Open Access
REVIEW
Regulation of pathological blood-brain barrier for intracranial enhanced drug delivery and anti-glioblastoma therapeutics
1 Key Laboratory of Prevention and Treatment of Cardiovascular and Cerebrovascular Diseases of Ministry of Education, Gannan Medical University, Ganzhou, 341000, China
2 College of Pharmaceutical Sciences, Zhejiang University, Hangzhou, 310058, China
3 Department of Orthopedics, First Affiliated Hospital of Gannan Medical University, Ganzhou, 341000, China
4 Medical College of Soochow University, Suzhou, 215123, China
5 Department of Infectious Diseases, Ganzhou People’s Hospital, Ganzhou, 341000, China
6 Ganzhou Key Laboratory of Neuroinflammation Research, Gannan Medical University, Ganzhou, 341000, China
* Corresponding Author: FUQIANG HU. Email: ; LIJUAN WEN. Email: Lijuan Wen
# These authors contributed equally to this work
Oncology Research 2021, 29(5), 351-363. https://doi.org/10.32604/or.2022.025696
Received 20 July 2022; Accepted 17 September 2022; Issue published 10 October 2022
Abstract
The blood-brain barrier (BBB) is an essential component in regulating and maintaining the homeostatic microenvironment of the central nervous system (CNS). During the occurrence and development of glioblastoma (GBM), BBB is pathologically destroyed with a marked increase in permeability. Due to the obstruction of the BBB, current strategies for GBM therapeutics still obtain a meager success rate and may lead to systemic toxicity. Moreover, chemotherapy could promote pathological BBB functional restoration, which results in significantly reduced intracerebral transport of therapeutic agents during multiple administrations of GBM and the eventual failure of GBM chemotherapy. The effective delivery of intracerebral drugs still faces severe challenges. However, strategies that regulate the pathological BBB to enhance the transport of therapeutic agents across the barrier may provide new opportunities for the effective and safe treatment of GBM. This article reviews the structure and function of BBB in physiological states, the mechanisms underlying BBB pathological fenestration during the development of GBM, and the therapeutic strategies of GBM based on BBB intervention and medicinal drugs transporting across the BBB.Keywords
The leading cause for the failure of tumor chemotherapy is the lack of effective drug delivery in the lesion site, which is still a severe challenge for glioblastoma (GBM) therapy [1,2]. The increase in tumor resistance to drugs primarily lies in the use of low-specific and highly cytotoxic therapeutics and fluctuations in plasma drug levels. The blood-brain barrier (BBB) is a specialized endothelial structure that is partially covered by pericytes and almost entirely surrounded by the end feet of astrocytes. The primary function of BBB is to maintain the normal physiological part of the brain and the balance of the central nervous systems (CNS) environment [3,4]. It is noteworthy that the lack of fenestration in endothelial cells results in the low permeability of BBB, and tight junctions (TJs) between adjacent brain endothelial cells make the barrier 50–100 times tighter than peripheral microvessels. Except for some special transport channels, almost 100% biological macromolecules and 98% small molecules cannot cross the BBB into the brain, which seriously limits the efficacy of therapeutic agents for brain diseases, including GBM [5]. Therefore, the critical scientific problem to be urgently solved for treating GBM is to improve the transport efficiency of therapeutics into the brain effectively.
Recently, extensive and substantial progress has been made in GBM therapy, including brain receptor-mediated targeted therapy [6], focused ultrasound opening the BBB [7] and Borneol for “orifice-opening” of the BBB [8]. Among them, the preferred strategy for GBM-targeted treatment is receptor-mediated intracerebral transport which shares characteristics of high specificity, affinity and selectivity [9,10]. Several studies have described that many kinds of receptors are overexpressed on the BBB, including insulin receptors (IR) [11], low-density lipoprotein receptors (LDLR) [12], transferrin receptors (TfR) [13] and nicotinic acetylcholine receptors (nAChRs) [14]. And their ligands are usually employed to facilitate receptor-mediated intracerebral delivery of anti-GBM therapeutic drugs. However, the intracerebral transport efficiency of medications is still unsatisfactory. In the author’s crude opinion, some of these reasons are mainly attributed to the fact that current studies on brain-targeted drug delivery systems primarily focus on the interactions between ligands and their receptors, as well as targeted cells and targeted tissues, while ignoring the dynamic changes of BBB function during the development and treatment periods of brain diseases. One or even several treatments of the brain-targeted delivery system may achieve tumor-inhibiting effects in the short term. Still, in the long run, its impact on the restoration of pathological BBB can continue to inhibit the intracerebral delivery efficiency of therapeutic drugs, and lead to the failure of their treatment. In addition, the poor druggability of the vast majority of brain-targeted drug delivery systems limited their clinical application. Therefore, there is still a long way to find safe and effective ways to go to treat brain diseases, and anti-GBM therapy still needs the discovery of new mechanisms, the breakthrough of new technologies and the emergence of new treatments.
The pathological process of GBM is likely to be closely related to BBB, which might also play a vital role in the occurrence and treatment of GBM [15]. Under physiological conditions, BBB is a biological membrane barrier composed of brain microvascular endothelial cells and their TJs, basement membranes and the end feet of glial cells around capillaries [16]. TJs structure is an essential morphological basis of the BBB, forming the low permeability of the barrier and its high resistance properties [17]. Under pathological conditions, the occurrences of brain diseases [18–21], including GBM, Parkinson’s disease (PD), cerebral ischemia and Alzheimer’s disease (AD), are usually accompanied by pathological impairment of BBB, with decreased TJs proteins and increased BBB permeability.
Studies have confirmed that BBB pathological fenestration provides an opening paracellular pathway for the transport of therapeutics across the BBB. Hu et al. have demonstrated that BBB pathological fenestration in GBM provided an extra paracellular route for angiopep-2 modified glycolipid-like nanoparticles transporting across the barrier in vitro and in vivo [22]. Nevertheless, during multiple dosing treatments for GBM, the first administration with brain-targeted nanotherapeutics would cause pathological BBB restoration, which resulted in poor intracerebral transport efficiency during re-administration and eventual treatment failure. Our previous study has confirmed that the treatment of targeted Ap-CSssSA/P nanoparticles could restore pathological BBB mainly via VEGF downstream signaling pathway inhibition. However, pathological BBB functional recovery seriously reduces the intracerebral transport efficiency of Ap-CSssSA/P nanoparticles, leading to a poor outcome in GBM therapy. Therefore, combined sequential treatment with functionalized Ap-CSssSA/P nanoparticles and BBB permeability regulator SC79, which could reversibly re-open the restored BBB, was proposed to treat GBM. And the strategy finally produced an observable and significantly enhanced anti-GBM effect in vivo, compared to single Ap-CSssSA/P nanoparticles treatment [23]. Hence, the regulation of pathological BBB for improving the transport of therapeutic agents across the BBB may provide new opportunities for GBM effective treatment.
Physiological Characteristics of the BBB
BBB is the most distinct structural difference between the peripheral and cerebral vasculatures, and the barrier achieves CNS homeostasis by strictly regulating molecules transport between brain parenchyma and blood [24]. The BBB is formed by brain endothelial cells and is characterized by extensive windowless TJs, cytoskeleton and adherens junctions (AJs) (Fig. 1A).
Figure 1: BBB structure under physiological and pathological conditions. (A) Under physiological conditions, the BBB is formed by specialized endothelial cells characterized by no fenestration and extensive TJs and AJs. Transmembrane proteins of TJs mainly include Claudins, Occludin and ZOs. TJs and AJs are generally linked to the actin cytoskeleton. (B) GBM growth promotes the over-secretion of VEGF, inflammatory cytokines, ROS and MMPs in the lesion area, which may cause down-regulated expression of TJs and AJs proteins and cytoskeleton disruption, ultimately leading to BBB disruption and pathological fenestration.
TJs, distributed at the apex of cerebral endothelial cells, are consist of a series of paralleled and interconnected chains of transmembrane and cytoplasmic proteins that resemble an intricate network [25]. It is often thought that the closed endothelial fissures of the TJs allow the continued formation of vascular structures. The molecular biology of the TJs is quite complicated, and there are mainly three proteins in TJs, including Claudins, Occludin and Zonula Occludens (ZOs) proteins. Claudins homotypically bind to other Claudin molecules in adjacent cerebral endothelial cells, generating the primary seal of TJs [26,27]. Among Claudins, Claudin-5 is usually considered a critical marker of TJs breakdown and BBB pathological fenestration. The growth of GBM reduces expression and alters the intracellular distribution of Claudin-5, which in turn lead to BBB functional disruption [28]. Occludin is not necessary for TJs formation, and its function is mainly an additional support structure for TJs regulation as indicated in the knockdown and knockout experiments [29,30]. The regular expression and localization of other junctional proteins, such as vascular endothelial cadherin, are well compensated for the loss of Occludin [31]. Submembranous TJ-related proteins, also known as peripheral occlusive domain proteins, like ZO proteins, are another component of TJs. They might be involved in forming scaffolds that connect TJs and cytoskeleton [32,33]. Moreover, other junctional adhesion molecules present in brain endothelial cells, including JAM-A, JAM-B and JAM-C, are also involved in the formation and maintenance of TJs [17,34], as well as in the establishment of cell polarity and the promotion of leukocytes migrating across endothelial junctions [16]. In addition, the brain endothelial cytoskeleton, composed of three critical elements: actin microfilaments, intermediate filaments and microtubules, is also vitally crucial in the integrity establishment of TJs [35].
Adherens junctions (AJs) are distributed beneath the TJs in the basal region of the lateral plasma membrane. AJs are mainly composed of transmembrane glycoproteins, whose primary function is to connect cytoplasmic proteins to the cytoskeleton and exert additional contractile structures between adjacent endothelial cells to maintain the stability of the BBB [32]. Moreover, AJs also participate in brain endothelial cell interactions, cell polarity initiation and paracellular permeability regulation [36].
Mechanisms of BBB Pathological Disruption in GBM
BBB breakdown is generally considered detrimental in most cases because it leads to an influx of partial blood components, including leukocytes, potentially neuroactive compounds, and water (edema). The leakage of harmful substances into the brain would also accelerate the deterioration of the disease, leading to a poor prognosis of the disease. Abnormal increase of vascular endothelial growth factor (VEGF), reactive oxygen species (ROS), inflammatory cytokines and matrix metalloproteinases (MMPs) during the growth period of GBM, could seriously cause BBB disruption, accompanied by TJs proteins and AJs proteins down-regulation and cytoskeleton damage (Fig. 1B).
Vascular endothelial growth factor
Recent data indicated that VEGF, an angiogenic growth factor known to be overexpressed in GBM, promoted the endocytosis of endothelial cell adhesion molecule VE-cadherin and the down-regulation of TJs proteins (including Claudin-5, Occludin, ZO-1), resulting in the disruption of BBB function and increased endothelial permeability [37,38]. Moreover, the exogenous VEGF treatment may also induce TJs structural fracture and Occludin loss, accompanied by the phosphorylation of Occludin Ser490, resulting in vascular impairment [39]. Yang et al. indicated that VEGF could increase the permeability of BBB in GBM by a paracellular pathway (down-regulation of Claudin-5 and Occludin) and a transcellular pathway (up-regulation of caveolin-1 and caveolin-2), and cGMP/PKG/NF-κB signaling pathway might also be involved in VEGF-mediated BBB disruption [40]. Inhibition of the VEGF/VEGFR signaling pathway promotes the restoration of pathological BBB, accompanied by increased expression of TJs, including Claudin-5 and Occludin, and cytoskeletal remodeling [23,41].
Proinflammatory status, causes oxidative stress and increases endothelial expression of cell adhesion molecules. ROS are generally overexpressed in tumor cells, including GBM cells, compared to healthy cells. Studies have shown that the VEGF secreted by GBM can activate its downstream signaling pathways, which cause the increase of nitric oxide (NO) release and ROS production and finally lead to the pathological disruption of BBB and the development of brain edema [42,43]. Elizabeth et al. proposed that the generation of ROS could enhance the tyrosine phosphorylation of VE-cadherin and β-catenin, which ultimately influences AJs integrity [44]. Gerty et al. further demonstrated that ROS regulates BBB integrity, characterized by rearrangement of cytoskeleton and disappearance of TJs proteins. And this process involves specific signaling pathways, including RhoA, PI3K kinase and PKB signaling [45].
In the regulation of BBB permeability, inflammatory mediators are known modulators. In most neuroinflammatory disease states, including GBM, compromised TJs, are prominent hallmark [46]. Magnetic resonance imaging (MRI) has fully verified that BBB disruption is associated with the development of vasogenic edema in patients with intracranial tumors [47]. Consistently, studies based on the GBM-bearing mice model indicate the down-regulation of TJs proteins (including Claudin-5, Occludin and ZO-1) in microvasculature may be induced by cytokines, such as interleukin-6 (IL-6), tumor necrosis factor-alpha (TNF-α), interferon-γ and NF-κB [48,49].
As previously mentioned, increased levels of MMPs have also been observed in GBM, with disrupted basal lamina proteins and degraded TJ complexes. Zinc-containing enzymes MMPs mediates the degradation of protein substrates mainly via Zn2+-mediated activation of the site-bound water molecules. The accumulation of zinc in microvessels of GBM could activate MMP-2 and MMP-9, producing the remodeling and degradation of TJs [50,51]. Suppression of MMP-2/9 using SB-3CT could partially alleviate the disruption of TJs [52].
In conclusion, the pathological process of GBM causes pathological fenestration of BBB, which is rooted in the destruction of TJs structure. Therefore, signaling pathways in which TJs proteins (Occludin, Claudins and ZOs) are involved may be potential targets for BBB intervention in treating GBM.
Targeting Pathological BBB for GBM Therapy
In general, water-soluble molecules are transported across the BBB by simple diffusion through the paracellular pathway [53]. Small-molecule lipid-soluble substances such as steroids and ethanol are transported into the brain through the transcellular diffusion pathway. In addition, the transport of substances across the BBB also includes absorptive-mediated transport (AMT), carrier-mediated transport (CMT), receptor-mediated transport (RMT), active efflux transport, and cell-mediated transport [54,55]. Among them, AMT has poor selectivity, and cationic protein can bind to any negatively charged cell membrane component. In addition, the use of cationic proteins may produce potential toxicity and immunogenicity. The transport of CMT is substrate selective, and only drug molecules structurally similar to endogenous carrier substrates can be captured and transported into the brain. Previous studies have confirmed that in anti-GBM therapy, RMT is considered one of the most mature strategies for intracerebral drug delivery [56–58]. Brain endothelial cells express many receptors, including TfR, IR, LDLR, scavenger receptor, acetylcholine receptor, leptin receptor, etc. Structural modification with corresponding ligands (such as transferrin, monoclonal antibody OX26, CDX, RVG29, TGN, angiopep-2, etc.) [59–61] can promote the transport of therapeutics across the BBB via the RMT pathway, offering the possibility for GBM-targeted therapy.
Cell-penetrating peptides (CPPs) are a class of short peptides with amino acids less than 30. They are extracted from insect, viral and mammalian proteins that can induce translocation of bioactive macromolecules to cross cell membranes without interacting with receptors [62]. They are generally classified into three categories based on their physical and chemical properties: cationic, amphiphilic, and hydrophobic. Cationic CPPs are mainly composed of positively charged amino acids, such as arginine and lysine, which can interact with the negatively charged plasma membrane [63]. The apical surface of brain capillaries is densely covered with negatively charged polysaccharide-protein complexes, making positively charged CPPs effective drug carriers for brain diseases passing through the BBB. However, the low tissue specificity, poor instability, and potential cytotoxicity of CPPs in vivo limit their application.
In recent years, a new class of targeted biomimetic drug delivery systems coated with biological membranes has attracted wide attention from researchers due to its immune escape and homotypic binding capacities. Various membranes have been taken for coating, such as erythrocyte [64], platelets [65], stem cells [66], tumor cells [67], bacteria [68], etc. By translocation of membrane onto the surface, these membrane-coated drug delivery systems integrate the biogenicity of membrane proteins and the chemical stability of synthetic drug delivery systems, with the advantages of good biocompatibility, low immunogenicity, and homologous targeting function at lesion sites [69,70]. Gboxin-loaded mesoporous silicon nanoparticles coated with a mixed membrane composed of erythrocyte and tumor cell membrane can effectively target glioma cells and inhibit tumor cell proliferation [71].
In addition, exosome-based technology has also been extensively studied as a means of delivering therapeutic drugs into the brain. As a kind of secretory vesicle, exosomes inherit some receptors on the surface of the cell membrane, possess the targeting ability of parental cells, and can cross a variety of biological barriers [72]. Using tumor-associated macrophage exosomes as a carrier to wrap thermosensitive liposomes could effectively target glioma cells, and photothermal combined with chemotherapy could reverse drug resistance of glioma [73]. Functionalized modification of exosomes with angiopep-2 to wrap the anti-tumor drug docetaxel can significantly enhance intracerebral drug delivery and improve its anti-glioma efficacy [74].
As a collection of natural and synthetic entities, membrane carriers show substantial therapeutic advantages and biocompatibility, a boon for researchers. However, each membrane has its advantages and limitations. For example, the erythrocyte membrane can prevent phagocytosis by the reticuloendothelial system (RES) and prolong the circulation time in vivo, but it lacks active targeting ability; leukocytes have homologous targeting capabilities, but are specific for certain tumors; platelets can target tumor and injured areas, but can also activate the immune system; cancer cells can attach homologously and aggressively, but have a short circulation time in the blood; stem cells have the ability to target tumors, but with low specificity; bacterial membranes have immune-inducing properties, but the process of membrane extraction is complicated due to the need to remove peptidoglycan [75–77]. Therefore, a fusion of two membranes can be effectively carried out to form a hybrid membrane with therapeutic benefits for both cells. Although significant achievements have been made in the research of membrane-coated nanoparticles, there are still some challenges to be solved that the level of translation from laboratories to clinical trials has not yet been fully developed, and more practical explorations are needed in the future.
Blood brain tumor barrier (BBTB) targeting
The structure of BBTB is similar to that of BBB, and the barrier is located between GBM tissue and microvessels formed by brain microvascular endothelial cells. BBTB is composed of highly specialized endothelial cells, and restricts the paracellular transport of most hydrophilic molecules to tumor tissues. With the development of GBM, tumor cells invade the surrounding normal brain tissue, and when tumor volume is >2 mm3, BBB function and integrity are destroyed, and BBTB begins to appear. The formation of BBTB is critical for the supply of oxygen and nutrients to tumor cells’ growth [78]. Moreover, with the emergence of BBTB, GBM cells can migrate along the newly formed blood vessels to surrounding healthy brain tissue. It has been shown that efflux proteins such as P-gp, MRP1, MRP3, and BCRP overexpressed in TJs and BBTB severely limit the effective delivery of therapeutic drugs to GBM lesions [79]. Hence, the use of receptors highly expressed on BBTB, such as epidermal growth factor receptor (EGFR) and integrin receptor, may become one of the effective strategies for GBM targeted therapy.
Opening Pathological BBB for GBM Therapy
BBB permeability also exhibits high variability in different areas of the same tumor, as BBB integrity often exhibits high heterogeneity within tumor tissue. In the core of the cancer, the permeability of BBB is generally the strongest, while the barrier structure remains relatively intact in peripheral regions of the cancer. Moreover, the BBB of the peripheral GBM still exhibits highly functional. The intact BBB of tumor-infiltrating areas severely limits the effective delivery of chemotherapeutic drugs to GBM cells, and is a critical factor leading to the inefficiency of GBM treatment and high recurrence rate. Therefore, an essential strategy for the effective treatment of GBM is to increase the permeability of BBB and promote the intracerebral delivery of therapeutic drugs. Moreover, BBB pathological fenestration in GBM could provide an additional paracellular pathway for active targeting therapeutics transporting into the brain (Fig. 2B) [80–82]. At the same time, chemotherapy could promote the functional recovery of pathological BBB. Seriously, in the course of GBM multiple administration, the restoration of pathological BBB further limits the transport of intracerebral therapeutics, making anti-GBM therapy fail (Fig. 2C). Therefore, there has been much interest in seeking methods to further enhance endothelial permeability for biomedical and (nano-) pharmaceutics across the BBB in anti-GBM therapy (Fig. 2D). Here we reviewed three types of strategies opening pathological BBB for enhanced drug delivery in the brain (Fig. 3).
Figure 2: GBM therapy under the pathological state of the BBB. (A) Under physiological conditions, targeted therapeutics cross the BBB mainly by receptor-mediated pathway. (B) GBM growth causes BBB disruption and pathological fenestration, which in turn providing an extra paracellular way for therapeutics transporting into the brain. (C) Chemotherapy promotes pathological BBB functional recovery, which induces a significant decrease in targeted therapeutics transport across the BBB. (D) Opening of pathological BBB or re-opening of functional recovering pathological BBB via external stimulation, including biological stimuli, chemical stimuli, physical stimuli and signaling pathway regulators, could increase the intracranial delivery of targeted therapeutics for enhanced GBM therapy.
Figure 3: Mechanism of opening pathological BBB or re-opening functional recovering pathological BBB for enhanced drug delivery in the brain. Under biological stimuli, physical stimuli and chemical stimuli, (A) signaling pathways involved in the regulation of pathological BBB permeability and (B) transport routes of therapeutics across the BBB.
The virus is a kind of biostimulant material that can open TJs via modulating chemokines as precursors for inflammatory cell infiltration in the CNS [83]. Verma et al. indicated that the West Nile virus exhibited the ability to cross the BBB via the “Trojan horse” mechanism by enhancing the expression of cell adhesion molecules, which allows macrophages to migrate through the BBB without disrupting the BBB [84]. Foust et al. also verified that adeno-associated virus (AAV9) exhibits practical ability to effectively target CNS cells, including neurons and adult astrocytes, without disrupting BBB integrity [85]. Rabies virus (RABV) is a highly neurotropic virus, the RABV glycoprotein expressed in RABV can specifically bind to nAchR on the surface of neuron cells to realize the intracerebral transport of the virus [70,86,87]. The unique properties of the virus crossing the BBB have led to a wide range of targeted therapies for brain diseases. Zhang et al. used recombinant adeno-associated virus rAAV as a carrier to introduce the single-chain antibody scFV targeting abnormal phosphorylated Tau protein (P-Tau) into the brain, successfully inducing the continuous expression of scFV in vivo for AD immunotherapy [88]. Qiao et al. used RABV as a biological carrier to wrap virus-like nanoparticles, which could effectively overcome BBB, finally realizing GBM targeting and safe treatment [89].
Although biological viruses can reach the brain in a reasonable and precise way, there are still some inherent security issues in the use of virus-mediated drug delivery systems. In addition, the existing virus-like biomimetic nanocarriers mostly focus on the simulation of specific viral proteins, while ignoring the physical and chemical properties of viruses, leading to low drug delivery efficiency and poor therapeutic efficacy. Therefore, the construction of biomimetic nanocarriers that are highly similar in structure, shape and function to the virus may be one of the most effective ways to target delivery therapeutics for brain diseases treatment in the future.
Mannitol, an osmotic agent commonly explored, could shrink the endothelial cells line CNS capillaries with resultant separation of the endothelial TJs, which finally promotes passive diffusion of large molecules transport through the BBB. It has been used in combination with peptides, nanoparticles and gene delivery. Nevertheless, although mannitol therapy exhibits its beneficial effects on BBB opening, but there are still some risk factors, including brain damage, altered glucose uptake, the passage of plasma proteins and abnormal neuronal function [90,91]. Notably, mannitol produces non-selective disruption of the BBB, and only a 25% increase in the permeability of tumor microvasculature.
Bradykinin is a 9-peptide substance with a cardioprotective effect by reducing the infarct area of acute ischemia-reperfusion myocardium. Although the endogenous bradykinin may achieve some advances in regulating the permeability of BBB in animal studies, some drawbacks limit its application as a BBB permeability regulator, such as the short half-life (only several seconds), potent vasoactive metabolites [92], weak BBB opening effect. Therefore, the selective B2 bradykinin agonist Cereport (labradimil or RMP-7) has also been designed and investigated as potential inducer for BBB transient disruption. RMP-7 can cause intracellular Ca2+ influx by selectively binding to B2 receptors on the membrane of cerebral endothelial cells, which triggers a series of signal transmission reactions, including phosphatidylinositol, endothelial NOS and cGMP. The main mechanisms of RMP-7 in opening BBB is to increase the transport of endocytic vesicles or open the TJs of endothelial cells [93,94]. And it exhibits specific time-dependent and dose-dependent actions on human cerebral microvascular endothelial cells. Either intracarotid or i.v. administration with RMP-7 could produce an effect on BBB opening. Since RMP-7 cannot suppress the P-gp efflux pump, RMP-7 stimulation shows no effect on drugs that are P-gp substrates (such as paclitaxel) transported across the BBB.
Alkylglycerols can also be employed for BBB opening. The extent of disruption in the BBB mainly depends on the number and length of glycerols and alky groups presented in the chemical structure, respectively. Hulper et al. indicated that 1-O-pentylglycerol and 2-O-hexyldiglycerol exhibited the ability to reversibly increase the permeability of BBB, without any influence on the TJs strand complexity [95,96]. The phenomena could be due to protein rearrangement and alterations in the shape of the cells, and the cytoskeleton remodeling under the invention of alkylglycerols.
There are a series of traditional Chinese medicine (TCM) used explicitly for resuscitation purposes to restore consciousness in certain emergency conditions, such as coma, stroke, heart attack and brain-related traumatic brain injury etc. TCM resuscitation agents are mainly derived from aromatic minerals and animal materials, and are known as aromatic ‘orifice-opening’ agents. Borneol is a bicyclic monoterpene with fragrant odor, pungent and highly hydrophobic, which is a representative resuscitation agent in TCM that has been used in clinical practice for more than 1500 years. Increasing evidence prove that Borneol is an effective adjuvant that can modulate BBB permeability and promote drug delivery into the brain. The enhanced permeability induced by Borneol is closely associated with activating adenosine receptors, the down-regulation of TJs, the increase of void structures between the endothelial cells and the suppression of efflux protein function [97,98]. Moreover, systemic Borneol was found to modulate the vasodilatory neurotransmitters such as histamine and serotonin in the hypothalamus [99]. The activation of these neurotransmitters can induce cerebral vasodilation and enhance the permeability of BBB through nitric oxide and its receptors distributed on endothelial cells and astrocytes. However, current research is mainly limited to studies in cell lines or rodent-based models. There is still a lack of research data on permeation enhancing effect of Borneol in higher animal models such as pigs and primates. And the efficacy and safety of Borneol still need to be further studied.
Adenosine, a purine nucleoside produced by many organs (heart, lung, gut, brain) and immune cells throughout the body, acts as a cellular metabolic distress signal. Its primary function is to reduce tissue damage and promote repair through diverse receptor-mediated mechanisms, including increasing oxygen supply ratio to demand, protecting against ischemic injury, anti-inflammatory effects and stimulating angiogenesis [100,101]. The combination of adenosine and A2A adenosine receptor (AR) expressed on the BBB could temporarily and transiently increase intercellular spaces between the brain endothelial cells, with an up-regulation of BBB permeability [102]. Kim et al. indicated that the activation of AR with Lexiscan increases the permeability of a primary human BBB with an increased transmembrane transport of chemotherapeutic drugs and T cells. And the phenomenon was mainly due to the morphological changes in actin-cytoskeletal reorganization induced by RhoA signaling and a potent down-regulated expression of VE-cadherin and Claudin-5 [103]. Gao et al. developed a series of nanoagonists (NAs) for AR activation, and NAs increased the permeability of the endothelial cell monolayer through TJs disruption. In vivo imaging studies further indicated that the intravenous injection of NAs could remarkably increase brain uptake of the model drug and the BBB opening time in a range of 0.5–2.0 h. And the temporary opening of the BBB induced by NAs shows the promise to reduce potential risks like overdosage and uncontrollable BBB leakage [104].
5-phosphodiesterase inhibitors
5-phosphodiesterase inhibitors are the selective inhibitors of type 5 PDE (PDE5), such as sildenafil, vardenafil, and tadalafil, which can prevent phosphodiesterases (PDE) from reducing intracellular cGMP levels down to 5′-GMP. cGMP is a crucial intracellular second messenger, which could activate cGMP-dependent protein kinase (PKG) and stimulate KCa channels to increase vesicle trafficking in brain tumor microvasculature [105,106]. Thus, as an oral treatment for erectile dysfunction in men approved by FDA, oral 5-phosphodiesterase inhibitors cannot regulate tight junction integrity in tumor capillary endothelium, but can increase transendothelial vesicular density to enhance the penetration of chemotherapy into the brain [107]. In addition, 5-phosphodiesterase inhibitors may reduce the chaperone glucose-regulated protein (GRP78) and P-glycoprotein involved in chemoresistance in glioblastoma mice and inhibit microglial activation induced by lipopolysaccharide (LPS), which indicated they were excellent drugs that can be combined with chemotherapy drugs to treat brain tumors [108,109].
NOS-3 activators and NO donors
The nitric oxide synthase-3, (also called endothelial constitutive nitric oxide synthase, eNOS or ecNOS) was mainly expressed in endothelial cells and neurons. The principal physiological function of NOS-3 was to catalyze L-arginine to L-citrulline and produce NO with the participation of many cofactors, such as tetrahydrobiopterin (BH4) and calmodulin. Nitric oxide, as a biological messenger, was first identified as an endothelial cell relaxing factor and closely involved in mediating the regulation of the integrity of the BBB. On one hand, NO can activate soluble guanylate cyclase, resulting in increased intracellular cyclic GMP levels, further regulating the macromolecular transport into the brain microvessels [94]. For another, NO has an unpaired electron and readily reacts with H2O2 or proteins to form highly toxic peroxynitrite (ONOO−) and the S-nitrosylation proteins in some degenerative diseases or tumors [110]. Excessive ONOO− and the S-nitrosylation of proteins severely damaged target cells and inactivated target enzymes, further enhancing the opening of the pathological blood-brain barrier [110,111].
VEGF-A is described as VPF (vascular permeability factor), a key regulator of normal and pathological blood vessel growth of the VEGF family, and a potent protein that rapidly and instantaneously enhances the vascular permeability of an intact BBB [112]. It is widely acknowledged that VEGF-A possesses two major biological activities, one is to stimulate the proliferation of vascular endothelial cells, the other is to increase vascular permeability [113]. The increase in vascular permeability induced by VEGF-A is common in pathological angiogenesis areas, such as solid tumors, wounds, and chronic inflammation. Notably, although some inflammatory cytokines, such as histamine and bradykinin, can also induce increased vascular permeability, experimental evidence showed that vascular leakage caused by VEGF and inflammatory mediators occurs through different molecular processes [114]. Microvascular wall exposure to VEGF induces permeability with abnormally rapid kinetics and 50,000 times higher than histamine [115].
The mechanism of VEGF-A in the regulation of vascular permeability is mainly involved with several complex transmembrane transport processes [115]. VEGF-A could induce fenestrations for small solute leakage, and also cause the formation of caveolae, which assembled into vesiculovacuolar organelles (VVOs) for protein transmembrane transport. Leakage of larger proteins and extravasation of erythrocytes depends on the release of vascular endothelial (VE), which mediates adhesions by cadherin. Furthermore, VEGF-A phosphorylates VEGFR2 and activates downstream signaling pathways involved in permeability regulation process. VEGF regulates the production of endothelial nitric oxide synthetase (eNOS) by PI3K/AKT. Furthermore, the activation of VEGFR2 stimulated phospholipase C-γ(PLC), produced diacylglycerol (DAG) and PKC, which possibly activated the nonselective cation channels (e.g., TRPC6 or TRPC3) and induced calcium influx [116].
Ultrasound-facilitated opening
Focused ultrasound (FU) is emerging as a new strategy for localized, reversible, and safe BBB disruption to enhance therapeutics delivery to the brain [117]. However, due to the complex structure of the brain, FU is easily reflected by the skull, which significantly attenuates the ultrasonic energy, and brings new challenges to the use of ultrasound to open the BBB for the treatment of brain diseases. Therefore, FU is typically used with prefabricated microbubbles (FU-MBs) consisting of albumin or lipid core, which encapsulate a semi-solid gas (perfluorocarbon) and are confined in the vasculature. FU-MBs can realize the non-invasive, targeted and reversible opening BBB at a low sound pressure, with good repeatability and no permanent damage to brain tissue. At present, FU-MBs has been widely used in delivering nano drug delivery system, gene drugs and other small molecule drugs across the BBB [59,118,119]. The potential mechanisms for intracerebral transport induced by FU-MBs include transcytosis, partial opening of interendothelial clefts and TJs, transendothelial channel formation and fenestration, and free passage through the damaged endothelium [120,121]. However, the use of FU-MBs may be accompanied by the temperature increase of local target tissues, resulting in microvascular rupture and cerebral edema. In addition, the type and concentration of MBs would seriously affect the degree and duration of ultrasonic opening of the BBB. Therefore, optimizing ultrasound equipment, controlling ultrasound parameters (sound pressure, frequency, irradiation mode, etc.) and screening appropriate MBs doses have important guiding significance for FU-MBs to mediate BBB opening in the treatment of brain diseases and clinical translation.
Microwave is another physical stimulus to open BBB for enhancing drug delivery into the brain. Wang et al. found that microwave exposure could cause VEGF/FlK-1-ERK pathway activation and Occludin phosphorylation, accompanied by Occludin down-regulation and its interaction with ZO-1 [122]. In addition, the resultant brain temperature increase induced by sufficiently strong microwave energy may also produce increased BBB permeability. Indeed, previous study has reported that the rate head exposed to microwave frequencies (2.5–3.2 GHz) could make brain temperature rise with an enhanced permeability of HRP, Evans blue and sodium fluorescein to BBB, and the temperature measured above 40°C. When brain temperature cooled below 40°C, the BBB opening effect is ignorable, suggesting that the mechanism of BBB opening is also related to the thermal effect of microwaves [123,124]. Notably, the thermal effect induced by microwaves may also increase brain infections. Furthermore, long-term exposure to microwave radiation can easily cause damage to brain tissues, resulting in symptoms such as memory loss, insomnia, headaches and dreams. The related mechanisms remain unclear, possibly including neuronal degeneration, apoptosis and necrosis, mitochondria swelling and cavitation, reduction of Nissl bodies, BBB damage, synaptic structure and function of plastic damage and calcium overload [125]. For safety concerns, there are no relevant reports on the application of microwave-opening BBB in treating brain diseases.
The use of electromagnetic field (EMF) pulses can also increase BBB permeability. Qiu et al. reported that EMF pulses could regulate protein kinase C signaling and ZO-1 translocation, inducing BBB permeability to increase [18]. Experimental data in vitro further showed enhanced transportation of antiviral drugs across BBB with EMF stimuli, and the wave shape, frequency and amplitude of EMF directly affect the intracerebral delivery efficiency of drugs [126]. Do et al. also confirmed that applying an external EMF could significantly enhance the rate of nanoparticles across the BBB [127]. Nano-carrier combined with EMF enormously facilitates intracerebral delivery of therapeutic drugs and could have potential clinical application in treating brain tumors and other brain-related diseases.
So far, no effective treatments for brain diseases (AD, PD, stroke, etc.), including GBM. In addition to the complexity of these diseases, the main reason is the presence of the BBB. For decades, the delivery of drugs to the brain and spinal cord has been an exciting but exhausting research area due to the highly complex structure of the BBB. With the development of pharmaceutical technology, therapeutic pharmaceutics and methods aiming at the BBB are constantly being discovered. Controllably regulating BBB opening provides the possibility for the transport of medicinal drugs into the brain. Various drug transport systems (AMT, CMT, RMT) also show great potential in the transportation of biological macromolecules and small molecules across the BBB. The emergence of biomimetic nanotechnology makes the treatment of brain diseases safer and more efficient.
BBB dysfunction, often referred to as “BBB opening,” is likely to be the common feature of the progression of brain diseases, including GBM. One practical and rational way to design a novel drug delivery system for intracerebral therapeutics delivery is to study the molecular mechanism regulating BBB permeability, and further utilize the differences between physiological conditions and pathological conditions. Notably, the brain is a vital organ that governs all the life activities of the human body. It is also necessary to evaluate the safety, risk and benefit for patients when the new technologies or brain-targeted systems are used to increase BBB permeability, especially for some brain diseases requiring long-term drug treatment. Overall, in the design of an intracerebral drug delivery system, significantly to increase the penetration of drugs in the brain, it is necessary to consider the selectivity of drugs for the lesion site, minimizing the distribution in non-target tissues.
Acknowledgement: We thank Jin, X.Y. (Zhejiang University) for graphics technical support.
Author Contribution: The authors confirm contribution to the paper as follows: study conception and design: Wen, L.J. and Hu, F.Q.; data collection: Wang, K. and Zhang, F.T.; analysis and interpretation of results: Wen, C.L.; manuscript preparation: Wen, L.J., Wang, K., Zhang, F.T., Wen, C.L., Huang, Z.H., Hu, Z.H. and Zhang, Y.W. All authors reviewed the content and approved the final version of the manuscript.
Funding Statement: The research was supported by the National Natural Science Foundation of China (NSFC No. 82104101), Jiangxi Provincial Natural Science Foundation, China (Grant No. 20212BAB216003), the Project of Gannan Medical University (No. ZD201903) and Ph.D. Start-up Fund of Gannan Medical University (QD201908).
Conflicts of Interest: The authors declare that they have no conflicts of interest to report regarding the present study.
References
1. Adhikaree, J., Moreno-Vicente, J., Kaur, A. P., Jackson, A. M., Patel, P. M. (2020). Resistance mechanisms and barriers to successful immunotherapy for treating glioblastoma. Cells, 9(2), 263–283. DOI 10.3390/cells9020263. [Google Scholar] [CrossRef]
2. Shergalis, A., Bankhead, A., Luesakul, U., Muangsin, N., Neamati, N. (2018). Current challenges and opportunities in treating glioblastoma. Pharmacological Reviews, 70(3), 412–445. DOI 10.1124/pr.117.014944. [Google Scholar] [CrossRef]
3. Kadry, H., Noorani, B., Cucullo, L. (2020). A blood-brain barrier overview on structure, function, impairment, and biomarkers of integrity. Fluids and Barriers of the CNS, 17(1), 69–92. DOI 10.1186/s12987-020-00230-3. [Google Scholar] [CrossRef]
4. Sweeney, M., Zhen, Z., Montagne, A., Nelson, A. R., Zlokovic, B. V. (2019). Blood-brain barrier: From physiology to disease and back. Physiological Reviews, 99(1), 21–78. DOI 10.1152/physrev.00050.2017. [Google Scholar] [CrossRef]
5. Pandit, R., Chen, L., Gtz, J. (2019). The blood-brain barrier: Physiology and strategies for drug delivery. Advanced Drug Delivery Reviews, 165–166(Suppl. 3), 1–14. DOI 10.1016/j.addr.2019.11.009. [Google Scholar] [CrossRef]
6. Fan, R., Chuan, D., Hou, H., Chen, H. F., Han, B. et al. (2019). Development of a hybrid nanocarrier-recognizing tumor vasculature and penetrating the BBB for glioblastoma multi-targeting therapy. Nanoscale, 11(23), 11285–11304. DOI 10.1039/C9NR01320B. [Google Scholar] [CrossRef]
7. Brighi, C., Salimova, E., de Veer, M., Puttick, S., Egan, G. (2022). Translation of focused ultrasound for blood-brain barrier opening in glioma. Journal of Controlled Release, 345(Suppl. 4), 443–463. DOI 10.1016/j.jconrel.2022.03.035. [Google Scholar] [CrossRef]
8. Zhang, Q. L., Fu, B. M., Zhang, Z. J. (2017). Borneol, a novel agent that improves central nervous system drug delivery by enhancing blood-brain barrier permeability. Drug Delivery, 24(1), 1037–1044. DOI 10.1080/10717544.2017.1346002. [Google Scholar] [CrossRef]
9. Lajoie, J. M., Shusta, E. V. (2015). Targeting receptor-mediated transport for delivery of biologics across the blood-brain barrier. Annual Review of Pharmacology & Toxicology, 55(1), 613–631. DOI 10.1146/annurev-pharmtox-010814-124852. [Google Scholar] [CrossRef]
10. Xiao, G., Gan, L. S. (2013). Receptor-mediated endocytosis and brain delivery of therapeutic biologics. International Journal of Cell Biology, 2013(2), 703545–703558. DOI 10.1155/2013/703545. [Google Scholar] [CrossRef]
11. Zhang, W., Liu, Q. Y., Haqqani, A. S., Leclerc, S., Stanimirovic, D. B. (2020). Differential expression of receptors mediating receptor-mediated transcytosis (RMT) in brain microvessels, brain parenchyma and peripheral tissues of the mouse and the human. Fluids and Barriers of the CNS, 17(1), 47–63. DOI 10.1186/s12987-020-00209-0. [Google Scholar] [CrossRef]
12. Xie, B. S., Wang, X., Pan, Y. H., Jiang, G., Lin, Y. (2021). Apolipoprotein E, low-density lipoprotein receptor, and immune cells control blood-brain barrier penetration by AAV-PHP.eB in mice. Theranostics, 11(3), 1177–1191. DOI 10.7150/thno.46992. [Google Scholar] [CrossRef]
13. Bendix Johnsen, K., Burkhart, A., Thomsen, L. B., Lars Andresen, T., Moos, T. (2019). Targeting the transferrin receptor for brain drug delivery. Progress in Neurobiology, 181, 101665. DOI 10.1016/j.pneurobio.2019.101665. [Google Scholar] [CrossRef]
14. Han, B., Xie, W., Zhang, Y., Zhou, S., Zhang, X. (2020). The influx/efflux mechanisms of d-peptide ligand of nAChRs across the blood-brain barrier and its therapeutic value in treating glioma. Journal of Controlled Release, 327, 384–396. DOI 10.1016/j.jconrel.2020.08.010. [Google Scholar] [CrossRef]
15. Neuwelt, E. A., Bauer, B., Fahlke, C., Fricker, G., Ladecola, C. et al. (2011). Engaging neuroscience to advance translational research in brain barrier biology. Nature Reviews Neuroscience, 12(3), 169–182. DOI 10.1038/nrn2995. [Google Scholar] [CrossRef]
16. Chen, Y., Liu, L. (2012). Modern methods for delivery of drugs across the blood—brain barrier. Advanced Drug Delivery Reviews, 64(7), 640–665. DOI 10.1016/j.addr.2011.11.010. [Google Scholar] [CrossRef]
17. Abbott, N. J., Ronnback, L., Hansson, E. (2006). Astrocyte-endothelial interactions at the blood-brain barrier. Nature Reviews Neuroscience, 7(1), 41–53. DOI 10.1038/nrn1824. [Google Scholar] [CrossRef]
18. Gao, P., Chen, Q., Hu, J. F., Lin, Y. Y., Lin, J. J. et al. (2020). Effect of ultrawideband electromagnetic pulses on blood brain barrier permeability in rats. Molecular Medicine Reports, 22(4), 2775–2782. DOI 10.3892/mmr.2020.11382. [Google Scholar] [CrossRef]
19. Caterina, B., Lee, R., Genovesi, L. A., Kojic, M., Millar, A. et al. (2020). Comparative study of preclinical mouse models of high-grade glioma for nanomedicine research: The importance of reproducing blood-brain barrier heterogeneity. Theranostics, 10(14), 6361–6371. DOI 10.7150/thno.46468. [Google Scholar] [CrossRef]
20. Sweeney, M., Sagare, A. P., Zlokovic, B. V. (2018). Blood-brain barrier breakdown in Alzheimer disease and other neurodegenerative disorders. Nature Reviews Neurology, 14(3), 133–150. DOI 10.1038/nrneurol.2017.188. [Google Scholar] [CrossRef]
21. Yang, C., Hawkins, K. E., Dore, S., Candelario-Jalil, E. (2018). Neuroinflammatory mechanisms of blood-brain barrier damage in ischemic stroke. AJP Cell Physiology, 316(2), C135–C153. DOI 10.1152/ajpcell.00136.2018. [Google Scholar] [CrossRef]
22. Wen, L. J., Tan, Y. N., Dai, S. H., Zhu, Y., Meng, T. T. et al. (2017). VEGF-mediated tight junctions pathological fenestration enhances doxorubicin-loaded glycolipid-like nanoparticles traversing BBB for glioblastoma-targeting therapy. Drug delivery, 24(1), 1843–1855. DOI 10.1080/10717544.2017.1386731. [Google Scholar] [CrossRef]
23. Wen, L. J., Wang, K., Zhang, F. T., Tan, Y. N., Shang, X. W. et al. (2020). AKT activation by SC79 to transiently re-open pathological blood brain barrier for improved functionalized nanoparticles therapy of glioblastoma. Biomaterials, 237, 119793. DOI 10.1016/j.biomaterials.2020.119793. [Google Scholar] [CrossRef]
24. Jiang, X., Andjelkovic, A. V., Zhu, L. (2017). Blood-brain barrier dysfunction and recovery after ischemic stroke. Progress in Neurobiology, 163–164(Suppl 1), 144–171. DOI 10.1016/j.pneurobio.2017.10.001. [Google Scholar] [CrossRef]
25. Rubin, L. L., Staddon, J. M. (1999). The cell biology of the blood-brain barrier. Annuual Review of Neuroscience, 22(1), 11–28. DOI 10.1146/annurev.neuro.22.1.11. [Google Scholar] [CrossRef]
26. Heiskala, M., Peterson, P. A., Yang, Y. (2010). The roles of claudin superfamily proteins in paracellular transport. Traffic, 2(2), 92–98. DOI 10.1034/j.1600-0854.2001.020203.x. [Google Scholar] [CrossRef]
27. Kubota, K., Furuse, M., Sasaki, H., Sonoda, N., Fujita, K. et al. (1999). Ca2+-independent cell-adhesion activity of claudins, a family of integral membrane proteins localized at tight junctions. Current Biology, 9(18), 1035–1038. DOI 10.1016/S0960-9822(99)80452-7. [Google Scholar] [CrossRef]
28. Yang, Y., Estrada, E. Y., Thompson, J. F., Liu, W., Rosenberg, G. A. (2007). Matrix metalloproteinase-mediated disruption of tight junction proteins in cerebral vessels is reversed by synthetic matrix metalloproteinase inhibitor in focal ischemia in rat. Journal of Cerebral Blood Flow & Metabolism, 27(4), 697–709. DOI 10.1038/sj.jcbfm.9600375. [Google Scholar] [CrossRef]
29. Furuse, M. (1993). Occludin: A novel integral membrane protein localizing at tight junctions. Journal of Cell Biology, 123(6), 1777–1788. DOI 10.1083/jcb.123.6.1777. [Google Scholar] [CrossRef]
30. Saito, A. C., Higashi, T., Fukazawa, Y., Otani, T., Tauchi, M. et al. (2021). Occludin and tricellulin facilitate formation of anastomosing tight-junction strand network to improve barrier function. Molecular Biology of the Cell, 32(8), 722–738. DOI 10.1091/mbc.E20-07-0464. [Google Scholar] [CrossRef]
31. Saitou, M., Furuse, M., Sasaki, H., Schulzke, J. D., Fromm, M. et al. (2000). Complex phenotype of mice lacking Occludin, a component of tight junction strands. Molecular Biology of the Cell, 11(12), 4131–4142. DOI 10.1091/mbc.11.12.4131. [Google Scholar] [CrossRef]
32. Cardoso, F. L. O., Brites, D., Brito, M. A. (2010). Looking at the blood-brain barrier: Molecular anatomy and possible investigation approaches. Brain Research Reviews, 64(2), 328–363. DOI 10.1016/j.brainresrev.2010.05.003. [Google Scholar] [CrossRef]
33. Zlokovic, B. V. (2008). The blood-brain barrier in health and chronic neurodegenerative disorders. Neuron, 57(2), 178–201. DOI 10.1016/j.neuron.2008.01.003. [Google Scholar] [CrossRef]
34. Sladojevic, N., Stamatovic, S. M., Keep, R. F., Grailer, J. J., Sarma, J. V. et al. (2014). Inhibition of junctional adhesion molecule-A/LFA interaction attenuates leukocyte trafficking and inflammation in brain ischemia/reperfusion injury. Neurobiology of Disease, 67, 57–70. DOI 10.1016/j.nbd.2014.03.010. [Google Scholar] [CrossRef]
35. Stamatovic, S., Keep, R., Andjelkovic, A. (2008). Brain endothelial cell-cell junctions: How to open the blood brain barrier. Current Neuropharmacology, 6(3), 179–192. DOI 10.2174/157015908785777210. [Google Scholar] [CrossRef]
36. Hawkins, B. T., Davis, T. P. (2005). The blood-brain barrier/neurovascular unit in health and disease. Pharmacological Reviews, 57(2), 173–185. DOI 10.1124/pr.57.2.4. [Google Scholar] [CrossRef]
37. Bayir, E., Sendemir, A. (2021). Role of intermediate filaments in blood-brain barrier in health and disease. Cells, 10(6), 1400. DOI 10.3390/cells10061400. [Google Scholar] [CrossRef]
38. Gavard, J., Gutkind, J. S. (2006). VEGF controls endothelial-cell permeability by promoting the beta-arrestin-dependent endocytosis of VE-cadherin. Nature Cell Biology, 8(11), 1223–1234. DOI 10.1038/ncb1486. [Google Scholar] [CrossRef]
39. Tomoaki, M., Felinski, E. A., Antonetti, D. A. (2009). Occludin phosphorylation and ubiquitination regulate tight junction trafficking and vascular endothelial growth factor-induced permeability. Journal of Biological Chemistry, 284(31), 21036–21046. DOI 10.1074/jbc.M109.016766. [Google Scholar] [CrossRef]
40. Yang, Z. H., Liu, L. B., Zhao, L. N., Liu, Y. H., Xue, Y. X. (2014). Permeability of the blood-tumor barrier is enhanced by combining vascular endothelial growth factor with papaverine. Journal of Neuroscience Research, 92(6), 703–713. DOI 10.1002/jnr.23348. [Google Scholar] [CrossRef]
41. Xue, J., Zhao, Z., Zhang, L., Xue, L. J., Shen, S. Y. et al. (2017). Neutrophil-mediated anticancer drug delivery for suppression of postoperative malignant glioma recurrence. Nature Nanotechnology, 12(7), 692–700. DOI 10.1038/nnano.2017.54. [Google Scholar] [CrossRef]
42. Pérez-Asensio, F. J., Hurtado, O., Burguete, M. C., Moro, M. A., Salom, J. B. et al. (2005). Inhibition of iNOS activity by 1400W decreases glutamate release and ameliorates stroke outcome after experimental ischemia. Neurobiology of Disease, 18(2), 375–384. DOI 10.1016/j.nbd.2004.10.018. [Google Scholar] [CrossRef]
43. Ye, X., Zhang, Y. L., Asim, M., Meng, Y. L., Zhang, Z. G. et al. (2012). Neuroprotective and neurorestorative effects of thymosin β4 treatment initiated 6 hours after traumatic brain injury in rats. Journal of Neurosurgery, 116(5), 1081–1092. DOI 10.3171/2012.1.JNS111729. [Google Scholar] [CrossRef]
44. Monaghan-Benson, E., Burridge, K. (2009). The regulation of vascular endothelial growth factor-induced microvascular permeability requires Rac and reactive oxygen species. The Journal of Biological Chemistry, 284(38), 25602–25611. DOI 10.1074/jbc.M109.009894. [Google Scholar] [CrossRef]
45. Schreibelt, G., Kooij, G., Reijerkerk, A., Doorn, R. V., Gringhuis, S. I. et al. (2007). Reactive oxygen species alter brain endothelial tight junction dynamics via RhoA, PI3 kinase, and PKB signaling. FASEB Journal, 21(13), 3666–3676. DOI 10.1096/fj.07-8329com. [Google Scholar] [CrossRef]
46. Perez-Carro, R., Cauli, O., Lopez-Larrubia, P. (2014). Multiparametric magnetic resonance in the assessment of the gender differences in a high-grade glioma rat model. EJNMMI Research, 4(1), 44. DOI 10.1186/s13550-014-0044-4. [Google Scholar] [CrossRef]
47. Hawkins, B. T., Davis, T. P. (2005). The blood-brain barrier/neurovascular unit in health and disease. Pharmacological Reviews, 57(2), 173–185. DOI 10.1124/pr.57.2.4. [Google Scholar] [CrossRef]
48. Wright, J. L., Merchant, R. E. (1994). Blood-brain barrier changes following intracerebral injection of human recombinant tumor necrosis factor-α in the rat. Journal of Neuro-Oncology, 20(1), 17–25. DOI 10.1007/BF01057957. [Google Scholar] [CrossRef]
49. Zhu, Q., Enkhjargal, B., Huang, L., Zhang, L., Zhang, T. Y. et al. (2018). Aggf1 attenuates neuroinflammation and BBB disruption via PI3K/Akt/NF-κB pathway after subarachnoid hemorrhage in rats. Journal of Neuroinflammation, 15(1), 178. DOI 10.1186/s12974-018-1211-8. [Google Scholar] [CrossRef]
50. Hideyuki, I., Hisashi, K., Lindberg, R. L. P., Leppert, D., Gloor, S. M. et al. (2008). Endothelial cell barrier impairment induced by glioblastomas and transforming growth factor β2 involves matrix metalloproteinases and tight junction proteins. Journal of Neuropathology & Experimental Neurology, 67(5), 435–448. DOI 10.1097/NEN.0b013e31816fd622. [Google Scholar] [CrossRef]
51. Zhang, Y. M., Zhou, Y., Qiu, L. B., Ding, G. R., Pang, X. F. (2012). Altered expression of matrix metalloproteinases and tight junction proteins in rats following PEMF-induced BBB permeability change. Biomedical and Environmental Sciences: BES, 25, 197–202. DOI 10.3967/0895-3988.2012.02.011. [Google Scholar] [CrossRef]
52. Liu, X., Su, P., Meng, S. S., Aschner, M., Cao, Y. P. et al. (2017). Role of matrix metalloproteinase-2/9 (MMP2/9) in lead-induced changes in an in vitro blood-brain barrier model. International Journal of Biological Sciences, 13(11), 1351–1360. DOI 10.7150/ijbs.20670. [Google Scholar] [CrossRef]
53. Pardridge, W. M. (2012). Drug transport across the blood-brain barrier. Journal of Cerebral Blood Flow & Metabolism, 32(11), 1959–1972. DOI 10.1038/jcbfm.2012.126. [Google Scholar] [CrossRef]
54. Helms, H. C. C., Kristensen, M., Saaby, L., Fricker, G., Brodin, B. (2020). Drug delivery strategies to overcome the blood-brain barrier (BBB). Handbook of Experimental Pharmacology, 273, 151–183. DOI 10.1007/978-3-030-99654-3. [Google Scholar] [CrossRef]
55. Abbott, N. J. (2013). Blood-brain barrier structure and function and the challenges for CNS drug delivery. Journal of Inherited Metabolic Disease, 36(3), 437–449. DOI 10.1007/s10545-013-9608-0. [Google Scholar] [CrossRef]
56. Lajoie, J. M., Shusta, E. V. (2015). Targeting receptor-mediated transport for delivery of biologics across the blood-brain barrier. Annual Review of Pharmacology and Toxicology, 55(1), 613–631. DOI 10.1146/annurev-pharmtox-010814-124852. [Google Scholar] [CrossRef]
57. Li, J., Zhao, J., Tan, T. T., Liu, M. M., Zeng, Z. W. et al. (2020). Nanoparticle drug delivery system for glioma and its efficacy improvement strategies: A comprehensive review. International Journal of Nanomedicine, 15, 2563–2582. DOI 10.2147/IJN.S243223. [Google Scholar] [CrossRef]
58. Omidi, Y., Kianinejad, N., Kwon, Y., Omidian, H. (2021). Drug delivery and targeting to brain tumors: Considerations for crossing the blood-brain barrier. Expert Review of Clinical Pharmacology, 14(3), 357–381. DOI 10.1080/17512433.2021.1887729. [Google Scholar] [CrossRef]
59. Wu, S. K., Tsai, C. L., Huang, Y., Hynynen, K. (2020). Focused ultrasound and microbubbles-mediated drug delivery to brain tumor. Pharmaceutics, 13(1), 15. DOI 10.3390/pharmaceutics13010015. [Google Scholar] [CrossRef]
60. Hao, R., Sun, B., Yang, L., Ma, C., Li, S. (2020). RVG29-modified microRNA-loaded nanoparticles improve ischemic brain injury by nasal delivery. Drug Delivery, 27(1), 772–781. DOI 10.1080/10717544.2020.1760960. [Google Scholar] [CrossRef]
61. Jiang, Z., Liu, J., Guan, J., Wang, H., Zhan, C. (2021). Self-adjuvant effect by manipulating the bionano interface of liposome-based nanovaccines. Nano Letters, 21(11), 4744–4752. DOI 10.1021/acs.nanolett.1c01133. [Google Scholar] [CrossRef]
62. Sharma, G., Lakkadwala, S., Modgil, A., Singh, J. (2016). The role of cell-penetrating peptide and transferrin on enhanced delivery of drug to brain. International Journal of Molecular Sciences, 17(6), 806. DOI 10.3390/ijms17060806. [Google Scholar] [CrossRef]
63. Xie, J., Shen, Z., Anraku, Y., Kataoka, K., Chen, X. (2019). Nanomaterial-based blood-brain-barrier (BBB) crossing strategies. Biomaterials, 224(Suppl 1), 119491. DOI 10.1016/j.biomaterials.2019.119491. [Google Scholar] [CrossRef]
64. Xia, Q., Zhang, Y., Li, Z., Hou, X., Feng, N. (2019). Red blood cell membrane-camouflaged nanoparticles: A novel drug delivery system for antitumor application. Acta Pharmaceutica Sinica B, 9(4), 675–689. DOI 10.1016/j.apsb.2019.01.011. [Google Scholar] [CrossRef]
65. Bahmani, B., Gong, H., Luk, B. T., Haushalter, K. J., Zhang, J. (2021). Intratumoral immunotherapy using platelet-cloaked nanoparticles enhances antitumor immunity in solid tumors. Nature Communications, 12(1), 999. DOI 10.1038/s41467-021-22311-z. [Google Scholar] [CrossRef]
66. Wu, H. H., Zhou, Y., Tabata, Y., Gao, J. Q. (2018). Mesenchymal stem cell-based drug delivery strategy: from cells to biomimetic. Journal of Controlled Release, 294(1), 102–113. DOI 10.1016/j.jconrel.2018.12.019. [Google Scholar] [CrossRef]
67. Deng, J., Xu, W., Wang, J., Li, Q., Wang, Z. (2021). Cancer cell membrane-coated nanogels as redox/pH dual-responsive drug carrier for tumor-targeted therapy. Journal of Materials Chemistry B, 9(38), 8031–8037. DOI 10.1039/D1TB00788B. [Google Scholar] [CrossRef]
68. Cao, Z., Liu, J. (2020). Bacteria and bacterial derivatives as drug carriers for cancer therapy. Journal of Controlled Release, 326(6), 396–407. DOI 10.1016/j.jconrel.2020.07.009. [Google Scholar] [CrossRef]
69. Luk, B. T., Zhang, L. (2015). Cell membrane-camouflaged nanoparticles for drug delivery. Journal of Controlled Release, 220(Suppl. 2), 600–607. DOI 10.1016/j.jconrel.2015.07.019. [Google Scholar] [CrossRef]
70. Fang, R. H., Kroll, A. V., Gao, W. W., Zhang, L. F. (2018). Cell membrane coating nanotechnology. Advanced Materials, 30(23), 1706759. DOI 10.1002/adma.201706759. [Google Scholar] [CrossRef]
71. Jiao, X., Yu, X., Gong, C., Zhu, H., Zhang, B. et al. (2021). Erythrocyte-cancer hybrid membrane-camouflaged mesoporous silica nanoparticles loaded with gboxin for glioma-targeting therapy. Current Pharmaceutical Biotechnology, 23(6), 835–846. DOI 10.2174/1389201022666210719164538. [Google Scholar] [CrossRef]
72. Wu, J. Y., Li, Y. J., Hu, X. B., Huang, S., Luo, S. L. et al. (2021). Exosomes and biomimetic nanovesicles-mediated anti-glioblastoma therapy: A head-to-head comparison. Journal of Controlled Release, 336(69), 510–521. DOI 10.1016/j.jconrel.2021.07.004. [Google Scholar] [CrossRef]
73. Ma, Y. Y., Zhang, J. N., Rui, Y. L., Rolle, J. Q., Xu, T. et al. (2021). Depletion of glioma stem cells by synergistic inhibition of mTOR and c-Myc with a biological camouflaged cascade brain-targeting nanosystem. Biomaterials, 268, 120564. DOI 10.1016/j.biomaterials.2020.120564. [Google Scholar] [CrossRef]
74. Wu, J. Y., Li, Y. J., Wang, J., Hu, X. B., Huang, S. et al. (2021). Multifunctional exosome-mimetics for targeted anti-glioblastoma therapy by manipulating protein corona. Journal of Nanobiotechnology, 19(1), 405. DOI 10.1186/s12951-021-01153-3. [Google Scholar] [CrossRef]
75. Guo, M., Xia, C., Wu, Y., Zhou, N., Chen, Z. P. et al. (2021). Research progress on cell membrane-coated biomimetic delivery systems. Frontiers in Bioengineering and Biotechnology, 9, 772522. DOI 10.3389/fbioe.2021.772522. [Google Scholar] [CrossRef]
76. He, Z., Zhang, Y., Feng, N. (2020). Cell membrane-coated nanosized active targeted drug delivery systems homing to tumor cells: A review. Materials Science and Engineering: C, 106(12), 110298. DOI 10.1016/j.msec.2019.110298. [Google Scholar] [CrossRef]
77. Tan, S., Wu, T., Zhang, D., Zhang, Z. (2015). Cell or cell membrane-based drug delivery systems. Theranostics, 5(8), 863–881. DOI 10.7150/thno.11852. [Google Scholar] [CrossRef]
78. Chen, M., Cui, Y., Hao, W., Fan, Y., Zhang, J. et al. (2021). Ligand-modified homologous targeted cancer cell membrane biomimetic nanostructured lipid carriers for glioma therapy. Drug Delivery, 28(1), 2241–2255. DOI 10.1080/10717544.2021.1992038. [Google Scholar] [CrossRef]
79. Mojarad-Jabali, S., Farshbaf, M., Walker, P. R., Hemmati, S., Fatahi, Y. et al. (2021). An update on actively targeted liposomes in advanced drug delivery to glioma. International Journal of Pharmaceutics, 602, 120645–120661. DOI 10.1016/j.ijpharm.2021.120645. [Google Scholar] [CrossRef]
80. Breier, G., Damert, A., Blum, S., Reichmann, E., Risau, W. (1998). The role of vascular endothelial growth factor in tumor angiogenesis. The Biology of Tumors, 9, 305–308. DOI 10.1007/978-1-4899-1352-4. [Google Scholar] [CrossRef]
81. Chang, J., Mancuso, M. R., Maier, C., Liang, X. B., Yuki, K. et al. (2017). Gpr124 is essential for blood-brain barrier integrity in central nervous system disease. Nature Medicine, 23(4), 450–460. DOI 10.1038/nm.4309. [Google Scholar] [CrossRef]
82. Liu, W. Y., Wang, Z. B., Zhang, L. C., Wei, X., Li, L. (2012). Tight junction in blood-brain barrier: An overview of structure, regulation, and regulator substances. CNS Neuroscience & Therapeutics, 18(8), 609–615. DOI 10.1111/j.1755-5949.2012.00340.x. [Google Scholar] [CrossRef]
83. Yi, K., Lackay, S. N., Ling, Z., Fu, Z. F. (2009). Role of chemokines in the enhancement of BBB permeability and inflammatory infiltration after rabies virus infection. Virus Research, 144(1–2), 18–26. DOI 10.1016/j.virusres.2009.03.014. [Google Scholar] [CrossRef]
84. Verma, S., Kumar, M., Gurjav, U., Lum, S., Nerurkar, V. R. (2010). Reversal of West Nile virus-induced blood-brain barrier disruption and tight junction proteins degradation by matrix metalloproteinases inhibitor. Virology, 397, 130–138. DOI 10.1016/j.virol.2009.10.036. [Google Scholar] [CrossRef]
85. Focust, K. D., Nurre, E., Montgomery, C. L., Hernandez, A., Chan, C. M. et al. (2009). Intravascular AAV9 preferentially targets neonatal neurons and adult astrocytes. Nature Biotechnology, 27(1), 59–65. DOI 10.1038/nbt.1515. [Google Scholar] [CrossRef]
86. Gaudin, Y. (2000). Rabies virus-induced membrane fusion pathway. The Journal of Cell Biology, 150(3), 601–612. DOI 10.1083/jcb.150.3.601. [Google Scholar] [CrossRef]
87. Ribadeau Dumas, F., Cliquet, F., Gautret, P., Robardet, E., Le Pen, C. et al. (2016). Travel-associated rabies in pets and residual rabies risk, Western Europe. Emerging Infectious Diseases, 22(7), 1268–1271. DOI 10.3201/eid2207.151733. [Google Scholar] [CrossRef]
88. Zhang, Y., Qian, L., Kuang, Y., Liu, J. T., Wang, D. Q. et al. (2022). An adeno-associated virus-mediated immunotherapy for Alzheimer’s disease. Molecular Immunology, 144(4), 26–34. DOI 10.1016/j.molimm.2022.02.006. [Google Scholar] [CrossRef]
89. Qiao, C. Q., Zhang, R. L., Wang, Y. D., Jia, Q., Wang, X. F. et al. (2020). Rabies virus-inspired metal organic frameworks (MOFs) for targeted imaging and chemotherapy of glioma. Angewandte Chemie, 59(39), 16982–16988. DOI 10.1002/anie.202007474. [Google Scholar] [CrossRef]
90. Krizbai, I. A., Nyul-Toth, A., Bauer, H. C., Farkas, A. E., Traweger, A. et al. (2016). Pharmaceutical targeting of the brain. Current Pharmaceutical Design, 22(35), 5442–5462. DOI 10.2174/1381612822666160726144203. [Google Scholar] [CrossRef]
91. Lalatsa, A., Schatzlein, A. G., Uchegbu, I. F. (2014). Strategies to deliver peptide drugs to the brain. Molecular Pharmaceutics, 11(4), 1081–1093. DOI 10.1021/mp400680d. [Google Scholar] [CrossRef]
92. Erdos, E. G., Ervin, G. (1990). Some old and some new ideas on kinin metabolism. Journal of Cardiovascular Pharmacology, 15(Suppl 6), S20–S24. DOI 10.1097/00005344-199015061-00005. [Google Scholar] [CrossRef]
93. Hashizume, K., Black, K. L. (2002). Increased endothelial vesicular transport correlates with increased blood-tumor barrier permeability induced by bradykinin and leukotriene C4. Journal of Neuropathology & Experimental Neurology, 61(8), 725–735. DOI 10.1093/jnen/61.8.725. [Google Scholar] [CrossRef]
94. Sugita, M., Black, K. L. (1998). Cyclic GMP-specific phosphodiesterase inhibition and intracarotid bradykinin infusion enhances permeability into brain tumors. Cancer Research, 58, 914–920. [Google Scholar]
95. Hulper, P., Veszelka, S., Walter, F. R., Wolburg, H., Fallier-Becker, P. et al. (2013). Acute effects of short-chain alkylglycerols on blood-brain barrier properties of cultured brain endothelial cells. British Journal of Pharmacology, 169(7), 1561–1573. DOI 10.1111/bph.12218. [Google Scholar] [CrossRef]
96. Erdlenbruch, B., Alipour, M., Fricker, G., Miller, D. S., Kugler, W. et al. (2010). Alkylglycerol opening of the blood-brain barrier to small and large fluorescence markers in normal and C6 glioma-bearing rats and isolated rat brain capillaries. British Journal of Pharmacology, 140(7), 1201–1210. DOI 10.1038/sj.bjp.0705554. [Google Scholar] [CrossRef]
97. Duan, M., Xing, Y., Guo, J., Chen, H., Zhang, R. (2016). Borneol increases blood-tumour barrier permeability by regulating the expression levels of tight junction-associated proteins. Pharmaceutical Biology, 54(12), 1–10. DOI 10.1080/13880209.2016.1199044. [Google Scholar] [CrossRef]
98. Yu, B., Ruan, M., Dong, X., Yu, Y., Cheng, H. (2013). The mechanism of the opening of the blood-brain barrier by borneol: A pharmacodynamics and pharmacokinetics combination study. Journal of Ethnopharmacology, 150(3), 1096–1108. DOI 10.1016/j.jep.2013.10.028. [Google Scholar] [CrossRef]
99. Zhang, Q. L., Fu, B. M., Zhang, Z. J. (2017). Borneol, a novel agent that improves central nervous system drug delivery by enhancing blood-brain barrier permeability. Drug Delivery, 24(1), 1037–1044. DOI 10.1080/10717544.2017.1346002. [Google Scholar] [CrossRef]
100. Jacobson, K. A., Gao, Z. G. (2006). Adenosine receptors as therapeutic targets. Nature Reviews Drug Discovery, 5(3), 247–264. DOI 10.1038/nrd1983. [Google Scholar] [CrossRef]
101. Sebastiao, A. M., Ribeiro, J. A. (2009). Adenosine receptors and the central nervous system. Handbook of Experimental Pharmacology, 83(3), 471. DOI 10.1007/978-3-540-89615-9. [Google Scholar] [CrossRef]
102. Bynoe, M. S., Viret, C., Yan, A., Kim, D. G. (2015). Adenosine receptor signaling: A key to opening the blood-brain door. Fluids and Barriers of the CNS, 12(1), 20. DOI 10.1186/s12987-015-0017-7. [Google Scholar] [CrossRef]
103. Kim, D. G., Bynoe, M. S. (2014). A2A adenosine receptor regulates the human blood-brain barrier permeability. Molecular Neurobiology, 52(1), 664–678. DOI 10.1007/s12035-014-8879-2. [Google Scholar] [CrossRef]
104. Gao, X., Qian, J., Zheng, S., Changyi, Y. Z., Zhang, J. P. et al. (2014). Overcoming the Blood-brain barrier for delivering drugs into the brain by using adenosine receptor nanoagonist. ACS Nano, 8(4), 3678–3689. DOI 10.1021/nn5003375. [Google Scholar] [CrossRef]
105. Black, K. L., Yin, D., Ong, J. M., Hu, J. W., Konda, B. M. et al. (2008). PDE5 inhibitors enhance tumor permeability and efficacy of chemotherapy in a rat brain tumor model. Brain Research, 1230(Suppl 6), 290–302. DOI 10.1016/j.brainres.2008.06.122. [Google Scholar] [CrossRef]
106. Nakano, S., Matsukado, K., Black, K. L. (1996). Increased brain tumor microvessel permeability after intracarotid bradykinin infusion is mediated by nitric oxide. Cancer Research, 56, 4027–4031. [Google Scholar]
107. Luo, H., Shusta, E. V. (2020). Blood-brain barrier modulation to improve glioma drug delivery. Pharmaceutics, 12(11), 1085. DOI 10.3390/pharmaceutics12111085. [Google Scholar] [CrossRef]
108. Booth, L., Roberts, J. L., Tavalllai, M., Nourbakhsh, A., Chuckalovcak, J. et al. (2015). OSU-03012 and viagra treatment inhibits the activity of multiple chaperone proteins and disrupts the blood-brain barrier: implications for anti-cancer therapies. Journal of Cellular Physiology, 230(8), 1982–1998. DOI 10.1002/jcp.24977. [Google Scholar] [CrossRef]
109. Zhao, S., Yang, J., Wang, L., Peng, S. Y., Yin, J. et al. (2016). NF-κB upregulates type 5 phosphodiesterase in N9 microglial cells: inhibition by sildenafil and yonkenafil. Molecular Neurobiology, 53(4), 1–12. DOI 10.1007/s12035-015-9293-0. [Google Scholar] [CrossRef]
110. Santhanam, L., Christianson, D. W., Nyhan, D., Berkowitz, D. E. (2008). Arginase and vascular aging. Journal of Applied Physiology, 105(5), 1632–1642. DOI 10.1152/japplphysiol.90627.2008. [Google Scholar] [CrossRef]
111. Kovacic, S., Rumora, L., Gjurcevic, E., Klaric, M., Ivkic, G. (2015). Effects of nitric oxide on blood-brain barrier permeability in common carp (Cyprinus carpio L.). American Journal of Veterinary Research, 76(7), 615–624. DOI 10.2460/ajvr.76.7.615. [Google Scholar] [CrossRef]
112. Ferrara, N., Adamis, A. P. (2016). Ten years of anti-vascular endothelial growth factor therapy. Nature Reviews. Drug Discovery, 15(6), 385–403. DOI 10.1038/nrd.2015.17. [Google Scholar] [CrossRef]
113. Takahashi, H., Shibuya, M. (2005). The vascular endothelial growth factor (VEGF)/VEGF receptor system and its role under physiological and pathological conditions. Clinical Science, 109(3), 227–241. DOI 10.1042/CS20040370. [Google Scholar] [CrossRef]
114. Weis, S. M., Sara, M., Cheresh, D. A. (2005). Pathophysiological consequences of VEGF-induced vascular permeability. Nature, 437(7058), 497–504. DOI 10.1038/nature03987. [Google Scholar] [CrossRef]
115. Olsson, A. K., Dimberg, A., Claesson-Welsh, L., Kreuger, J. (2013). VEGF receptor signalling- in control of vascular function. Nature Reviews. Molecular Cell Biology, 7(5), 359–371. DOI 10.1038/nrm1911. [Google Scholar] [CrossRef]
116. Bates, D. O., Harper, S. J. (2002). Regulation of vascular permeability by vascular endothelial growth factors. Vascular Pharmacology, 39(4–5), 225–237. DOI 10.1016/S1537-1891(03)00011-9. [Google Scholar] [CrossRef]
117. Brighi, C., Salimova, E., de Veer, M., Puttick, S., Egan, G. (2022). Translation of focused ultrasound for blood-brain barrier opening in glioma. Journal of Controlled Release, 345(Suppl. 4), 443–463. DOI 10.1016/j.jconrel.2022.03.035. [Google Scholar] [CrossRef]
118. Timbie, K. F., Mead, B. P., Price, R. J. (2015). Drug and gene delivery across the blood–brain barrier with focused ultrasound. Journal of Controlled Release, 219, 61–75. DOI 10.1016/j.jconrel.2015.08.059. [Google Scholar] [CrossRef]
119. Yang, Q., Zhou, Y., Chen, J., Huang, N., Cheng, Y. (2021). Gene therapy for drug-resistant glioblastoma via lipid-polymer hybrid nanoparticles combined with focused ultrasound. International Journal of Nanomedicine, 16, 185–199. DOI 10.2147/IJN.S286221. [Google Scholar] [CrossRef]
120. Yang, F. Y., Horng, S. C. (2011). Ultrasound enhanced delivery of macromolecular agents in brain tumor rat model. Annual International Conference of the IEEE Engineering in Medicine and Biology Society, 2011, pp. 5573–5576. DOI 10.1109/IEMBS.2011.6091348. [Google Scholar] [CrossRef]
121. Kovacs, Z., Werner, B., Rassi, A., Sass, J. O., Martin-Fiori, E. et al. (2014). Prolonged survival upon ultrasound-enhanced doxorubicin delivery in two syngenic glioblastoma mouse models. Journal of Controlled Release, 187, 74–82. DOI 10.1016/j.jconrel.2014.05.033. [Google Scholar] [CrossRef]
122. Wang, L. F., Li, X., Gao, Y. B., Wang, S. M., Zhao, L. et al. (2015). Activation of VEGF/Flk-1-ERK pathway induced blood-brain barrier injury after microwave exposure. Molecular Neurobiology, 52(1), 478–491. DOI 10.1007/s12035-014-8848-9. [Google Scholar] [CrossRef]
123. Quock, R. M., Kouchich, F. J., Ishii, T. K., Lange, D. G. (1987). Microwave facilitation of domperidone antagonism of apomorphine-induced stereotypic climbing in mice. Bioelectromagnetics, 8(1), 45–55. DOI 10.1002/(ISSN)1521-186X. [Google Scholar] [CrossRef]
124. Moriyama, E. (1991). Blood-brain barrier alteration after microwaveinduced hypethermia is purely a thermal effect: I. Temperatue and power measurement. Surgical Neurology, 35(3), 177–182. DOI 10.1016/0090-3019(91)90068-K. [Google Scholar] [CrossRef]
125. Zhang, Y., Li, Q., Hu, J., Wang, C. Q., Wan, D. L. et al. (2022). Nasal delivery of cinnarizine thermo- and ion-sensitive in situ hydrogels for treatment of microwave-induced brain injury. Gels, 8(2), 108. DOI 10.3390/gels8020108. [Google Scholar] [CrossRef]
126. Heydarheydari, S., Firoozabadi, S. M., Mirnajafi-Zadeh, J., Shankayi, Z. (2021). Pulsed high magnetic field-induced reversible blood-brain barrier permeability to enhance brain-targeted drug delivery. Electromagnetic Biology and Medicine, 40(3), 361–374. DOI 10.1080/15368378.2021.1925905. [Google Scholar] [CrossRef]
127. Do, T. D., Ul, A. F., Noh, Y., Kim, M. O., Yoon, J. (2016). Guidance of magnetic nanocontainers for treating Alzheimer’s disease using an electromagnetic, targeted drug-delivery actuator. Journal of Biomedical Nanotechnology, 12(3), 569–574. DOI 10.1166/jbn.2016.2193. [Google Scholar] [CrossRef]
Cite This Article
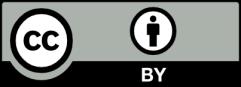
This work is licensed under a Creative Commons Attribution 4.0 International License , which permits unrestricted use, distribution, and reproduction in any medium, provided the original work is properly cited.