Open Access
REVIEW
Circulating tumor cells and circulating tumor DNA in breast cancer diagnosis and monitoring
1 Infectious Diseases Research Center, Birjand University of Medical Sciences, Birjand, 9717853577, Iran
2 Midwifery Department, Ministry of Health and Medical Education, Tehran, 9413933336, Iran
3 Student Research Committee, Birjand University of Medical Sciences, Birjand, 9717853577, Iran
4 Department of Health Assistant, Shiraz University of Medical Sciences, Shiraz, 7134814336, Iran
5 Social Determinants of Health Research Center, Birjand University of Medical Sciences, Birjand, 32048321, Iran
* Corresponding Author: Hamid Salehiniya,
Oncology Research 2023, 31(5), 667-675. https://doi.org/10.32604/or.2023.028406
Received 16 December 2022; Accepted 19 May 2023; Issue published 21 July 2023
Abstract
Liquid biopsy, including both circulating tumor cells and circulating tumor DNA, is becoming more popular as a diagnostic tool in the clinical management of breast cancer. Elevated concentrations of these biomarkers during cancer treatment may be used as markers for cancer progression as well as to understand the mechanisms underlying metastasis and treatment resistance. Thus, these circulating markers serve as tools for cancer assessing and monitoring through a simple, non-invasive blood draw. However, despite several study results currently noting a potential clinical impact of ctDNA mutation tracking, the method is not used clinically in cancer diagnosis among patients and more studies are required to confirm it. This review focuses on understanding circulating tumor biomarkers, especially in breast cancer.Graphic Abstract
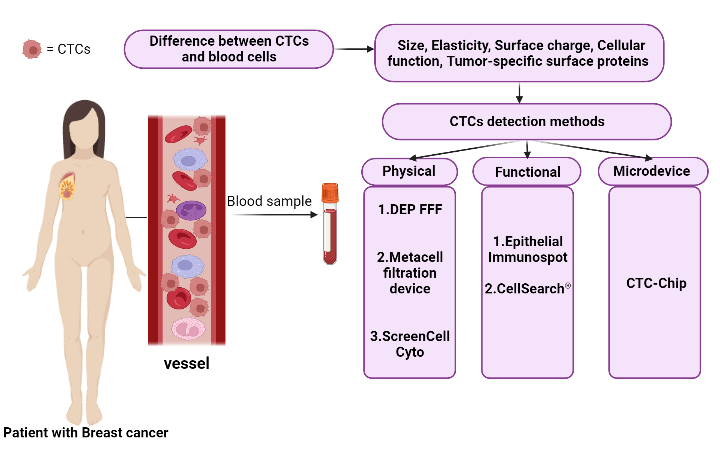
Keywords
Breast cancer (BC) is the most prevalent malignancy affecting women worldwide. The 5-year survival rate in individuals with a localized breast cancer diagnosis and no nodal involvement is 99% compared to 27% for patients with distant metastatic disease [1–3]. Breast cancer is a heterogeneous and complex condition with a wide range of treatment responses, where the incidence of cancer rises with age due to an accumulation of somatic mutations in the mammary glands [4,5]. Despite improvements in the treatment of breast cancer, more than 8.2 million individuals still die every year due to a lack of a reliable method to detect the disease early and to monitor the response to therapy effectively [6,7]. Among all new cases, 6%–7% are diagnosed with de-novo metastatic disease, and about 30% of patients initially identified in earlier stages eventually relapse in distant sites. As a result, it is always a challenge for researchers to consider methods to help with cancer detection and monitoring. In addition, the techniques used today for the early detection of breast cancer have remained unchanged for more than 20 years and can be invasive to the patient’s physical health during the diagnosis process. For example, in radiology, ultrasound detection, and MRI scans, solid biopsies are commonly used methods for cancer detection which are limited by poor sensitivity, overdiagnosis, false-positive rates, ineffective detection of minimal residual disease, and incapable of monitoring dynamic changes [8,9].
Thus, a breast cancer prognosis worsens with the absence of young women’s early screening programs and efficient diagnostic tools in general [10]. These drawbacks emphasize how critical it is to create new tools and methods for the early detection and management of breast cancer. Liquid biopsy is a new minimally invasive technique for the early detection and risk management of breast cancer. Since liquid biopsy is a technique for examining nonsolid biological tissues, hence it has good potential to overcome the drawbacks of conventional approaches [11,12].
The purpose of this review is to summarize technologies for Circulating tumor cells (CTCs) and circulating tumor DNA (ctDNA) detection as well as their clinical applications as complementary tools to improve the outcome of patients with breast cancer.
CTCs and ctDNA are new, noninvasive, and multifunctional biomarkers used in the liquid biopsy which enable early diagnosis, precise prognosis, therapeutic target selection, spatiotemporal monitoring of metastasis, and monitoring of therapeutic response as well as resistance. Different tumor types at different stages release CTCs and ctDNA, which could provide complementary information for clinical decisions [11].
Since the 1860s, when tumor cells were first discovered in patients’ peripheral blood, there have been tremendous advancements in the ability to isolate CTCs from a diverse population of blood cells [12]. CTCs are released from primary tumors, travel via the circulatory system, and are responsible for the growth of metastatic (or secondary) malignancies at distant locations throughout the body [13]. Their percentage in the blood is very low, with only around one CTC being identified for every million leukocytes [14]. According to morphological studies, CTCs have different shapes based on the stage and/or kind of the tumor C. Furthermore, compared to their isolated CTC counterparts, aggregated CTCs have been shown to spread to further locations in the body after affixing to cells such as fibroblasts, platelets, etc. Thus, these cellular aggregates are shielded from oxidative damage and can evade detection by the immune system of the host organism, which can contribute to the development of metastases [15,16].
CTCs have become increasingly important in the detection of cancers due to their ease of collection and ability to provide real-time information about the tumor’s state without the need for invasive tissue biopsies. Unlike typical blood indicators, CTC levels have been shown to change in a more dynamic manner, closely tracking changes in the tumor status with greater precision [17]. Additionally, CTC counts have been shown to be a more accurate predictor of treatment response, with lower CTC counts being associated with improved overall survival in a large cohort of breast cancer patients [18]. Additionally, CTCs have demonstrated encouraging outcomes in the early identification of numerous cancer forms, including lung cancer, though only in a small subset of individuals with chronic obstructive pulmonary disease [19].
A highly effective diagnostic method based on a patient’s genetic and epigenetic makeup has been made possible by ctDNA in precision medicine [20]. ctDNA is highly heterogeneous both in size and composition, and can be detected in different body fluids [21]. The mechanism though which cfDNA is released by cells is yet to be fully clarified. Electrophoresis assays demonstrated that most fragments range between 180 and 200 base pairs (bp) and are often associated with histone proteins that form the nucleosome, suggesting that apoptotic cells could be one of the most important source of cfDNA [22,23]. These observations were strongly related to a rapid increase in circulating nucleosomes during anticancer treatments and by a rapid decline at disease progression, supporting the idea that the quantification of nucleosome bodies can represent an efficient index of responsiveness to the therapy [24].
In cancer patients, ctDNA is found in a variable but usually very low percentage (0.01%–1.0%) of the total cfDNA, which is usually less than 1 ng/μL, and varies depending on the stage, location, or vascularization of the tumor [25]. The global cfDNA can be easily quantified and is known to rise in breast cancer patients compared to healthy subjects [26]. The concentration of circulating cell-free DNA among healthy individuals and those with various types of cancer was measured at 13 ± 3 ng/mL and 180 ± 38 ng/mL, respectively [27].
ctDNA detection was significantly associated with the molecular subtypes, with the Basal-like and the Luminal-A subtypes showing the highest (86%) and lowest (0%) ctDNA detection rates, respectively [28]. Elevated levels of circulating tumor cells have been associated with a worse prognosis [29,30]. In contrast to early, non-metastatic breast cancer, ctDNA is detectable in the majority of metastatic breast cancers. Zhou et al. reported that 85.71% of stage IV/M1 patients carried tumor-derived mutations in blood, compared to only 57.81% of stage I–III/M0 patients [31]. In another study, Catarino et al. reported elevated levels of circulating DNA in breast cancer patients compared to control individuals (105.2 vs. 77.06 ng/mL, p < 0.001). They also found statistically significant differences in circulating DNA amounts in patients before and after breast surgery (105.2 vs. 59.0 ng/mL, p = 0.001) [32].
ctDNA is also related to tumor volume in breast, ovarian, lung, colorectal, and stomach cancers, which results in a reduced overall survival time [33]. Contradictory findings from various studies suggest that concentration is not related to general or advancement-free existence [34]. The limitations of ctDNA for diagnostic purposes can be inferred from that research, though ctDNA can still be employed to track tumor growth. Clinical uses for ctDNA include disease monitoring and diagnosis. Through the use of ctDNA, certain studies have been able to locate mutations in a patient’s tumor. For instance, a PIK3CA mutation provided a 95% accurate diagnosis of breast cancer [35]. As a result, ctDNA may be utilized to detect interesting mutations and genetic heterogeneity. CtDNA can also be used to monitor the effects of treatment by looking for mutation-driven resistance [36]. Endocrine treatment resistance to the ESR1 mutation is a frequent feature in patients with metastatic breast cancer [37]. Early diagnosis of this type of mutation and treatment prior to clinical progression are both conceivable utilizing ctDNA [38].
Technologies for CTC detection and characterization
CTCs are uncommon and infrequent neoplastic cells that should be detected using a high-level detection platform, the proper tools, and methodologies because of their low peripheral blood concentration (almost 1 cell per 105 to 107 mononuclear cells) [39,40]. Despite the availability of numerous techniques and technologies for identifying CTCs based on their physical (such as size, elasticity, and surface charge) and biological (such as cellular function) features as well as the expression of tumor-specific surface protein characteristics (Fig. 1), the Food and Drug Administration (FDA) has only approved the CellSearch® system as a platform for counting CTCs thus far [41,42].
Figure 1: Summary of CTCs detection methods in patient blood samples and the basis of these methods.
The CellSearch® was designed to enable fluorescent labelling, immunomagnetic enrichment, and detection of CTCs. This approach utilizes ferrofluid nanoparticles coated with EpCAM-targeting antibodies in a CTC-enrichment stage, allowing for efficient capture of CTCs from blood samples [41,43]. This strategy can be used to monitor patients after chemotherapy or surgery, as well as to treat breast cancer and other cancers [39]. However, this approach is time-consuming, requires expensive tools, and involves antibody staining, which would hamper the wide application of this technology [40]. CellSearch®’s enrichment approach results in cell loss, which affects the system’s sensitivity despite the technology’s proven clinical validity. Additionally, it only selects CTCs that express EpCAM, missing other CTC phenotypes such as mesenchymal and stem cell-like tumor cells that express EpCAM at low or zero levels [41].
In addition to Cell Search as an immunobead assay, there are other methods and approaches for detecting CTCs, including physical property-based assays (such as Dielectrophoretic field-flow fractionation (DEP-FFF), the Metacell filtration device, ScreenCell Cyto, etc.), functional assays (such as the Epithelial Immunospot (EPISPOT) assay, and etc.), microdevices, as well as microfluidic platforms (such as the CTC-Chip, which consists of microposts coated with anti-EpCAM antibodies, and etc.) (Fig. 1) [42].
Kong et al. [44] used the Drop Cell as a single-cell isolation platform for CTC isolation at single-cell resolution. In order to assess the genetic heterogeneity of ctDNA and CTCs in patients with lung and breast cancer, they actually constructed a standardized sample processing methodology which allowed for the concurrent separation of CTC and ctDNA followed by targeted amplicon sequencing. To isolate CTCs at single-cell resolution, they used CD45-antibody negative selection employing a single-cell capture. The results of this study indicated that CTCs and ctDNA had a higher degree of concordance with the metastatic tumor than with the primary tumor. As a conclusion, a standardized sample processing and data analysis workflow for the concurrent analysis of CTCs and ctDNA was successful in separating the heterogeneity of metastatic tumors that were circulating as well as the progressive genomic changes that may guide the selection of an appropriate therapy against evolving tumor clonality [44].
Based on cell size and deformability, Lopes et al. evaluated and contrasted the RUBYchipTM (size-based microfluidic device) system’s performance vs. that of the Cell Search® system for CTC capture. In isolated CTCs, the expression of HER2 was evaluated and compared to tissue biopsy. The study found that, on average, the RUBYchipTM was up to ten times more effective at isolating CTCs compared to the Cell Search® system. Additionally, a precise assessment of various CTC subpopulations, including HER2+ CTCs, was given. The study’s results indicated that liquid biopsy, when used in the clinic with the RUBYchipTM, can overcome the limitations of histological testing and determine a patient’s HER2 status in real-time, allowing for more precise treatment planning as the disease progresses [41].
CTC detection has been proven in patients with both early-stage and metastatic breast cancer [45,46]. Among the benefits of using CTC are ease of collection, non-invasiveness, the possibility of continuous evaluation, as well as analysis of the total tumor burden rather than a limited part of a tumor. Currently, CTC is used in breast cancer to gain prognostic information, monitor the effectiveness of treatment and therapeutic interventions, detect the disease stages early, investigate drug sensitivity, and discover new drugs for personalized treatment [47]. However, the practical use of CTCs is limited due to their rarity and heterogeneity, as well as problems with initial culture, necessitating the development of easier detection methods compared to the existing ones [48]. It appears that CTC count is a criterion for evaluating treatment efficacy, and patients with metastatic breast cancer who had a higher CTC count experienced more metastases over time [49]. Also, CTC cells appear to be a better model for studying the malignant behavior of breast cancer than existing cell lines. Zhao et al. revealed that the CTC-3 cell line grows more aggressively in vitro and in vivo than the commonly used MCF-7 breast cancer cell line [50].
Given the numerous benefits of CTCs in the diagnosis and early treatment of breast tumors, the presence of CTC clusters in primary breast cancer may be considered an important risk factor for disease progression [47]. Further research is required, however, to address the prognostic potential of CTC in the early stages of breast cancer [46].
Kroll et al. discovered the peripheral blood circulation of CTCs in patients with primary non-metastatic breast cancer, shortly after diagnosis and prior to surgical intervention for therapeutic purposes [46].
Using conventional and epithelial-based methods, it is difficult to analyze and identify CTCs in the early stages of breast cancer. However, the Reduzzi et al.’s study showed that marker-independent approaches for CTC evaluation would improve diagnosis and that CTC is more common in patients with early-stage breast cancer than in patients with metastatic breast cancer [43].
Elevated levels of CTCs have been confirmed as an independent prognostic factor in metastatic breast cancer. Xie et al. discovered that CTC had a prognostic value in patients with metastatic breast cancer. In this study, 38 patients with metastatic breast cancer were enrolled. CTCs, collected in vivo by the Cell Collector method in Chinese patients with metastatic breast cancer, showed prognostic significance. It seems that the increase of CTCs in the blood is a sign of the progression of cancer and the worsening of the disease. This is because, as the number of CTCs in the blood increases, so do the genetic heterogeneity of these cells and their interaction with the internal environment of the body [45]. Stefanovic et al. showed that in patients with metastatic breast cancer, CTCs might not only develop their genetic potential but also communicate with their surroundings, including chemokine systems, hemocytes, and extracellular matrix components, to regulate the organ-specific metastases of breast cancer. It appears that the degree of HER2 status matching between CTCs and primary tumors or metastatic sites can reach 77% or 67%, implying that studying CTC HER2 expression can guide clinical HER2-targeted therapy [51]. Deutsch et al. examined the CTC status of 264 patients with metastatic breast cancer before and after 4 weeks of a new line of palliative systemic therapy. According to the findings, HER2-targeted therapy appears to reduce the overall CTC count in patients with metastatic breast cancer [52].
Technologies for DNA detection and characterization
Fig. 2 provides a summary of the technologies, their underlying principles, and the workflow used for the detection of ctDNA.
Figure 2: Summary detection of ctDNA: Technologies, bases of technologies, and work process of technologies [53–63].
The main techniques employed to evaluate ctDNA are: droplet digital polymerase chain reaction (ddPCR), beads, emulsion, amplification, and magnetics (BEAMing), tagged-amplicon deep sequencing (TAm-Seq), cancer personalized profiling by deep sequencing (CAPP-Seq), whole genome bisulfite sequencing (WGBS-Seq), whole exome sequencing (WES), and whole genome sequencing (WGS) [59,64–66].
An important method in translational cancer research is droplet digital polymerase chain reaction (ddPCR), because of its superior sensitivity and precision for genomic DNA detection or RNA expression [67,68], and could be useful for detecting HER2 amplification levels [69]. However, it can only be used to evaluate the presence of characterizing sequences [65]. In breast cancer, the ddPCR results had high concordance with FISH and IHC-defined HER2 status with a sensitivity of 90.9% and a specificity of 100% [69].
BEAMing combines PCR with flow cytometry and is an alternative sensitive approach which provides molecular information about mutations with a frequency of 1 over 10000 [65,70].
Tagged-amplicon deep sequencing (TAm-Seq) enhanced the identification of low frequency mutations in ctDNA [71]. The main features of TAm-Seq include a high sequencing flux, reduced sequencing time plus cost, and the ability to simultaneously sequence millions of DNA molecules, thereby enabling the analysis of the transcripts and genomes of a species in detail [66]. The detection rate of TAm-Seq was reported >2% [53], with sensitivity and specificity of ~100% [71].
CAPP-Seq is a recent NGS-based ctDNA analysis method that achieves both an ultralow detection limit and broad patient coverage at a reasonable cost and low ctDNA input level, thus allowing the quantitation of ctDNA from early-stage tumors [72–74]. CAPP-Seq identifies alterations in ctDNA/cfDNA using large genomic libraries and individual patient sample sequence signatures. It statistically assesses well-characterized tumor alterations with DNA oligonucleotides to find patient-specific alterations. It can identify multiple mutations in patients with the same type of cancer and assess tumor heterogeneity. It was previously shown to be capable of identifying tumor burdens prior to medical imaging. It can identify many major mutation types including insertions, deletions, single nucleotide variants, copy variants, and rearrangements, but cannot identify fusions [60,66].
Whole genome bisulfite sequencing (WGBS-Seq) is the gold-standard approach to acquiring comprehensive base-pair resolution and quantitative information at most genomic methylated cytokines, allowing for unbiased genome-wide DNA methylation profiling [54]. WGBS is an effective and reliable strategy to identify individually methylated cytosines on a genome-wide scale [75]. Its sensitivity may be lower than other methods, since it includes exomic alterations. It is characterized by low cost and high yield [76].
Whole Genome Sequencing (WGS) and Whole Exome Sequencing (WES) technologies provide novel opportunities for comprehensive profiling of ctDNA by detecting genome-wide rearrangements, somatic chromosomal aberrations and Copy Number Variations [64]. These technologies have become increasingly faster, more sensitive, and cost-effective, making their clinical application more feasible. With the ability to sequence at greater depth, WGS and WES offer a promising approach for accurate and sensitive detection of ctDNA in clinical settings [77,78].
Tumor DNA in the blood is a result of various mechanisms including apoptosis, necrosis, and circulating tumor cell lysis causing a DNA leak into the bloodstream [79]. Various studies have reported a direct correlation between serum levels and tumor burden in breast cancer patients [26,32,80–85], but a diagnostic threshold has not yet been defined [86].
Recent advancements in ctDNA technologies have improved sensitivity and selectivity, allowing ctDNA to be detected in early-stage disease, including early-stage breast cancer [87]. In early breast cancer, ctDNA clearance has been associated with higher rates of complete pathological response after neoadjuvant treatment and with fewer recurrences after radical treatments [88]. Generally, the current clinical application of ctDNA for breast cancer involves real-time monitoring of tumor response [89], detecting drug-resistant clones [90,91], assessing dynamic variations in tumor mutational landscape [92], identifying actionable mutations [93], detecting minimal residual disease [28] and screening of early tumor [94,95]. Since there is no wildly accepted baseline level of ctDNA for breast cancer diagnosis, variations of ctDNA over time do seem to reflect the burden of the disease, determine the prognosis of cancer patients, and help predict therapeutic response [96].
In metastatic disease, ctDNA can aid in selecting the optimal sequencing of treatments [88]. Studies have shown that ctDNA is a reliable tool for monitoring tumor burden dynamics in patients with metastatic breast cancer undergoing systemic therapy [97]. Compared to traditional biomarkers such as CA 15-3 or circulating tumor cells, ctDNA levels have a wider dynamic range and exhibit a stronger correlation with changes in tumor burden. Additionally, ctDNA analysis provides an early measure of treatment response, with up to 53% of patients showing a response within a few weeks of treatment initiation [85]. Serial analysis of ctDNA can also help track clone evolution and predict the development of resistance to therapy, enabling clinical decisions to be made in a timely manner and sparing patients from ineffective treatments. Since ctDNA is believed to be shed by all tumor sites, it has the potential to be a useful tool for addressing tumor heterogeneity and therapeutic resistance in both the adjuvant and metastatic settings, guiding therapy decisions more effectively [96].
Liu et al. [98] demonstrated that higher levels of ctDNA alterations (levels 3–4) were associated with an increased likelihood of liver metastasis. In addition, a novel ctDNA-level RECIST (ctle-RECIST) was developed to evaluate treatment response based on ctDNA alteration levels and variant allele frequency.
Conclusion and Future Directions
One of the most pressing challenges in breast cancer treatment is how to better manage patients with early disease. In recent years, research on the applications of liquid biopsies, including CTCs and ctDNA, has increased dramatically to enhance early diagnosis, detection of recurrence, and personalized treatment. CTCs and ctDNA have great potential for determining prognosis, monitoring therapy, and integrating data processing methodologies. Combining ctDNA and CTC analysis may further improve their prognostic value and clinical utility. As precision and personalized medicine become increasingly important, CTC and ctDNA monitoring can help detect and subtype the disease and identify patients at high risk of relapse. However, the clinical utility of ctDNA mutation tracking needs further investigation before it can become a standard of care for patients with early-stage breast cancer. Standardization of the blood collection process to enhance sample stability, defining ctDNA quantification methods, standardizing ctDNA isolation, and enhancing the sensitivity of ctDNA detection for rare molecular alterations are among the challenges that need to be addressed.
In conclusion, the next generation of liquid biopsy studies will be crucial in establishing the clinical applicability of blood-based genomic profiling. Liquid biopsy techniques offer physicians a relatively inexpensive and non-invasive method for detecting and monitoring early-stage cancers, but it remains to be seen whether treatments based on liquid biopsies will lead to better outcomes.
Acknowledgement: Not applicable.
Funding Statement: No specific funding was obtained for this study.
Author Contributions: Study conception and design: EA, LA, AM and HS. Data collection: EA, LA, MA and AG; analysis and interpretation of results: HS, AM, EA and AG. All authors reviewed the results and approved the draft and final version of the manuscript.
Availability of Data and Materials: The data and material used in the current study are available from the corresponding author upon reasonable request.
Ethics Approval: Not applicable.
Conflicts of Interest: The authors declare that they have no conflicts of interest to report regarding the present study.
References
1. Allahqoli, L., Mazidimoradi, A., Momenimovahed, Z., Rahmani, A., Hakimi, S. et al. (2022). The global incidence, mortality, and burden of breast cancer in 2019: Correlation with smoking, drinking, and drug use. Frontiers in Oncology, 12, 921015. https://doi.org/10.3389/fonc.2022.921015 [Google Scholar] [PubMed] [CrossRef]
2. Torre, L. A., Bray, F., Siegel, R. L., Ferlay, J., Lortet-Tieulent, J. et al. (2015). Global cancer statistics, 2012. CA: A Cancer Journal for Clinicians, 65(2), 87–108. https://doi.org/10.3322/caac.21262 [Google Scholar] [PubMed] [CrossRef]
3. Hassanipour, S., Maghsoudi, A., Rezaeian, S., Arab-Zozani, M., Mokhtari, A. M. et al. (2019). Survival rate of breast cancer in eastern mediterranean region countries: A systematic review and meta-analysis. Annals of Global Health, 85(1), 138. https://doi.org/10.5334/aogh.2521 [Google Scholar] [PubMed] [CrossRef]
4. Prat, A., Perou, C. M. (2011). Deconstructing the molecular portraits of breast cancer. Molecular Oncology, 5(1), 5–23. https://doi.org/10.1016/j.molonc.2010.11.003 [Google Scholar] [PubMed] [CrossRef]
5. Addanki, S., Meas, S., Sarli, V. N., Singh, B., Lucci, A. (2022). Applications of circulating tumor cells and circulating tumor DNA in precision oncology for breast cancers. International Journal of Molecular Sciences, 23(14), 7843. https://doi.org/10.3390/ijms23147843 [Google Scholar] [PubMed] [CrossRef]
6. Ghoncheh, M., Mohammadian-Hafshejani, A., Salehiniya, H. (2015). Incidence and mortality of breast cancer and their relationship to development in Asia. Asian Pacific Journal of Cancer Prevention, 16(14), 6081–6087. https://doi.org/10.7314/APJCP.2015.16.14.6081 [Google Scholar] [PubMed] [CrossRef]
7. Alemzadeh, E., Dehshahri, A., Dehghanian, A. R., Afsharifar, A., Behjatnia, A. A. et al. (2019). Enhanced anti-tumor efficacy and reduced cardiotoxicity of doxorubicin delivered in a novel plant virus nanoparticle. Colloids and Surfaces B: Biointerfaces, 174, 80–86. https://doi.org/10.1016/j.colsurfb.2018.11.008 [Google Scholar] [PubMed] [CrossRef]
8. Lauby-Secretan, B., Scoccianti, C., Loomis, D., Benbrahim-Tallaa, L., Bouvard, V. et al. (2015). Breast-cancer screening—Viewpoint of the IARC working group. New England Journal of Medicine, 372(24), 2353–2358. https://doi.org/10.1056/NEJMsr1504363 [Google Scholar] [PubMed] [CrossRef]
9. Dainiak, N. (2016). Inferences, risk modeling, and prediction of health effects of ionizing radiation. Health Physics, 110(3), 271–273. https://doi.org/10.1097/HP.0000000000000465 [Google Scholar] [PubMed] [CrossRef]
10. Saha, S., Araf, Y., Promon, S. K. (2022). Circulating tumor DNA in cancer diagnosis, monitoring, and prognosis. Journal of the Egyptian National Cancer Institute, 34(1), 8. https://doi.org/10.1186/s43046-022-00109-4 [Google Scholar] [PubMed] [CrossRef]
11. Tan, C. R., Zhou, L., El-Deiry, W. S. (2016). Circulating tumor cells versus circulating tumor DNA in colorectal cancer: Pros and cons. Current Colorectal Cancer Reports, 12(3), 151–161. https://doi.org/10.1007/s11888-016-0320-y [Google Scholar] [PubMed] [CrossRef]
12. Ko, J., Baldassano, S. N., Loh, P. L., Kording, K., Litt, B. et al. (2018). Machine learning to detect signatures of disease in liquid biopsies—A user’s guide. Lab on a Chip, 18(3), 395–405. https://doi.org/10.1039/C7LC00955K [Google Scholar] [PubMed] [CrossRef]
13. Parkinson, D. R., Dracopoli, N., Petty, B. G., Compton, C., Cristofanilli, M. et al. (2012). Considerations in the development of circulating tumor cell technology for clinical use. Journal of Translational Medicine, 10(1), 138. https://doi.org/10.1186/1479-5876-10-138 [Google Scholar] [PubMed] [CrossRef]
14. Young, R., Pailler, E., Billiot, F., Drusch, F., Barthelemy, A. et al. (2012). Circulating tumor cells in lung cancer. Acta Cytologica, 56(6), 655–660. https://doi.org/10.1159/000345182 [Google Scholar] [PubMed] [CrossRef]
15. Le Gal, K., Ibrahim, M. X., Wiel, C., Sayin, V. I., Akula, M. K. et al. (2015). Antioxidants can increase melanoma metastasis in mice. Science Translational Medicine, 7(308), 308re8. https://doi.org/10.1126/scitranslmed.aad3740 [Google Scholar] [PubMed] [CrossRef]
16. Lone, S. N., Nisar, S., Masoodi, T., Singh, M., Rizwan, A. et al. (2022). Liquid biopsy: A step closer to transform diagnosis, prognosis and future of cancer treatments. Molecular Cancer, 21(1), 79. https://doi.org/10.1186/s12943-022-01543-7 [Google Scholar] [PubMed] [CrossRef]
17. de Bono, J. S., Scher, H. I., Montgomery, R. B., Parker, C., Miller, M. C. et al. (2008). Circulating tumor cells predict survival benefit from treatment in metastatic castration-resistant prostate cancer. Clinical Cancer Research, 14(19), 6302–6309. https://doi.org/10.1158/1078-0432.CCR-08-0872 [Google Scholar] [PubMed] [CrossRef]
18. Smerage, J. B., Barlow, W. E., Hortobagyi, G. N., Winer, E. P., Leyland-Jones, B. et al. (2014). Circulating tumor cells and response to chemotherapy in metastatic breast cancer: SWOG S0500. Journal of Clinical Oncology, 32(31), 3483–3489. https://doi.org/10.1200/JCO.2014.56.2561 [Google Scholar] [PubMed] [CrossRef]
19. Ilie, M., Hofman, V., Long-Mira, E., Selva, E., Vignaud, J. M. et al. (2014). “Sentinel” circulating tumor cells allow early diagnosis of lung cancer in patients with chronic obstructive pulmonary disease. PLoS One, 9(10), e111597. https://doi.org/10.1371/journal.pone.0111597 [Google Scholar] [PubMed] [CrossRef]
20. Shi, J., Zhang, R., Li, J., Zhang, R. (2020). Size profile of cell-free DNA: A beacon guiding the practice and innovation of clinical testing. Theranostics, 10(11), 4737–4748. https://doi.org/10.7150/thno.42565 [Google Scholar] [PubMed] [CrossRef]
21. Ilie, M., Long, E., Hofman, V., Selva, E., Bonnetaud, C. et al. (2014). Clinical value of circulating endothelial cells and of soluble CD146 levels in patients undergoing surgery for non-small cell lung cancer. British Journal of Cancer, 110(5), 1236–1243. https://doi.org/10.1038/bjc.2014.11 [Google Scholar] [PubMed] [CrossRef]
22. Diehl, F., Schmidt, K., Choti, M. A., Romans, K., Goodman, S. et al. (2008). Circulating mutant DNA to assess tumor dynamics. Nature Medicine, 14(9), 985–990. https://doi.org/10.1038/nm.1789 [Google Scholar] [PubMed] [CrossRef]
23. Mouliere, F., Robert, B., Arnau Peyrotte, E., Del Rio, M., Ychou, M. et al. (2011). High fragmentation characterizes tumour-derived circulating DNA. PLoS One, 6(9), e23418. https://doi.org/10.1371/journal.pone.0023418 [Google Scholar] [PubMed] [CrossRef]
24. Kohler, C., Barekati, Z., Radpour, R., Zhong, X. Y. (2011). Cell-free DNA in the circulation as a potential cancer biomarker. Anticancer Research, 31(8), 2623–2628. [Google Scholar] [PubMed]
25. Pantel, K., Alix-Panabières, C. (2019). Liquid biopsy and minimal residual disease–latest advances and implications for cure. Nature Reviews Clinical Oncology, 16(7), 409–424. https://doi.org/10.1038/s41571-019-0187-3 [Google Scholar] [PubMed] [CrossRef]
26. Huang, Z. H., Li, L. H., Hua, D. (2006). Quantitative analysis of plasma circulating DNA at diagnosis and during follow-up of breast cancer patients. Cancer Letters, 243(1), 64–70. https://doi.org/10.1016/j.canlet.2005.11.027 [Google Scholar] [PubMed] [CrossRef]
27. Leon, S. A., Shapiro, B., Sklaroff, D. M., Yaros, M. J. (1977). Free DNA in the serum of cancer patients and the effect of therapy. Cancer Research, 37(3), 646–650. [Google Scholar] [PubMed]
28. Rothé, F., Silva, M. J., Venet, D., Campbell, C., Bradburry, I. et al. (2019). Circulating tumor DNA in HER2-amplified breast cancer: A translational research substudy of the NeoALTTO phase III trial. Clinical Cancer Research, 25(12), 3581–3588. https://doi.org/10.1158/1078-0432.CCR-18-2521 [Google Scholar] [PubMed] [CrossRef]
29. Cristofanilli, M., Budd, G. T., Ellis, M. J., Stopeck, A., Matera, J. et al. (2004). Circulating tumor cells, disease progression, and survival in metastatic breast cancer. The New England Journal of Medicine, 351(8), 781–791. https://doi.org/10.1056/NEJMoa040766 [Google Scholar] [PubMed] [CrossRef]
30. Pierga, J. Y., Hajage, D., Bachelot, T., Delaloge, S., Brain, E. et al. (2012). High independent prognostic and predictive value of circulating tumor cells compared with serum tumor markers in a large prospective trial in first-line chemotherapy for metastatic breast cancer patients. Annals of Oncology, 23(3), 618–624. https://doi.org/10.1093/annonc/mdr263 [Google Scholar] [PubMed] [CrossRef]
31. Zhou, Y., Xu, Y., Gong, Y., Zhang, Y., Lu, Y. et al. (2019). Clinical factors associated with circulating tumor DNA (ctDNA) in primary breast cancer. Molecular Oncology, 13(5), 1033–1046. https://doi.org/10.1002/1878-0261.12456 [Google Scholar] [PubMed] [CrossRef]
32. Catarino, R., Ferreira, M. M., Rodrigues, H., Coelho, A., Nogal, A. et al. (2008). Quantification of free circulating tumor DNA as a diagnostic marker for breast cancer. DNA and Cell Biology, 27(8), 415–421. https://doi.org/10.1089/dna.2008.0744 [Google Scholar] [PubMed] [CrossRef]
33. Shao, X., He, Y., Ji, M., Chen, X., Qi, J. et al. (2015). Quantitative analysis of cell-free DNA in ovarian cancer. Oncology Letters, 10(6), 3478–3482. https://doi.org/10.3892/ol.2015.3771 [Google Scholar] [PubMed] [CrossRef]
34. Herrera, L. J., Raja, S., Gooding, W. E., El-Hefnawy, T., Kelly, L. et al. (2005). Quantitative analysis of circulating plasma DNA as a tumor marker in thoracic malignancies. Clinical Chemistry, 51(1), 113–118. https://doi.org/10.1373/clinchem.2004.039263 [Google Scholar] [PubMed] [CrossRef]
35. Board, R. E., Wardley, A. M., Dixon, J. M., Armstrong, A. C., Howell, S. et al. (2010). Detection of PIK3CA mutations in circulating free DNA in patients with breast cancer. Breast Cancer Research and Treatment, 120(2), 461–467. https://doi.org/10.1007/s10549-010-0747-9 [Google Scholar] [PubMed] [CrossRef]
36. Bettegowda, C., Sausen, M., Leary, R. J., Kinde, I., Wang, Y. et al. (2014). Detection of circulating tumor DNA in early- and late-stage human malignancies. Science Translational Medicine, 6(224), 224ra24. https://doi.org/10.1126/scitranslmed.3007094 [Google Scholar] [PubMed] [CrossRef]
37. Fribbens, C., O’Leary, B., Kilburn, L., Hrebien, S., Garcia-Murillas, I. et al. (2016). Plasma ESR1 mutations and the treatment of estrogen receptor-positive advanced breast cancer. Journal of Clinical Oncology, 34(25), 2961–2968. https://doi.org/10.1200/JCO.2016.67.3061 [Google Scholar] [PubMed] [CrossRef]
38. Chu, D., Paoletti, C., Gersch, C., VanDenBerg, D. A., Zabransky, D. J. et al. (2016). ESR1 mutations in circulating plasma tumor DNA from metastatic breast cancer patients ESR1 mutations detected in circulating tumor DNA. Clinical Cancer Research, 22, 993–999. https://doi.org/10.1158/1078-0432.CCR-15-0943 [Google Scholar] [PubMed] [CrossRef]
39. Zhang, H., Lin, X., Huang, Y., Wang, M., Cen, C. et al. (2021). Detection methods and clinical applications of circulating tumor cells in breast cancer. Frontiers in Oncology, 11, 652253. https://doi.org/10.3389/fonc.2021.652253 [Google Scholar] [PubMed] [CrossRef]
40. Safarpour, H., Dehghani, S., Nosrati, R., Zebardast, N., Alibolandi, M. et al. (2020). Optical and electrochemical-based nano-aptasensing approaches for the detection of circulating tumor cells (CTCs). Biosensors and Bioelectronics, 148, 111833. https://doi.org/10.1016/j.bios.2019.111833 [Google Scholar] [PubMed] [CrossRef]
41. Lopes, C., Piairo, P., Chícharo, A., Abalde-Cela, S., Pires, L. R. et al. (2021). HER2 expression in circulating tumour cells isolated from metastatic breast cancer patients using a size-based microfluidic device. Cancers, 13(17), 4446. https://doi.org/10.3390/cancers13174446 [Google Scholar] [PubMed] [CrossRef]
42. Sharma, S., Zhuang, R., Long, M., Pavlovic, M., Kang, Y. et al. (2018). Circulating tumor cell isolation, culture, and downstream molecular analysis. Biotechnology Advances, 36(4), 1063–1078. https://doi.org/10.1016/j.biotechadv.2018.03.007 [Google Scholar] [PubMed] [CrossRef]
43. Reduzzi, C., di Cosimo, S., Gerratana, L., Motta, R., Martinetti, A. et al. (2021). Circulating tumor cell clusters are frequently detected in women with early-stage breast cancer. Cancers, 13(10), 2356. https://doi.org/10.3390/cancers13102356 [Google Scholar] [PubMed] [CrossRef]
44. Kong, S. L., Liu, X., Tan, S. J., Tai, J. A., Phua, L. Y. et al. (2021). Complementary sequential circulating tumor cell (CTC) and cell-free tumor DNA (ctDNA) profiling reveals metastatic heterogeneity and genomic changes in lung cancer and breast cancer. Frontiers in Oncology, 11, 698551. https://doi.org/10.3389/fonc.2021.698551 [Google Scholar] [PubMed] [CrossRef]
45. Xie, N., Hu, Z., Tian, C., Xiao, H., Liu, L. et al. (2020). In vivo detection of CTC and CTC plakoglobin status helps predict prognosis in patients with metastatic breast cancer. Pathology Oncology Research, 26(4), 2435–2442. https://doi.org/10.1007/s12253-020-00847-7 [Google Scholar] [PubMed] [CrossRef]
46. Krol, I., Schwab, F. D., Carbone, R., Ritter, M., Picocci, S. et al. (2021). Detection of clustered circulating tumour cells in early breast cancer. British Journal of Cancer, 125(1), 23–27. https://doi.org/10.1038/s41416-021-01327-8 [Google Scholar] [PubMed] [CrossRef]
47. Rossi, T., Gallerani, G., Angeli, D., Cocchi, C., Bandini, E. et al. (2020). Single-cell NGS-based analysis of copy number alterations reveals new insights in circulating tumor cells persistence in early-stage breast cancer. Cancers, 12(9), 2490. https://doi.org/10.3390/cancers12092490 [Google Scholar] [PubMed] [CrossRef]
48. Liu, C., Liu, Y., Xu, X. X., Wu, H., Xie, H. G. et al. (2015). Potential effect of matrix stiffness on the enrichment of tumor initiating cells under three-dimensional culture conditions. Experimental Cell Research, 330(1), 123–134. https://doi.org/10.1016/j.yexcr.2014.07.036 [Google Scholar] [PubMed] [CrossRef]
49. Paoletti, C., Miao, J., Dolce, E. M., Darga, E. P., Repollet, M. I. et al. (2019). Circulating tumor cell clusters in patients with metastatic breast cancer: A SWOG S0500 translational medicine study. Clinical Cancer Research, 25(20), 6089–6097. https://doi.org/10.1158/1078-0432.ccr-19-0208 [Google Scholar] [PubMed] [CrossRef]
50. Zhao, P., Zhou, W., Liu, C., Zhang, H., Cheng, Z. et al. (2019). Establishment and characterization of a CTC cell line from peripheral blood of breast cancer patient. Journal of Cancer, 10(24), 6095–6104. https://doi.org/10.7150/jca.33157 [Google Scholar] [PubMed] [CrossRef]
51. Stefanovic, S., Deutsch, T. M., Riethdorf, S., Fischer, C., Hartkopf, A. et al. (2020). The lack of evidence for an association between cancer biomarker conversion patterns and CTC-status in patients with metastatic breast cancer. International Journal of Molecular Sciences, 21(6), 2161. https://doi.org/10.3390/ijms21062161 [Google Scholar] [PubMed] [CrossRef]
52. Deutsch, T. M., Riethdorf, S., Fremd, C., Feisst, M., Nees, J. et al. (2020). HER2-targeted therapy influences CTC status in metastatic breast cancer. Breast Cancer Research and Treatment, 182(1), 127–136. https://doi.org/10.1007/s10549-020-05687-2 [Google Scholar] [PubMed] [CrossRef]
53. Forshew, T., Murtaza, M., Parkinson, C., Gale, D., Tsui, D. W. et al. (2012). Noninvasive identification and monitoring of cancer mutations by targeted deep sequencing of plasma DNA. Science Translational Medicine, 4(136), 136ra68. https://doi.org/10.1126/scitranslmed.3003726 [Google Scholar] [PubMed] [CrossRef]
54. Huang, K., Fan, G. (2010). DNA methylation in cell differentiation and reprogramming: An emerging systematic view. Regenerative Medicine, 5(4), 531–544. https://doi.org/10.2217/rme.10.35 [Google Scholar] [PubMed] [CrossRef]
55. Lin, J., Su, G., Su, W., Zhou, C. (2017). Progress in digital PCR technology and application. Sheng Wu Gong Cheng Xue Bao = Chinese Journal of Biotechnology, 33(2), 170–177. https://doi.org/10.13345/j.cjb.160269 [Google Scholar] [PubMed] [CrossRef]
56. Mao, X., Liu, C., Tong, H., Chen, Y., Liu, K. (2019). Principles of digital PCR and its applications in current obstetrical and gynecological diseases. American Journal of Translational Research, 11(12), 7209–7222. [Google Scholar] [PubMed]
57. Li, M., Diehl, F., Dressman, D., Vogelstein, B., Kinzler, K. W. (2006). BEAMing up for detection and quantification of rare sequence variants. Nature Methods, 3(2), 95–97. https://doi.org/10.1038/nmeth850 [Google Scholar] [PubMed] [CrossRef]
58. Diehl, F., Li, M., He, Y., Kinzler, K. W., Vogelstein, B. et al. (2006). BEAMing: Single-molecule PCR on microparticles in water-in-oil emulsions. Nature Methods, 3(7), 551–559. https://doi.org/10.1038/nmeth898 [Google Scholar] [PubMed] [CrossRef]
59. Elazezy, M., Joosse, S. A. (2018). Techniques of using circulating tumor DNA as a liquid biopsy component in cancer management. Computational and Structural Biotechnology Journal, 16, 370–378. https://doi.org/10.1016/j.csbj.2018.10.002 [Google Scholar] [PubMed] [CrossRef]
60. Newman, A. M., Bratman, S. V., To, J., Wynne, J. F., Eclov, N. C. W. et al. (2014). An ultrasensitive method for quantitating circulating tumor DNA with broad patient coverage. Nature Medicine, 20(5), 548–554. https://doi.org/10.1038/nm.3519 [Google Scholar] [PubMed] [CrossRef]
61. Brinkman, A. B., Nik-Zainal, S., Simmer, F., Rodríguez-González, F. G., Smid, M. et al. (2019). Partially methylated domains are hypervariable in breast cancer and fuel widespread CpG island hypermethylation. Nature Communications, 10(1), 1749. https://doi.org/10.1038/s41467-019-09828-0 [Google Scholar] [PubMed] [CrossRef]
62. Deng, W., Murugan, S., Lindberg, J., Chellappa, V., Shen, X. et al. (2022). Fusion gene detection using whole-exome sequencing data in cancer patients. Frontiers in Genetics, 13, 820493. https://doi.org/10.3389/fgene.2022.820493 [Google Scholar] [PubMed] [CrossRef]
63. CDC (2022). Whole genome sequencing. PulseNet Methods & Protocols: CDC. [Google Scholar]
64. Buono, G., Gerratana, L., Bulfoni, M., Provinciali, N., Basile, D. et al. (2019). Circulating tumor DNA analysis in breast cancer: Is it ready for prime-time? Cancer Treatment Reviews, 73, 73–83. https://doi.org/10.1016/j.ctrv.2019.01.004 [Google Scholar] [PubMed] [CrossRef]
65. Nikanjam, M., Kato, S., Kurzrock, R. (2022). Liquid biopsy: Current technology and clinical applications. Journal of Hematology & Oncology, 15(1), 131. https://doi.org/10.1186/s13045-022-01351-y [Google Scholar] [PubMed] [CrossRef]
66. Li, H., Jing, C., Wu, J., Ni, J., Sha, H. et al. (2019). Circulating tumor DNA detection: A potential tool for colorectal cancer management. Oncology Letters, 17(2), 1409–1416. https://doi.org/10.3892/ol.2018.9794 [Google Scholar] [PubMed] [CrossRef]
67. Kinugasa, H., Nouso, K., Tanaka, T., Miyahara, K., Morimoto, Y. et al. (2015). Droplet digital PCR measurement of HER2 in patients with gastric cancer. British Journal of Cancer, 112(10), 1652–1655. https://doi.org/10.1038/bjc.2015.129 [Google Scholar] [PubMed] [CrossRef]
68. Ma, Z. Y., Chan, C. S. Y., Lau, K. S., Ng, L., Cheng, Y. Y. et al. (2021). Application of droplet digital polymerase chain reaction of plasma methylated septin 9 on detection and early monitoring of colorectal cancer. Scientific Reports, 11(1), 23446. https://doi.org/10.1038/s41598-021-02879-8 [Google Scholar] [PubMed] [CrossRef]
69. Zhu, Y., Lu, D., Lira, M. E., Xu, Q., Du, Y. et al. (2016). Droplet digital polymerase chain reaction detection of HER2 amplification in formalin fixed paraffin embedded breast and gastric carcinoma samples. Experimental and Molecular Pathology, 100(2), 287–293. https://doi.org/10.1016/j.yexmp.2015.11.027 [Google Scholar] [PubMed] [CrossRef]
70. Chen, X., Wang, L., Lou, J. (2020). Nanotechnology strategies for the analysis of circulating tumor DNA: A review. Medical Science Monitor, 26, e921040. https://doi.org/10.12659/MSM.921040 [Google Scholar] [PubMed] [CrossRef]
71. Gale, D., Lawson, A. R. J., Howarth, K., Madi, M., Durham, B. et al. (2018). Development of a highly sensitive liquid biopsy platform to detect clinically-relevant cancer mutations at low allele fractions in cell-free DNA. PLoS One, 13(3), e0194630. https://doi.org/10.1371/journal.pone.0194630 [Google Scholar] [PubMed] [CrossRef]
72. Newman, A. M., Lovejoy, A. F., Klass, D. M., Kurtz, D. M., Chabon, J. J. et al. (2016). Integrated digital error suppression for improved detection of circulating tumor DNA. Nature Biotechnology, 34(5), 547–555. https://doi.org/10.1038/nbt.3520 [Google Scholar] [PubMed] [CrossRef]
73. Przybyl, J., Chabon, J. J., Spans, L., Ganjoo, K. N., Vennam, S. et al. (2018). Combination approach for detecting different types of alterations in circulating tumor DNA in leiomyosarcoma. Clinical Cancer Research, 24(11), 2688–2699. https://doi.org/10.1158/1078-0432.CCR-17-3704 [Google Scholar] [PubMed] [CrossRef]
74. Scherer, F., Kurtz, D. M., Newman, A. M., Stehr, H., Craig, A. F. et al. (2016). Distinct biological subtypes and patterns of genome evolution in lymphoma revealed by circulating tumor DNA. Science Translational Medicine, 8(364), 364ra155. https://doi.org/10.1126/scitranslmed.aai8545 [Google Scholar] [PubMed] [CrossRef]
75. Olova, N., Krueger, F., Andrews, S., Oxley, D., Berrens, R. V. et al. (2018). Comparison of whole-genome bisulfite sequencing library preparation strategies identifies sources of biases affecting DNA methylation data. Genome Biology, 19(1), 33. https://doi.org/10.1186/s13059-018-1408-2 [Google Scholar] [PubMed] [CrossRef]
76. Imperial, R., Nazer, M., Ahmed, Z., Kam, A. E., Pluard, T. J. et al. (2019). Matched whole-genome sequencing (WGS) and whole-exome sequencing (WES) of tumor tissue with circulating tumor DNA (ctDNA) analysis: Complementary modalities in clinical practice. Cancers, 11(9), 1399. https://doi.org/10.3390/cancers11091399 [Google Scholar] [PubMed] [CrossRef]
77. Sun, Y., Liu, F., Fan, C., Wang, Y., Song, L. et al. (2021). Characterizing sensitivity and coverage of clinical WGS as a diagnostic test for genetic disorders. BMC Medical Genomics, 14(1), 102. https://doi.org/10.1186/s12920-021-00948-5 [Google Scholar] [PubMed] [CrossRef]
78. Davies, S. (2017). Annual report of the chief medical officer 2016, generation genome. London: Department of Health. [Google Scholar]
79. Gautschi, O., Bigosch, C., Huegli, B., Jermann, M., Marx, A. et al. (2004). Circulating deoxyribonucleic acid as prognostic marker in non-small-cell lung cancer patients undergoing chemotherapy. Journal of Clinical Oncology, 22(20), 4157–4164. https://doi.org/10.1200/JCO.2004.11.123 [Google Scholar] [PubMed] [CrossRef]
80. Bettegowda, C., Sausen, M., Leary, R. J., Kinde, I., Wang, Y. et al. (2014). Detection of circulating tumor DNA in early-and late-stage human malignancies. Science Translational Medicine, 6(224), 224ra224. https://doi.org/10.1126/scitranslmed.3007094 [Google Scholar] [PubMed] [CrossRef]
81. Agassi, R., Czeiger, D., Shaked, G., Avriel, A., Sheynin, J. et al. (2015). Measurement of circulating cell-free DNA levels by a simple fluorescent test in patients with breast cancer. American Journal of Clinical Pathology, 143(1), 18–24. https://doi.org/10.1309/AJCPI5YHG0OGFAHM [Google Scholar] [PubMed] [CrossRef]
82. Roth, C., Pantel, K., Müller, V., Rack, B., Kasimir-Bauer, S. et al. (2011). Apoptosis-related deregulation of proteolytic activities and high serum levels of circulating nucleosomes and DNA in blood correlate with breast cancer progression. BMC Cancer, 11(1), 1–12. https://doi.org/10.1186/1471-2407-11-4 [Google Scholar] [PubMed] [CrossRef]
83. Gong, B., Xue, J., Yu, J., Li, H., Hu, H. et al. (2012). Cell-free DNA in blood is a potential diagnostic biomarker of breast cancer. Oncology Letters, 3(4), 897–900. https://doi.org/10.3892/ol.2012.576 [Google Scholar] [PubMed] [CrossRef]
84. Leary, R. J., Sausen, M., Kinde, I., Papadopoulos, N., Carpten, J. D. et al. (2012). Detection of chromosomal alterations in the circulation of cancer patients with whole-genome sequencing. Science Translational Medicine, 4(162), 162ra154. https://doi.org/10.1126/scitranslmed.3004742 [Google Scholar] [PubMed] [CrossRef]
85. Dawson, S. J., Tsui, D. W., Murtaza, M., Biggs, H., Rueda, O. M. et al. (2013). Analysis of circulating tumor DNA to monitor metastatic breast cancer. The New England Journal of Medicine, 368(13), 1199–1209. https://doi.org/10.1056/NEJMoa1213261 [Google Scholar] [PubMed] [CrossRef]
86. Rohanizadegan, M. (2018). Analysis of circulating tumor DNA in breast cancer as a diagnostic and prognostic biomarker. Cancer Genetics, 228–229, 159–168. https://doi.org/10.1016/j.cancergen.2018.02.002 [Google Scholar] [PubMed] [CrossRef]
87. Croessmann, S., Park, B. H. (2021). Circulating tumor DNA in early-stage breast cancer: New directions and potential clinical applications. Clinical Advances in Hematology & Oncology: H&O, 19(3), 155–161. [Google Scholar]
88. Sant, M., Bernat-Peguera, A., Felip, E., Margelí, M. (2022). Role of ctDNA in breast cancer. Cancers, 14(2), 310. https://doi.org/10.3390/cancers14020310 [Google Scholar] [PubMed] [CrossRef]
89. Bidard, F. C., Callens, C., Pistilli, B., Dalenc, F., de La Motte Rouge, T. et al. (2019). Emergence of ESR1 mutation in cell-free DNA during first line aromatase inhibitor and palbociclib: An exploratory analysis of the PADA-1 trial. Annals of Oncology, 30, v105–v106. https://doi.org/10.1093/annonc/mdz242.002 [Google Scholar] [CrossRef]
90. André, F., Hurvitz, S., Fasolo, A., Tseng, L. M., Jerusalem, G. et al. (2016). Molecular alterations and everolimus efficacy in human epidermal growth factor receptor 2-overexpressing metastatic breast cancers: Combined exploratory biomarker analysis from BOLERO-1 and BOLERO-3. Journal of Clinical Oncology, 34(18), 2115–2124. https://doi.org/10.1200/JCO.2015.63.9161 [Google Scholar] [PubMed] [CrossRef]
91. Berns, K., Horlings, H. M., Hennessy, B. T., Madiredjo, M., Hijmans, E. M. et al. (2007). A functional genetic approach identifies the PI3K pathway as a major determinant of trastuzumab resistance in breast cancer. Cancer Cell, 12(4), 395–402. https://doi.org/10.1016/j.ccr.2007.08.030 [Google Scholar] [PubMed] [CrossRef]
92. O’Leary, B., Hrebien, S., Morden, J. P., Beaney, M., Fribbens, C. et al. (2018). Early circulating tumor DNA dynamics and clonal selection with palbociclib and fulvestrant for breast cancer. Nature Communications, 9(1), 896. https://doi.org/10.1038/s41467-018-03215-x [Google Scholar] [PubMed] [CrossRef]
93. Turner, N. C., Kingston, B., Kilburn, L. S., Kernaghan, S., Wardley, A. M. et al. (2020). Circulating tumour DNA analysis to direct therapy in advanced breast cancer (plasmaMATCHA multicentre, multicohort, phase 2a, platform trial. Lancet Oncology, 21(10), 1296–1308. https://doi.org/10.1016/S1470-2045(20)30444-7 [Google Scholar] [PubMed] [CrossRef]
94. Lennon, A. M., Buchanan, A. H., Kinde, I., Warren, A., Honushefsky, A. et al. (2020). Feasibility of blood testing combined with PET-CT to screen for cancer and guide intervention. Science, 369(6499). https://doi.org/10.1126/science.abb9601 [Google Scholar] [PubMed] [CrossRef]
95. Cohen, J. D., Li, L., Wang, Y., Thoburn, C., Afsari, B. et al. (2018). Detection and localization of surgically resectable cancers with a multi-analyte blood test. Science, 359(6378), 926–930. https://doi.org/10.1126/science.aar3247 [Google Scholar] [PubMed] [CrossRef]
96. Wang, R., Li, X., Zhang, H., Wang, K., He, J. (2017). Cell-free circulating tumor DNA analysis for breast cancer and its clinical utilization as a biomarker. Oncotarget, 8(43), 75742–75755. https://doi.org/10.18632/oncotarget.20608 [Google Scholar] [PubMed] [CrossRef]
97. Heidary, M., Auer, M., Ulz, P., Heitzer, E., Petru, E. et al. (2014). The dynamic range of circulating tumor DNA in metastatic breast cancer. Breast Cancer Research, 16(4), 421. https://doi.org/10.1186/s13058-014-0421-y [Google Scholar] [PubMed] [CrossRef]
98. Liu, B., Hu, Z., Ran, J., Xie, N., Tian, C. et al. (2022). The circulating tumor DNA (ctDNA) alteration level predicts therapeutic response in metastatic breast cancer: Novel prognostic indexes based on ctDNA. The Breast, 65, 116–123. https://doi.org/10.1016/j.breast.2022.07.010 [Google Scholar] [PubMed] [CrossRef]
Cite This Article
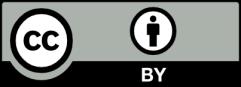
This work is licensed under a Creative Commons Attribution 4.0 International License , which permits unrestricted use, distribution, and reproduction in any medium, provided the original work is properly cited.