Google Scholar] [PubMed] [CrossRef]
139. Mughees, M., Wajid, S., Samim, M. (2020). Cytotoxic potential of Artemisia absinthium extract loaded polymeric nanoparticles against breast cancer cells: Insight into the protein targets. International Journal of Pharmaceutics, 586, 119583. https://doi.org/10.1016/j.ijpharm.2020.119583 [Google Scholar] [PubMed] [CrossRef]
140. Fang, L., Fan, H., Guo, C., Cui, L., Zhang, P. et al. (2019). Novel mitochondrial targeting multifunctional surface charge-reversal polymeric nanoparticles for cancer treatment. Journal of Biomedical Nanotechnology, 15(11), 2151–2163. https://doi.org/10.1166/jbn.2019.2854 [Google Scholar] [PubMed] [CrossRef]
141. Abdelaziz, H. M., Freag, M. S., Elzoghby, A. O. (2019). Solid lipid nanoparticle-based drug delivery for lung cancer. In: Nanotechnology-based targeted drug delivery systems for lung cancer, pp. 95–121. Academic Press. [Google Scholar]
142. Akanda, M., Getti, G., Nandi, U., Mithu, M. S., Douroumis, D. (2021). Bioconjugated solid lipid nanoparticles (SLNs) for targeted prostate cancer therapy. International Journal of Pharmaceutics, 599, 120416. https://doi.org/10.1016/j.ijpharm.2021.120416 [Google Scholar] [PubMed] [CrossRef]
143. Patel, P., Raval, M., Airao, V., Bhatt, V., Shah, P. (2022). Silibinin loaded inhalable solid lipid nanoparticles for lung targeting. Journal of Microencapsulation, 39(1), 1–24. https://doi.org/10.1080/02652048.2021.2002448 [Google Scholar] [PubMed] [CrossRef]
144. Farhadi, A., Homayouni Tabrizi, M., Sadeghi, S., Vala, D., Khosravi, T. (2023). Targeted delivery and anticancer effects of chrysin-loaded chitosan-folic acid coated solid lipid nanoparticles in pancreatic malignant cells. Journal of Biomaterials Science, Polymer Edition, 34(3), 315–333. https://doi.org/10.1080/09205063.2022.2121589 [Google Scholar] [PubMed] [CrossRef]
145. Ajnai, G., Chiu, A., Kan, T., Cheng, C. C., Tsai, T. H. et al. (2014). Trends of gold nanoparticle-based drug delivery system in cancer therapy. Journal of Experimental & Clinical Medicine, 6(6), 172–178. https://doi.org/10.1016/j.jecm.2014.10.015 [Google Scholar] [CrossRef]
146. Kong, F. Y., Zhang, J. W., Li, R. F., Wang, Z. X., Wang, W. J. et al. (2017). Unique roles of gold nanoparticles in drug delivery, targeting and imaging applications. Molecules, 22(9), 1445. https://doi.org/10.3390/molecules22091445 [Google Scholar] [PubMed] [CrossRef]
147. Lee, Y. J., Ahn, E. Y., Park, Y. (2019). Shape-dependent cytotoxicity and cellular uptake of gold nanoparticles synthesized using green tea extract. Nanoscale Research Letters, 14(1), 129. https://doi.org/10.1186/s11671-019-2967-1 [Google Scholar] [PubMed] [CrossRef]
148. Thiruvengadam, M., Rajakumar, G., Chung, I. M. (2018). Nanotechnology: Current uses and future applications in the food industry. 3 Biotech, 8(1), 74. https://doi.org/10.1007/s13205-018-1104-7 [Google Scholar] [PubMed] [CrossRef]
149. Wu, C., Lee, S. -L., Taylor, C., Li, J., Chan, Y. -M. et al. (2020). Scientific and regulatory approach to botanical drug development: A U.S. FDA perspective. Journal of Natural Products, 83(2), 552–562. https://doi.org/10.1021/acs.jnatprod.9b00949 [Google Scholar] [PubMed] [CrossRef]
150. Wiernik, P. H. (2016). Alvocidib (flavopiridol) for the treatment of chronic lymphocytic leukemia. Expert Opinion on Investigational Drugs, 25(6), 729–734. https://doi.org/10.1517/13543784.2016.1169273 [Google Scholar] [PubMed] [CrossRef]
151. Mehta, N., Mehta, K., Saini, R. (2022). Current status of commercial anticancer phytochemicals and their derivatives: Natural anticancer bioactive compounds. In: Handbook of research on advanced phytochemicals and plant-based drug discovery, pp. 141–162. IGI Global. [Google Scholar]
152. Talukdar, A., Kundu, B., Sarkar, D., Goon, S., Mondal, M. A. (2022). Topoisomerase I inhibitors: Challenges, progress and the road ahead. European Journal of Medicinal Chemistry, 236(80), 114304. https://doi.org/10.1016/j.ejmech.2022.114304 [Google Scholar] [PubMed] [CrossRef]
153. Brown, D., Bacha, J. A., Geller, J. I., Thompson, P. A., Oesterheld, J. E. et al. (2022). A pilot clinical study of VAL-413 (oral irinotecan HCl) in patients with recurrent pediatric solid tumors. Journal of Clinical Oncology, 40(16_suppl), TPS10063. https://doi.org/10.1200/JCO.2022.40.16_suppl.TPS10063 [Google Scholar] [CrossRef]
154. Fakhri, S., Abbaszadeh, F., Jorjani, M., Pourgholami, M. H. (2021). The effects of anticancer medicinal herbs on vascular endothelial growth factor based on pharmacological aspects: A review study. Nutrition and Cancer, 73(1), 1–15. https://doi.org/10.1080/01635581.2019.1673451 [Google Scholar] [PubMed] [CrossRef]
155. Hur, J., Ghosh, M., Kim, T. H., Park, N., Pandey, K. et al. (2021). Synergism of AZD6738, an ATR inhibitor, in combination with belotecan, a camptothecin analogue, in chemotherapy-resistant ovarian cancer. International Journal of Molecular Sciences, 22(3), 1223. https://doi.org/10.3390/ijms22031223 [Google Scholar] [PubMed] [CrossRef]
156. Giannini, G. (2014). Camptothecin and analogs. In: Natural products in medicinal chemistry, pp. 181–224. https://doi.org/10.1002/9783527676545.ch05 [Google Scholar] [CrossRef]
157. Braybrooke, J. P., Ranson, M., Manegold, C., Mattson, K., Thatcher, N. et al. (2003). Phase II study of exatecan mesylate (DX-8951f) as first line therapy for advanced non-small cell lung cancer. Lung Cancer, 41(2), 215–219. https://doi.org/10.1016/S0169-5002(03)00190-9 [Google Scholar] [PubMed] [CrossRef]
158. Trocóniz, I. F., Cendrós, J. M., Soto, E., Pruñonosa, J., Perez-Mayoral, A. et al. (2012). Population pharmacokinetic/pharmacodynamic modeling of drug-induced adverse effects of a novel homocamptothecin analog, elomotecan (BN80927in a Phase I dose finding study in patients with advanced solid tumors. Cancer Chemotherapy and Pharmacology, 70(2), 239–250. https://doi.org/10.1007/s00280-012-1906-y [Google Scholar] [PubMed] [CrossRef]
159. Joerger, M., Hess, D., Delmonte, A., Gallerani, E., Barbieri, P. et al. (2015). Phase-I dose finding and pharmacokinetic study of the novel hydrophilic camptothecin ST-1968 (namitecan) in patients with solid tumors. Investigational New Drugs, 33(2), 472–479. https://doi.org/10.1007/s10637-015-0219-5 [Google Scholar] [PubMed] [CrossRef]
160. Rahier, N. J., Thomas, C. J., Hecht, S. M. (2005). Camptothecin and its analogs. Anticancer Agents from Natural Products, 5, 22. [Google Scholar]
161. Dhupal, M., Chowdhury, D. (2020). Phytochemical-based nanomedicine for advanced cancer theranostics: Perspectives on clinical trials to clinical use. International Journal of Nanomedicine, 15, 9125–9157. https://doi.org/10.2147/IJN.S259628 [Google Scholar] [PubMed] [CrossRef]
162. Whitehead, R. P., Moon, J., McCachren, S. S., Hersh, E. M., Samlowski, W. E. et al. (2004). A phase II trial of vinorelbine tartrate in patients with disseminated malignant melanoma and one prior systemic therapy: A Southwest oncology group study. Cancer, 100(8), 1699–1704. https://doi.org/10.1002/cncr.20183 [Google Scholar] [PubMed] [CrossRef]
163. Meng, F., Zou, B., Yang, R., Duan, Q., Qian, T. (2022). The diagnostic efficiency of the perfusion-related parameters in assessing the vascular disrupting agent (CA4P) response in a rabbit VX2 liver tumor model. Acta Radiologica, 63(9), 1147–1156. https://doi.org/10.1177/02841851211032450 [Google Scholar] [PubMed] [CrossRef]
164. Nishio, M., Satouchi, M., Horiike, A., Horio, Y., Sunaga, Y. et al. (2018). Phase 1 study of ombrabulin in combination with docetaxel and cisplatin in Japanese patients with advanced solid tumors. Japanese Journal of Clinical Oncology, 48(4), 322–328. https://doi.org/10.1093/jjco/hyy026 [Google Scholar] [PubMed] [CrossRef]
165. Porter, K., Fairlie, W. D., Laczka, O., Delebecque, F., Wilkinson, J. (2020). Idronoxil as an anticancer agent: Activity and mechanisms. Current Cancer Drug Targets, 20(5), 341–354. https://doi.org/10.2174/1568009620666200102122830 [Google Scholar] [PubMed] [CrossRef]
166. Chen, X., Gu, J., Wu, Y., Liang, P., Shen, M. et al. (2020). Clinical characteristics of colorectal cancer patients and anti-neoplasm activity of genistein. Biomedicine & Pharmacotherapy, 124(3), 109835. https://doi.org/10.1016/j.biopha.2020.109835 [Google Scholar] [PubMed] [CrossRef]
167. Heppt, M. V., Dykukha, I., Graziadio, S., Salido-Vallejo, R., Chapman-Rounds, M. et al. (2022). Comparative efficacy and safety of tirbanibulin for actinic keratosis of the face and scalp in Europe: A systematic review and network meta-analysis of randomized controlled trials. Journal of Clinical Medicine, 11(6), 1654. https://doi.org/10.3390/jcm11061654 [Google Scholar] [PubMed] [CrossRef]
168. Jiang, W., Li, X., Dong, S., Zhou, W. (2021). Betulinic acid in the treatment of tumour diseases: Application and research progress. Biomedicine & Pharmacotherapy, 142(246), 111990. https://doi.org/10.1016/j.biopha.2021.111990 [Google Scholar] [PubMed] [CrossRef]
169. Ma, W., Yang, Y., Zhu, J., Jia, W., Zhang, T. et al. (2022). Biomimetic nanoerythrosome-coated aptamer-DNA tetrahedron/maytansine conjugates: pH-responsive and targeted cytotoxicity for HER2-positive breast cancer. Advanced Materials, 34(46), 2109609. https://doi.org/10.1002/adma.202109609 [Google Scholar] [PubMed] [CrossRef]
170. Calcabrini, C., Maffei, F., Turrini, E., Fimognari, C. (2020). Sulforaphane potentiates anticancer effects of doxorubicin and cisplatin and mitigates their toxic effects. Frontiers in Pharmacology, 11, 567. https://www.frontiersin.org/articles/10.3389/fphar.2020.00567 [Google Scholar] [PubMed]
171. Oketch-Rabah, H. A., Roe, A. L., Rider, C. V., Bonkovsky, H. L., Giancaspro, G. I. et al. (2020). United States pharmacopeia (USP) comprehensive review of the hepatotoxicity of green tea extracts. Toxicology Reports, 7(11), 386–402. https://doi.org/10.1016/j.toxrep.2020.02.008 [Google Scholar] [PubMed] [CrossRef]
172. Abd Wahab, N. A., Lajis, N. H., Abas, F., Othman, I., Naidu, R. (2020). Mechanism of anti-cancer activity of curcumin on androgen-dependent and androgen-independent prostate cancer. Nutrients, 12(3), 679. https://doi.org/10.3390/nu12030679 [Google Scholar] [PubMed] [CrossRef]
173. Renner, O., Mayer, M., Leischner, C., Burkard, M., Berger, A. et al. (2022). Systematic review of Gossypol/AT-101 in cancer clinical trials. Pharmaceuticals, 15(2), 144. https://doi.org/10.3390/ph15020144 [Google Scholar] [PubMed] [CrossRef]
174. Gong, Q., Hu, J., Wang, P., Li, X., Zhang, X. (2021). A comprehensive review on β-lapachone: Mechanisms, structural modifications, and therapeutic potentials. European Journal of Medicinal Chemistry, 210(7), 112962. https://doi.org/10.1016/j.ejmech.2020.112962 [Google Scholar] [PubMed] [CrossRef]
175. Eren, E., Watts, N. R., Sackett, D. L., Wingfield, P. T. (2021). Conformational changes in tubulin upon binding cryptophycin-52 reveal its mechanism of action. Journal of Biological Chemistry, 297(4), 101138. https://doi.org/10.1016/j.jbc.2021.101138 [Google Scholar] [PubMed] [CrossRef]
176. Xie, L., Zhu, G., Shang, J., Chen, X., Zhang, C. et al. (2021). An overview on the biological activity and anti-cancer mechanism of lovastatin. Cellular Signalling, 87(1), 110122. https://doi.org/10.1016/j.cellsig.2021.110122 [Google Scholar] [PubMed] [CrossRef]
177. Xia, F., Zhang, M. (2022). Kanglaite injection combined with transcatheter arterial chemoembolization for advanced hepatocellular carcinoma: A meta-analysis. Annals of Oncology Case Reports Open, 5(1), 1–7. [Google Scholar]
178. Mykkänen, A. J. H., Taskinen, S., Neuvonen, M., Paile-Hyvärinen, M., Tarkiainen, E. K. et al. (2022). Genomewide association study of simvastatin pharmacokinetics. Clinical Pharmacology and Therapeutics, 112(3), 676–686. https://doi.org/10.1002/cpt.2674 [Google Scholar] [PubMed] [CrossRef]
179. Gallego-Jara, J., Lozano-Terol, G., Sola-Martínez, R. A., Cánovas-Díaz, M., de Diego Puente, T. (2020). A compressive review about taxol(®History and future challenges. Molecules, 25(24), 5986. https://doi.org/10.3390/molecules25245986 [Google Scholar] [PubMed] [CrossRef]
180. Wang, S., Liu, J., Lv, H., Huang, X., Dong, P. et al. (2022). Complete regression of xenografted breast tumors by dextran-based dual drug conjugates containing paclitaxel and docosahexaenoic acid. European Journal of Medicinal Chemistry, 240, 114567. https://doi.org/10.1016/j.ejmech.2022.114567 [Google Scholar] [PubMed] [CrossRef]
181. Li, X., Li, J., Xu, J., Chen, K., Zhang, Z. et al. (2021). Nanostructure of functional larotaxel liposomes decorated with guanine-rich quadruplex nucleotide-lipid derivative for treatment of resistant breast cancer. Small, 17(13), 2007391. https://doi.org/10.1002/smll.202007391 [Google Scholar] [PubMed] [CrossRef]
182. Al-Hilfi, A., Walker, K. D. (2022). Biocatalysis of precursors to new-generation SB-T-taxanes effective against paclitaxel-resistant cancer cells. Archives of Biochemistry and Biophysics, 719, 109165. https://doi.org/10.1016/j.abb.2022.109165 [Google Scholar] [PubMed] [CrossRef]
183. Karati, D., Kumar, D. (2022). Exploring the structural and functional requirements of phyto-compounds and their synthetic scaffolds as anticancer agents: Medicinal chemistry perspective. Pharmacological Research-Modern Chinese Medicine, 4(11), 100123. https://doi.org/10.1016/j.prmcm.2022.100123 [Google Scholar] [CrossRef]
184. Zumbar, C. T., Usubalieva, A., King, P. D., Li, X., Mifsud, C. S. et al. (2018). The CNS penetrating taxane TPI 287 and the AURKA inhibitor alisertib induce synergistic apoptosis in glioblastoma cells. Journal of Neuro-Oncology, 137(3), 481–492. https://doi.org/10.1007/s11060-018-2755-2 [Google Scholar] [PubMed] [CrossRef]
185. Vermunt, M. A., Bergman, A. M., van der Putten, E., Beijnen, J. H. (2021). The intravenous to oral switch of taxanes: Strategies and current clinical developments. Future Oncology, 17(11), 1379–1399. https://doi.org/10.2217/fon-2020-0876 [Google Scholar] [PubMed] [CrossRef]
186. Soffietti, R., Ahluwalia, M., Lin, N., Rudà, R. (2020). Management of brain metastases according to molecular subtypes. Nature Reviews Neurology, 16(10), 557–574. https://doi.org/10.1038/s41582-020-0391-x [Google Scholar] [PubMed] [CrossRef]
187. Hedvig Arnamo, A., Huitema, A. D. R., Beijnen, J. H., Nuijen, B. (2021). Stability study of concentrate-solvent mixture & infusion solutions of Jevtana® cabazitaxel for extended multi-dosing. Journal of Oncology Pharmacy Practice, 10781552211016080(5), 1035–1041. https://doi.org/10.1177/10781552211016079 [Google Scholar] [PubMed] [CrossRef]
188. Barrera, E., Hernández-Benitez, R. I., González-González, C. A., Escalante, C. H., Fuentes-Benítes, A. et al. (2022). Synthesis of diarylamines and methylcarbazoles and formal total synthesis of alkaloids ellipticine and olivacine. European Journal of Organic Chemistry, 2022(24), e202200364. https://doi.org/10.1002/ejoc.202200364 [Google Scholar] [CrossRef]
189. Zhang, C., Lam, S. S. Y., Leung, G. M. K., Tsui, S. P., Yang, N. et al. (2020). Sorafenib and omacetaxine mepesuccinate as a safe and effective treatment for acute myeloid leukemia carrying internal tandem duplication of Fms-like tyrosine kinase 3. Cancer, 126(2), 344–353. https://doi.org/10.1002/cncr.32534 [Google Scholar] [PubMed] [CrossRef]
190. Xie, J., Yang, Z., Zhou, C., Zhu, J., Lee, R. J. et al. (2016). Nanotechnology for the delivery of phytochemicals in cancer therapy. Biotechnology Advances, 34(4), 343–353. https://doi.org/10.1016/j.biotechadv.2016.04.002 [Google Scholar] [PubMed] [CrossRef]
191. Upaganlawar, A., Polshettiwar, S., Raut, S., Tagalpallewar, A., Pande, V. (2022). Effective cancer management: Inimitable role of phytochemical based nano-formulations. Current Drug Metabolism, 23(11), 869–881. https://doi.org/10.2174/1389200223666220905162245 [Google Scholar] [PubMed] [CrossRef]
Cite This Article
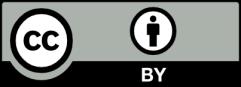
This work is licensed under a Creative Commons Attribution 4.0 International License , which permits unrestricted use, distribution, and reproduction in any medium, provided the original work is properly cited.