Open Access
VIEWPOINT
A Novel Biological Nano Confinement Inhibits Cancer Metastasis
1
First Affiliated Hospital of Anhui University of Science and Technology, Huainan, 232001, China
2
Shanghai Tenth People’s Hospital, Tongji University School of Medicine, Shanghai, 200072, China
3
Shanghai Tenth People’s Hospital Affiliated to Anhui University of Science and Technology, Shanghai, 200072, China
4
Gansu Provincial People’s Hospital, Gansu TCM University, Lanzhou, 730000, China
* Corresponding Author: Jianshe Yang. Email:
Oncologie 2022, 24(3), 591-597. https://doi.org/10.32604/oncologie.2022.025144
Received 24 June 2022; Accepted 13 July 2022; Issue published 19 September 2022
Abstract
Cancer is a complex genetic disease hallmarked with a strong competitive capacity in energy and utilization of substances compared to normal cells, which is partially due to the ability to adjust their metabolism in response to environmental changes. During the lifespan of cancer cells, either during carcinogenesis, progress, or metastasis, massive energy and other substances are essential prerequisites, however, the underlying mechanisms are controversial and still remain unclear. Understanding how cancer cells seize much of the energy and other substances than normal cells is of utmost importance for next-generation cancer therapy, along with the finding of novel drug target and drug design. Recent reports about ‘mitochondrial hijack’ of cancer cells through selfassembled protein nanotubes connected with normal cells and ‘graded messengers pool’ in cytoplasm have evoked a great interest. Considering the widely discussed ‘nanodomain’ in physical and chemical areas, we proposed the concept of biological nano confinement (BNC), by which we may rationally elucidate the priorities of solid tumors on utilization of energy and substances at hypoxia, and less nutrition supplying environments both extraand intra-cellular. The ultimate objective was to address the confusion that CAR-T therapies are effective for hematological cancers but less effective for solid tumors and also to reveal the fact that chimeric antigens receptor-T (CAR-T) adjuvant therapy with chemotherapy has synergetic enhancement effects. In turn, developing novel inhibitors to depolymerize biological nanoconfinement is urgently needed.Keywords
Cancer is one of the most serious devastating diseases after heart disease that is a common cause of death worldwide each year. Due to disease heterogeneity and diversity of standard treatment, cancer treatments are effective only in a subset of the patient population [1]. To date, four main types of standard cancer treatments are clinically adopted. Besides apart of individuals were treated with a single therapy method, most patients have to be treated in combination to tackle the resistant nature of cancer. Surgery can be used when solid tumors have not metastasized and are located in accessible areas of the body. However, many metastasized cancers may need more aggressive treatments, such as radiotherapy and chemotherapy. Aforementioned approaches involve high doses of radiation and drugs to kill cancer cells and shrink tumors but with significant adverse effects meantime. In common practice, the pathological metastasis of cancer may emerge at the very early stage of cancer occurrence, but the clinical metastatic manifestations often lag to a certain extent. In recent decades, with the progressive technical development of early detection and local disease control, the 5-year survival rate for many patients with solid cancers has increased manifold [2,3]. While, for some patients, the increase in late relapses always confused the physicians. Understanding the ways and mechanisms that cancer metastasis occurs and develops will be essential for cancer therapies [4]. Numerous evidence about cancer metastasis might support the possibility of blocking cancer metastasis theoretically, while this is not the case for clinical results. What could be the reason? Is the discovery of the signaling pathways that control cancer metastasis not comprehensive, or are there other ways? In this perspective, by introducing a novel conception of biological nanoconfinement, we have tried to discuss the potential mechanism of cancer cell metastasis regarding the cell energy metabolism.
2 Major Existing Cancer Treatment Strategies
Surgery for cancer therapy has flourished with the advent of anaesthesia and antisepsis, and radical surgery has long been seen as the primary approach to improve the cured rates. Although, the cooperation between trained experts, including oncologists, radiologists, anaesthetists and nurses developed the surgical effect to a new height of minimally invasive and almost innocuous [5], however, the high rate of cancer relapse has largely attenuated the cure efficacy actually.
Radiotherapy has been the classical treatment measure for cancers which was advanced by the radiation-dose fractionation design [6]. In addition, the advances in sensitizing tumors have greatly prompted the radiotherapy effects [7]. However, the multi-point occurrence and metastasis of cancers have remained difficult to be resolved.
Cancer immunotherapy has dramatically changed the way of treating cancer by calibrating the host immune system to recognize and destroy the cancer cells. However, the controversial efficacy of immunotherapy with identified adverse effect has posed great challenges for physicians in treating cancer patients [8], due to lacking the potential for treating solid tumors. Even though leukemia stem cells CAR-T therapy still showed promising outcomes for leukemia patients, which may be associated with energy metabolism [9]. Despite the fact that specific cell surface antigens, self-renewal pathways, signaling pathways, and the epigenome-related cancer stem cells have been focused on in-depth [10–14], the role of energy metabolism associated with these aspects still remains obscure.
3 Cancer and Energy Metabolism
The hallmarks of cancer disease comprise six biological capabilities, including sustaining proliferative signaling, evading growth suppressors, resisting cell death, enabling replicative immortality, inducing angiogenesis, and activating invasion and metastasis. Among these stated hallmarks, genome instability plays an essential and vital role [15]. In addition, the reprogramming of energy metabolism and the avoidance of immune destruction make cancer cells reshape a brand new ‘tumor microenvironment’, making it a bottleneck in treating cancer, and also laying the foundation for the discovery of new cancer treatments [16,17].
Glycolysis has long been considered the major metabolic process for energy and anabolism in cancer cells, which implies that mitochondria play a key role in oncogenesis; hence the mitochondria is regarded as promising target for anticancer agents design [18]. This knowledge upturns our perceptions that cancer stems from epigenetic or genetic alterations [19,20]; and aerobic glycolysis is the only path for energy supply to cancer cells [21,22]; Therefore, tumorigenesis and progression is a very complex interactive process [23,24], suggesting that mitochondrial metabolism plays an inestimable and important role in tumorigenesis [25,26].
4 Priority on Energy and Substance Seizing in Cancer Cells
Tumors are dynamic pseudo organs within which tumor cells interact with other non-tumor cells, thereby forming a complex dynamic network of physiological environments. Therefore, tumor cells face many challenges and try to change their metabolic properties through this network connection to better maintain their malignant proliferation [27].
In general, glycolysis is up-regulated in cancer cells, and the oxidative phosphorylation (OXPHOS) expression should be down-regulated. But OXPHOS was found to be up-regulated in some cancers, even concurrent with active glycolysis [28].
In fact, the metabolic phenotype of cells in the tumor is heterogeneous. The metabolic rate of glucose, lactate, pyruvate and hydroxybutyrate rate in cancer cells is much higher than that of normal cells, suggesting that the metabolic ecology of tumors is very complex. The heterogeneity and complexity of tumor cell metabolism enable it to not only use ATP as an energy source but also perform various functions, including the maintenance of reduction-oxidation balance, producing metabolic coupling with different cell populations, expanding its energy source, and to occupy an active dominant position in energy acquisition [29].
In either case, energy and essential substances required for anabolism are the prerequisites for the development and proliferation of cells. Different cells in the same biological system have the capabilities in competition of energy and essential substances through various routes; only the cells with higher efficiency in energy and essential substances utilization can develop preferentially. Cancer cells are usually in a relative hypoxia environment with less energy supply, while the cancer progress and metastasis need a large amount of energy and materials, suggesting that beyond the traditional energy supply theory, cancer cells must have some ways. Yet we have not recognized to satisfy this demand; so, what are the unknown routes by which cancer cells acquire energy and substances?
Nanoconfinement is confined in a nanosized region. Commonly, when some active materials, such as conjugated polymers, metals, and even bio-active materials (protein, DNA, RNA, etc.) are confined at the nanoscale, they exhibit unique nanoconfined behavior differeing with that observed at macroscale [30]. Moreover, application of nanoconfinement approach to other functional materials such as biomolecules, will provide a basis for the development and design of new strategies for cancer therapy in terms of energy and substance utilization [31–33].
Massive molecular studies revealed that cancer cells generally prioritize in taking up energy or essential substances compared to normal ones throughout their lifespan, yet we did not find any direct and more convincing clues to stand it. Of course, the above cited nanoconfinements are artificially designed to take advantage of their physicochemical properties. Although the substances contained in this confinement have rich diversity, the shell of the confinement is still relatively simple and rigid; hence its utilization in biological systems is greatly restricted. In this context, we believe that there must be a large number of various forms of such nano-confined units that exist in the biological system exerting important functions in the process of tumorigenesis, progression, metastasis and so on. Fortunately, very recent studies shed light on this mysterious phenomenon, which was then called as bio-nano-confinement (BNC).
A recent study reported that cancer cells could hijack the mitochondria from immune cells via physical nanotubes. When inhibiting the nanotube assembly machinery, this hijack was reduced significantly [34–37]. These nanotubes are actually the nanoconfinements, which were constituted of special proteins and glycoproteins that were recruited by the signaling molecules from the extracellular spatial around the non-tumor cells under the control of cancers through trogocytosis action. However, these nanotubes were occasionally found due to their sparsely presence having limited efficacy on mitochondrial hijack (Fig. 1A). This phenomenon involuntarily makes us consider the existence of other forms of nanoconfinements, such as those made up of the cytoplasm or other substances in the intercellular spaces, but have not been fully discovered yet.
Figure 1: Biological nano confinement examples, elucidated by mitochondria hijack (A), and supposed graded messengers pools (B)
G protein-coupled receptors (GPCRs) relay extracellular stimuli into specific cellular functions. Cells have expressed many different GPCRs, almost more than 200 types, but all those GPCRs targeted only a few second messengers, such as cAMP. It is unknown how cells distinguish between signals triggered by different GPCRs to orchestrate their complex functions. A recent study demonstrated that individual GPCRs signal via receptor-associated independent cAMP nanodomains (RAINs) that constitute self-sufficient, independent cell signaling units. The coexistence of many such RAINs allows a single cell to operate thousands of independent cellular signals simultaneously, rather than a simple ‘on/off’ switch (Fig. 1B). We firmly believe that such nanodomains are widely distributed in the cytoplasm and extracellular spaces in different forms and with a specific order, limiting different substances within them [38–41].
Moreover, as one of the star products of immunotherapy, CAR-T has proved with great strength in blood cancer therapy. However, for solid tumors, CAR-T therapy has achieved limited effect. What’s the reason? A previous study suggests that CAR-T therapy has a drawback: T cells entering solid tumors may stop working because of a ‘T-cell failure’ (T cell exhaustion) phenomenon [42]. Clinical CAR-T treatment is usually carried out with monoclonal antibodies (mAbs) due to the limited efficacy of independent use. Although chemotherapy has significant efficacy in hematological tumors, drug resistance is particularly obvious, often accompanied by severe adverse effects. While after CAR T cell re-transfusion, this resistance was reduced; why? Given the blood and lymphatic have higher fluidity, which was not conducive to the formation and maintenance of BNC, thereafter the cancer cells lose priority in the competition of energy and essential substances utilization with normal cells, and concurrent with the specific targeted CAR-T cells attacking, cancer cells become sensitive to the therapeutic interventions, and ultimately showed the good curative effect. On the other hand, CAR-T re-transfusion might have potentially disturbed the cancer cell signaling pathway, thereby regulating the trogocytosis to recruit components from normal cells to construct BNC, eventually causing BNC collapse. Regarding the solid tumors, a relatively stable environment can facilitate the cancer cells to construct BNC, to some extent, making cancer cells resistant to drugs and feasible to proliferate.
Cancer therapy encounters the great challenges by routine ways. With the new findings related to cell energy metabolism and anabolism, bio-nano-confinement (BNC) provide us with a novel conception and promising target for cancer therapy, whether they are solid tumors or not. Of course, the following problems need to be solved urgently: 1) Technologies for BNC characterization in biology systems; 2) What are the forms of BNC in biological systems? 3) Does the BNC interact with known signaling pathways? 4) How do homogeneous and heterogeneous BNC coordinately mediate energy and material uptake? The solution of these problems will bring cancer treatment into a new era.
Ethics Approval and Informed Consent: N/A.
Author Contributions: LS, ZZ: collection of data; data analysis and interpretation; draft writing. YJ, LZ: conception and design; data analysis and interpretation; critical revision of the article content; final approval of the article.
Funding Statement: This work was supported by the National Natural Science Foundation of China (82071964, 72171170), Shanghai Municipal Health Commission (GWV-10.1-XK09), Shanghai Shenkang Center (SHDC2020CR2054B).
Conflicts of Interest: The authors declare that the research was conducted in the absence of any commercial or financial relationships that could be construed as a potential conflict of interest.
References
1. DeSantis, C. E., Lin, C. C., Mariotto, A. B., Siegel, R. L., Stein, K. D. et al. (2014). Cancer treatment and survivorship statistics. CA: A Cancer Journal for Clinicians, 64(4), 252–271. DOI 10.3322/caac.21235. [Google Scholar] [CrossRef]
2. Taya, S. A., Colak, I., Suthar, B., Ramahi, O. M. (2021). Cancer cell detector based on a slab waveguide of anisotropic, lossy, and dispersive left-handed material. Applied Optics, 60(27), 8360–8367. DOI 10.1364/AO.437738. [Google Scholar] [CrossRef]
3. Daher, M. G., Taya, S. A., Colak, I., Vigneswaran, D., Olaimat, M. M. et al. (2022). Design of a nano-sensor for cancer cell detection based on a ternary photonic crystal with high sensitivity and low detection limit. Chinese Journal of Physics, 77, 1168–1181. DOI 10.1016/j.cjph.2022.03.032. [Google Scholar] [CrossRef]
4. Klein, C. A. (2020). Cancer progression and the invisible phase of metastatic colonization. Nature Reviews Cancer, 20(11), 681–694. DOI 10.1038/s41568-020-00300-6. [Google Scholar] [CrossRef]
5. Wyld, L., Audisio, R. A., Poston, G. J. (2015). The evolution of cancer surgery and future perspectives. Nature Reviews Clinical Oncology, 12(2), 115–124. DOI 10.1038/nrclinonc.2014.191. [Google Scholar] [CrossRef]
6. Schaue, D., McBride, W. H. (2015). Opportunities and challenges of radiotherapy for treating cancer. Nature Reviews Clinical Oncology, 12(9), 527–540. DOI 10.1038/nrclinonc.2015.120. [Google Scholar] [CrossRef]
7. Citrin, D. E. (2017). Recent developments in radiotherapy. New England Journal of Medicine, 377(11), 1065–1075. DOI 10.1056/NEJMra1608986. [Google Scholar] [CrossRef]
8. Cable, J., Greenbaum, B., Pe’er, D., Bollard, C. M., Bruni, S. et al. (2021). Frontiers in cancer immunotherapy–A symposium report. Annals of the New York Academy of Sciences, 1489(1), 30–47. DOI 10.1111/nyas.14526. [Google Scholar] [CrossRef]
9. Jones, C. L., Inguva, A., Jordan, C. T. (2021). Targeting energy metabolism in cancer stem cells: Progress and challenges in leukemia and solid tumors. Cell Stem Cell, 28(3), 378–393. DOI 10.1016/j.stem.2021.02.013. [Google Scholar] [CrossRef]
10. Desai, A., Yan, Y., Gerson, S. L. (2019). Concise reviews: Cancer stem cell targeted therapies: Toward clinical success. Stem Cells Translational Medicine, 8(1), 75–81. DOI 10.1002/sctm.18-0123. [Google Scholar] [CrossRef]
11. Pollyea, D. A., Jordan, C. T. (2017). Therapeutic targeting of acute myeloid leukemia stem cells. Blood, 129(12), 1627–1635. DOI 10.1182/blood-2016-10-696039. [Google Scholar] [CrossRef]
12. Gimple, R. C., Bhargava, S., Dixit, D., Rich, J. N. (2019). Glioblastoma stem cells: Lessons from the tumor hierarchy in a lethal cancer. Genes & Development, 33(11–12), 591–609. DOI 10.1101/gad.324301.119. [Google Scholar] [CrossRef]
13. Batlle, E., Clevers, H. (2017). Cancer stem cells revisited. Nature Medicine, 23(10), 1124–1134. DOI 10.1038/nm.4409. [Google Scholar] [CrossRef]
14. Saygin, C., Matei, D., Majeti, R., Reizes, O., Lathia, J. D. (2019). Targeting cancer stemness in the clinic: From hype to hope. Cell Stem Cell, 24(1), 25–40. DOI 10.1016/j.stem.2018.11.017. [Google Scholar] [CrossRef]
15. Rahman, N. (2014). Realizing the promise of cancer predisposition genes. Nature, 505(7483), 302–308. DOI 10.1038/nature12981. [Google Scholar] [CrossRef]
16. Hanahan, D., Weinberg, R. A. (2011). Hallmarks of cancer: The next generation. Cell, 144(5), 646–674. DOI 10.1016/j.cell.2011.02.013. [Google Scholar] [CrossRef]
17. Curtius, K., Wright, N. A., Graham, T. (2018). A. an evolutionary perspective on field cancerization. Nature Reviews Cancer, 18(1), 19–32. DOI 10.1038/nrc.2017.102. [Google Scholar] [CrossRef]
18. Porporato, P. E., Filigheddu, N., Pedro, J. M. B., Kroemer, G., Galluzzi, L. (2018). Mitochondrial metabolism and cancer. Cell Research, 28(3), 265–280. DOI 10.1038/cr.2017.155. [Google Scholar] [CrossRef]
19. Bui, J. D., Schreiber, R. D. (2007). Cancer immunosurveillance, immunoediting and inflammation: Independent or interdependent processes? Current Opinion in Immunology, 19(2), 203–208. DOI 10.1016/j.coi.2007.02.001. [Google Scholar] [CrossRef]
20. Kroemer, G., Senovilla, L., Galluzzi, L., André, F., Zitvogel, L. (2015). Natural and therapy-induced immunosurveillance in breast cancer. Nature Medicine, 21(10), 1128–1138. DOI 10.1038/nm.3944. [Google Scholar] [CrossRef]
21. Erez, A., DeBerardinis, R. J. (2015). Metabolic dysregulation in monogenic disorders and cancer-finding method in madness. Nature Reviews Cancer, 15(7), 440–448. DOI 10.1038/nrc3949. [Google Scholar] [CrossRef]
22. Danhier, P., Bański, P., Payen, V. L., Grasso, D., Ippolito, L. et al. (2017). Cancer metabolism in space and time: Beyond the warburg effect. Biochimica et Biophysica Acta. Bioenergetics, 1858(8), 556–572. DOI 10.1016/j.bbabio.2017.02.001. [Google Scholar] [CrossRef]
23. Chen, D. S., Mellman, I. (2017). Elements of cancer immunity and the cancer-immune set point. Nature, 541(7637), 321–330. DOI 10.1038/nature21349. [Google Scholar] [CrossRef]
24. Galluzzi, L., Buqué, A., Kepp, O., Zitvogel, L., Kroemer, G. (2015). Immunological effects of conventional chemotherapy and targeted anticancer agents. Cancer Cell, 28(6), 690–714. DOI 10.1016/j.ccell.2015.10.012. [Google Scholar] [CrossRef]
25. Vyas, S., Zaganjor, E., Haigis, M. C. (2016). Mitochondria and cancer. Cell, 166(3), 555–566. DOI 10.1016/j.cell.2016.07.002. [Google Scholar] [CrossRef]
26. Wallace, D. C. (2012). Mitochondria and cancer. Nature Reviews Cancer, 12(10), 685–698. DOI 10.1038/nrc3365. [Google Scholar] [CrossRef]
27. Lyssiotis, C. A., Kimmelman, A. C. (2017). Metabolic interactions in the tumor microenvironment. Trends in Cell Biology, 27(11), 863–875. DOI 10.1016/j.tcb.2017.06.003. [Google Scholar] [CrossRef]
28. Ashton, T. M., McKenna, W. G., Kunz-Schughart, L. A., Higgins, G. S. (2018). Oxidative phosphorylation as an emerging target in cancer therapy. Clinical Cancer Research, 24(11), 2482–2490. DOI 10.1158/1078-0432.CCR-17-3070. [Google Scholar] [CrossRef]
29. Martinez-Outschoorn, U. E., Peiris-Pagés, M., Pestell, R. G., Sotgia, F., Lisanti, M. P. (2017). Cancer metabolism: A therapeutic perspective. Nature Reviews Clinical Oncology, 14(1), 11–31. DOI 10.1038/nrclinonc.2016.60. [Google Scholar] [CrossRef]
30. Ko, J., Berger, R., Lee, H., Yoon, H., Cho, J. et al. (2021). Electronic effects of nano-confinement in functional organic and inorganic materials for optoelectronics. Chemical Society Review, 50(5), 3585–3628. DOI 10.1039/d0cs01501f. [Google Scholar] [CrossRef]
31. Liu, G., Müller, A. J., Wang, D. (2021). Confined crystallization of polymers within nanopores. Accounts of Chemical Research, 54(15), 3028–3038. DOI 10.1021/acs.accounts.1c00242. [Google Scholar] [CrossRef]
32. Napolitano, S., Glynos, E., Tito, N. B. (2017). Glass transition of polymers in bulk, confined geometries, and near interfaces. Reports on Progress in Physics, 80(3), 036602. DOI 10.1088/1361-6633/aa5284. [Google Scholar] [CrossRef]
33. Meldrum, F. C., O’Shaughnessy, C. (2020). Crystallization in confinement. Advanced Material, 32(31), e2001068. DOI 10.1002/adma.202001068. [Google Scholar] [CrossRef]
34. Saha, T., Dash, C., Jayabalan, R., Khiste, S., Kulkarni, A. et al. (2022). Intercellular nanotubes mediate mitochondrial trafficking between cancer and immune cells. Nature Nanotechnology, 17(1), 98–106. DOI 10.1038/s41565-021-01000-4. [Google Scholar] [CrossRef]
35. Sharma, P., Allison, J. P. (2020). Dissecting the mechanisms of immune checkpoint therapy. Nature Reviews Immunology, 20(2), 75–76. DOI 10.1038/s41577-020-0275-8. [Google Scholar] [CrossRef]
36. Wolchok, J. (2018). Putting the immunologic brakes on cancer. Cell, 175(6), 1452–1454. DOI 10.1016/j.cell.2018.11.006. [Google Scholar] [CrossRef]
37. Anton, S. E., Kayser, C., Maiellaro, I., Nemec, K., Möller, J. et al. (2022). Receptor-associated independent cAMP nanodomains mediate spatiotemporal specificity of GPCR signaling. Cell, 185(7), 1130–1142.e11. DOI 10.1016/j.cell.2022.02.011. [Google Scholar] [CrossRef]
38. Langeberg, L. K., Scott, J. D. (2015). Signalling scaffolds and local organization of cellular behaviour. Nature Reviews Molecular Cell Biology, 16(4), 232–244. DOI 10.1038/nrm3966. [Google Scholar] [CrossRef]
39. Lefkimmiatis, K., Zaccolo, M. (2014). cAMP signaling in subcellular compartments. Pharmacology & Therapeutics, 143(3), 295–304. DOI 10.1016/j.pharmthera.2014.03.008. [Google Scholar] [CrossRef]
40. Maiellaro, I., Lohse, M. J., Kittel, R. J., Calebiro, D. (2016). cAMP signals in drosophila motor neurons are confined to single synaptic boutons. Cell Reports, 17(5), 1238–1246. DOI 10.1016/j.celrep.2016.09.090. [Google Scholar] [CrossRef]
41. Surdo, N. C., Berrera, M., Koschinski, A., Brescia, M., Machado, M. R. et al. (2017). FRET biosensor uncovers cAMP nano-domains at β-adrenergic targets that dictate precise tuning of cardiac contractility. Nature Communications, 8, 15031. DOI 10.1038/ncomms15031. [Google Scholar] [CrossRef]
42. Chen, J., López-Moyado, I. F., Seo, H., Lio, C. J., Hempleman, L. J. et al. (2019). NR4A transcription factors limit CAR T cell function in solid tumours. Nature, 567(7749), 530–534. DOI 10.1038/s41586-019-0985-x. [Google Scholar] [CrossRef]
Cite This Article
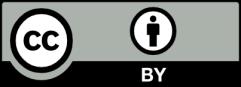
This work is licensed under a Creative Commons Attribution 4.0 International License , which permits unrestricted use, distribution, and reproduction in any medium, provided the original work is properly cited.