Open Access
ARTICLE
Exploring the Potential of Locally Sourced Fungal Chitosan for Paper Mechanical Property Enhancement
1 Latvian State Institute of Wood Chemistry, Riga, LV 1006, Latvia
2 Institute of Energy Systems and Environment, Riga Technical University, Riga, LV 1048, Latvia
* Corresponding Author: Inese Filipova. Email:
Journal of Renewable Materials 2025, 13(3), 583-597. https://doi.org/10.32604/jrm.2024.057663
Received 24 August 2024; Accepted 20 November 2024; Issue published 20 March 2025
Abstract
This study investigated the potential of locally sourced mushrooms as a sustainable alternative to marine-derived chitosan in papermaking. Chitosan was extracted from four local (Boletus edulis, Suillus luteus, Leccinum aurantiacum, Suillus variegatus), one commercially available (Agaricus bisporus) and one laboratory-grown (Phanerochaete chrysosporium) fungal species. Paper handsheets were prepared using either 100% regenerated paper or a 50/50 blend of regenerated paper and hemp fibres. 2.5% chitosan (based on dry mass) was incorporated into the paper mass, using chitosan sourced from B. edulis, A. bisporus, P. chrysosporium, and crustacean chitosan. Fungal chitosan sources were selected based on multiple factors. B. edulis exhibited the highest chitosan yield (5.03%), the highest degree of deacetylation (77.0%) and the highest molecular weight (59.18 kDa). It is also a widely prevalent species in the Baltic region. A. bisporus demonstrated the highest degree of crystallinity (62.7%). Additionally, it has readily available waste material due to its popularity in the food industry. P. chysosporium, with its low degree of crystallinity (33.9%) and small molecular weight (9.06 kDa), is easily cultivable in laboratory conditions. Mechanical testing of papers showed that fungal chitosan significantly improved tensile index and elongation at break (in wet and dry states) and burst strength while reducing air permeability. Notably, fungal chitosan consistently outperformed crustacean chitosan. Commercially available A. bisporus and locally sourced B. edulis emerged as promising alternatives to crustacean chitosan in papermaking. Further research is needed to explore other applications for fungal chitosan.Keywords
In line with the European Union’s Green Deal objectives to reduce greenhouse gas emissions, minimise unnecessary packaging and promote reuse and recycling [1], there has been increasing research focused on replacing plastic packaging with bio-based, sustainable, and recyclable alternatives. One such option is paper packaging. However, paper’s porous structure, which makes it highly susceptible to water and oil, limits its widespread use [2]. Consequently, there is ongoing research into natural additives that can enhance the mechanical properties of paper. Chitosan is a widely studied alternative to plastics [3–6]. Currently, commercial chitosan is derived from the deacetylation of chitin extracted from shrimp shells.
Due to its non-toxicity, biodegradability, good oxygen [7,8] and grease barrier properties [9], chitosan is widely regarded as a promising alternative to synthetic polymers. Chitin, the second most prevalent polysaccharide after cellulose [10], forms the primary structural component of fungal cell walls [11]. Consequently, fungal biomass offers a sustainable alternative to crustacean-based chitosan [12]. The fungal cell wall is a complex, well-ordered structure composed of a polysaccharide backbone, including chitin/chitosan and β-glucans, as well as (galacto)-mannans and glycosylated proteins [13]. The chitin content in fungal cell walls can vary significantly between species, influenced by environmental conditions and age, ranging from 1% to 15% of the fungal cell mass [13].
The production of chitin and chitosan from fungal sources has recently gained significant interest due to their potential advantages over marine-derived counterparts, including uniform polymer length, higher deacetylation levels, and improved solubility [11]. Additionally, the increasing demand for ethically and cruelty-free sourced products is also fuelling the growing interest in fungal chitosan [14].
Replacing crustacean chitosan with fungal chitosan does come with certain challenges. For example, the chitosan yield per wet weight of fungal fruit body or mycelium is relatively low compared to crustacean chitosan [12]. In addition, the extraction method has not yet been developed for industrial-scale production. However, despite the lower chitosan yield, fungal chitosan extraction offers several advantages over crustacean-derived chitosan. These include a simpler extraction process, lower energy consumption, reduced use of inorganic materials, and no need for demineralisation. They can be available with no seasonal or geographic limitations and no sun or extensive land use requirements, as they can be cultivated in vertical farms. Furthermore, fungal chitosan can be produced from readily available mushroom waste, such as stalks and irregular or damaged mushrooms, sourced from the hospitality industry [15].
Traditional paper reinforcing materials are fossil-based and pose significant environmental concerns. While crustacean chitosan has been shown to improve the mechanical properties of paper [2,4,16–18], the potential of locally sourced fungal chitosan as a sustainable alternative warrants further investigation.
With growing interest in chitosan extraction from the fungi, four different fungal species native to the forests of the Baltic region (Boletus edulis, Suillus luteus, Leccinum aurantiacum, Suillus variegatus) were selected for this study. Only damaged or otherwise inedible stipe and pile parts of these mushrooms were used. These species were compared to the commercially available Agaricus bisporus, one of the world’s most widely cultivated edible mushrooms, which generates approximately 50,000 metric tons of waste annually [19]. Additionally, mycelial biomass from Phanerochaete chrysosporium, a model white-rot fungus for lignin degradation, was grown in laboratory conditions as the sixth fungal source of chitosan [13]. Fungal-derived chitosan was then extracted, characterised, and tested as an additive in paper fabrication.
Therefore, this study aimed to demonstrate that chitosan can be successfully extracted from various locally sourced mushrooms, with quality and properties comparable to commercially available crustacean chitosan, establishing its potential as a papermaking additive. While crustacean chitosan has been used as an additive or coating in paper production, fungal chitosan has not previously been incorporated directly with cellulose fibres to improve paper’s mechanical properties. The results of this study show that fungal chitosan outperforms commercial chitosan in all mechanical tests conducted on paper handsheets. Consequently, fungal chitosan presents a promising additive for local-scale papermaking.
The mushrooms B. edulis, S. luteus, L. aurantiacum, and S. variegatus were collected from the forest ecosystem of Latvia (57°05′46.8″N 23°14′27.4″E). Commercially available A. bisporus was purchased from a local grocery store, while P. chrysosporium strain LMKK 407 was obtained from the Microbial Strain Collection of Latvia and cultivated as previously described [13]. Briefly, P. chrysosporium was kept on malt extract agar (MEA) slants at 6°C. Mycelial plugs were then transferred to MEA Petri dishes (5% malt extract, 3% agar, pH 6.0) and incubated at 21 ± 2°C, 70 ± 5% relative humidity (RH) for 14 days. Biomass was cultivated via submerged fermentation. Before chitosan extraction, all fungi were dried overnight at 60°C in the Universal oven U (Memmert GmBH, Schwabach, Germany).
Commercial chitosan from crustaceans, with a deacetylation degree > 90% and medium molecular weight, was acquired from Jiangsu Aoxin Biotechnology Co., Ltd. (Lianyungang, China).
For paper handsheets, two types of controls were used: paper made of 100% regenerated fibres (wastepaper; WP) and a 50/50 blend of regenerated fibres and hemp fibres (HF). The regenerated fibres were sourced from industrially recycled pulp provided by SIA “V.L.T.,” a local manufacturer of moulded fibre products. This pulp comprised 60% mixed wastepaper (journals, newspapers, office paper, books and packaging paper), 30% waste cardboard, 5% printing house waste, and 5% from egg packaging production.
Hemp fibres were prepared using the previously described method [20]. Briefly, hemp fibres were extracted from industrial hemp (Cannabis sativa USO-31). After decortication, the hemp stems were air-dried and cut into 30 × 7 mm pieces. The biomass was then cooked in a 4% NaOH solution at 165°C for 75 min, with a heating rate of 2°C/min. Cooked fibres were rinsed in tap water until the residual water reached a pH of 7.0, then refined to 18°SR freeness using a Blendtec 725 (Orem, UT, USA) at 179 W for 7 min at 1.5% consistency. The fibres were dried at room temperature (RT) and stored dry until use.
2.2 Chitosan Extraction Method
Chitosan extraction was performed following a previously established method [13]. Briefly, fungal fruiting bodies or mycelial biomass were subjected to a two-step chemical extraction process.
In the first step, branched polysaccharides, proteins and glycoproteins were removed by treating dry, pulverised fungal biomass with 1 M NaOH (ratio 1:20 (m/v)) at 90°C for 3 h. Samples were then rinsed with deionised (DI) water until neutral pH, filtered, washed with ethanol and acetone, and dried at 100°C for 1 h. Next, samples were heated in 2% acetic acid (1:40 (m/v); Sigma-Aldrich, St. Louis, MO, USA, Puriss, ≥99.8%) at 90°C for 3 h. This step separated the samples into an acid-insoluble fraction containing chitin and an acid-soluble fraction containing β-glucans, which was discarded. The AIS fraction was washed with DI water, ethanol, and acetone and stored at RT.
In the second step, the AIS fraction was deacetylated by treating it with 10 M (40%) NaOH (1:20 (m/v)) at 90°C for 3 h, followed by the washing and drying steps as in the first phase. The samples were then heated in 2% acetic acid (1:40 (m/v)), filtered, and the filtrate was collected. Chitosan was precipitated by adjusting the pH to 9.0 using NaOH, and its yield was determined gravimetrically.
2.3 Determination of Nitrogen Content
Nitrogen (N) content was determined according to ISO 16948:2015 [21] using Elementar Analysensysteme GmbH (Langenselbold, Germany) Vario MACRO CHNS. Homogenised samples (30 mg) were packed in tin foil and placed into the carousel of an automatic sample feeder, with the combustion tube set to a temperature of 1150°C.
2.4 Fourier Transform Infrared Spectroscopy (FTIR)
A 2 mg of milled sample was mixed with 198 mg KBr powder (IR 145 grade, Sigma-Aldrich, St. Louis, MI, USA) and pressed into tablets. FTIR spectra were recorded using a Nicolet iS50 spectrometer (Thermo Fisher Scientific, Waltham, MA, USA). The spectral range was selected between 4000 and 450 cm−1, with a resolution of 4 cm−1 and 32 scans per sample. Spectra were normalised to the highest absorption maximum.
2.5 Determination of the Degree of Deacetylation
The degree of deacetylation (DDA) of chitosan was determined by potentiometric titration of hydrogen chloride bound to the amino groups of chitosan molecules. Titration was conducted using an SI Analytics Titrolinem 7000 pH meter (Xylen Analytics GmbH, Germany) with an SI Analytics A 162 pH electrode.
0.05 g of chitosan was dissolved in 10 mL of a 0.1 M HCl aqueous solution, followed by the addition of 25 mL of DI. The solution was stirred overnight at RT, then an additional 25 mL of DI water was added, and stirring continued for 30 min to ensure complete dissolution of chitosan. The resulting solution was titrated with a 0.1 M NaOH solution. The DDA was calculated using Eq. (1).
DDA [%]=2.03v2−v1m+0.0042 (v2−v1), (1)
where V1 and V2 are the volumes of the 0.1 M NaOH at the inflection points on the titration curve. The coefficient 2.03 is derived from the molecular weight of the chitin monomer unit; m denotes the sample weight in kilograms. The value 0.0042 is a coefficient based on the difference in molecular weights between the chitin and chitosan monomer units [22].
2.6 Determination of the Degree of Polymerisation (Viscosimetry)
The molecular weight and, consequently, the degree of polymerisation of chitosan was detected with an Ostwald—Pinkevich viscometer. A 2% acetic acid aqueous solution served as the solvent. Various chitosan concentrations were prepared, and their flow rate through the viscometer was recorded. Values obtained from different solutions were plotted on a graph, with viscosity on the Y-axis and concentration on the X-axis. The values were extrapolated to zero concentration on the Y-axis to determine the intrinsic viscosity (η).
To determine the viscosity—average molecular weight (in Daltons), the intrinsic viscosity (η) was used, and the molecular weight was determined using the Mark–Houwink–Sakurada Eq. (2).
[η]=KMα, (2)
where K and α are constants that vary depending on the polymer type and the used solvent. In this case, they were 3.5 × 10−4 for K and 0.76 for α, respectively. M is viscosity average molecular weight.
2.7 X-Ray Diffractometry (XRD)
The crystallinity index (CrI, %) of chitosan was determined using a D8 Advance diffractometer (Bruker, USA) with CuKα-radiation (λ = 0.15418 nm). XRD was performed at 40 kV and 40 mA. Diffractograms were recorded at 0.5 s step time and 0.02° step size with a scan angle between 5° and 50°. Sizes of crystallites were calculated using the Scherrer Eq. (3).
τ=KλβcosΘ, (3)
where τ is the mean size of crystalline domains, K is a shape factor (a constant value) of 0.9, λ represents the X-ray wavelength, β signifies the line broadening at half maximum intensity, corrected for instrumental broadening, in radians and Θ is the Bragg angle.
2.8 Paper Handsheets Fabrication
Seven groups of paper sheets were prepared. Two control groups consisted of either regenerated paper (WP) alone or WP mixed in equal parts with hemp fibres (WPHF). Based on our previous studies [3,4], 2.5% of chitosan (based on dry mass) was added from three mushroom types: A. bisporus, B. edulis, and P. chrysosporium. These groups were labelled according to the fungal source: WP+Be, WP+Ab, WP+Pc, WPHF+Be, WPHF+Ab, WPHF+Pc. Commercial crustacean chitosan was labelled as WP+C or WPHF+C.
Paper handsheets were fabricated following our previously established method [23]. A chitosan solution was prepared by dissolving 1.5 g of chitosan in 150 g of 1% acetic acid solution. Sufficient paper and hemp fibres to produce 10 handsheets with a basis weight of 75 g m−2 were placed in a glass beaker and soaked in 2 L of DI water for 3 h. The fibres were then disintegrated in a Fank disintegrator (PTI, Austria) for 75,000 revolutions. Afterwards, 2.5% of chitosan in acetic acid (based on dry mass) was added to the fibre suspension and mixed for an additional 5000 revolutions. Paper sheets were fabricated according to ISO 5269-2:2004 standard [24] using Rapid Köthen paper machine (PTI, Austria).
2.9 Determination of Paper Properties
Before any experiments, samples were conditioned at 25°C and 50% RH for 24 h. Samples were then cut into 1.5 cm-wide strips using a strip cutter (FRANK-PTI, Austria). Tensile strength (TS, MPa) was tested in accordance with ISO 1924-1:2008 [25]. The burst test was evaluated according to ISO 2758:2014 [26], using an F81838 vertical tensile tester (FRANK-PTI, Laakirchen, Austria).
The tensile index and stretch were measured in both dry and wet states. Paper strips were immersed in water for 30 s for wet testing, and measurements were taken after removing excess water. Six samples per condition were tested to obtain average values.
Air permeability was tested by ISO 5636-3:2013 [27] using Bendtsen tester (Lorentzen & Wettre, Kista, Sweden). The average value of 12 repetitions per condition was recorded.
2.10 Scanning Electron Microscopy (SEM) Analysis
The surface morphology of papers without chitosan or chitosan derived from B. edulis was examined using a scanning electron microscope (Tescan Vega TX, Brno, Czech Republic). Before imaging, paper samples (0.5 × 0.5 cm2) were coated with gold plasma using a K550X sputter coater (Emitech, Chelmsford, UK). SEM micrographs were obtained at 500× and 1000× magnifications.
Data normality and homogeneity of variance were assessed using Levene’s test. Statistical analyses were conducted using IBM SPSS Statistics Version 20.0 (Armonk, NY, USA: IBM Corp). Data are presented as mean ± standard error of the mean (SEM). For data meeting the assumption of normality and equal variances, one-way ANOVA followed by Tukey’s post-hoc test was used. The non-parametric Kruskal-Wallis and Mann-Whitney U-test were used for data that violated these assumptions.
As shown in Table 1, the highest chitosan yield was obtained from B. edulis (5.03 ± 0.18%), which was more than twice the yield from A. bisporus (1.89 ± 0.05%), one of the most widely consumed mushrooms globally. Other fungi showed much lower yields, suggesting that B. edulis would be one of the most promising candidates for chitosan extraction from locally sourced fungi.
3.2 Determination of Nitrogen Content
Depending on the fungal species, the nitrogen content in isolated chitosan ranged from 5.28% to 6.32% (Table 1). The highest nitrogen content was found in A. bisporus, at 6.32 ± 0.05%, which is lower than values reported in other studies, where it ranged from 6.6% to 7.56% [28,29]. This value was also lower than that reported for the crustacean chitosan (7.19%) [28]. The lower nitrogen content reported in this study may be due to the presence of polysaccharides other than chitosan. Since fungal chitin is not pure and is closely associated with glucans, it complicates its extraction [15]. Future studies could benefit from adjustments to the extraction protocol to increase the purity of extracted chitosan.
Fig. 1 shows FTIR spectra of chitosan extracted from B. edulis, P. chrysosporium, and A. bisporus, alongside commercial chitosan derived from crustaceans.
Figure 1: FTIR spectra of chitosan samples extracted from A. bisporus, P. chrysosporium, B. edulis and commercial chitosan from shrimp shells
The FTIR spectra of fungal chitosan exhibited characteristic bands of chitosan resembling those of commercial chitosan used in this study. Chitosan presented a characteristic band in the region of 3430 cm−1, indicating the stretching vibrations of NH amine and OH groups and 2922 cm−1 for CH and C = O stretch. The peaks in regions 1660, 1597 and 1378 cm−1 are linked to amide I, II and III bands. The absence of a band at 1540 cm−1 indicates that chitosan samples are free of residual protein [30]. Similar results were obtained also from other mushrooms in different studies [13,30–32].
The degree of deacetylation (DDA) is essential in determining the possible application of chitosan. Table 2 shows the DDA values of chitosan from six fungal sources.
The highest DDA was detected in B. edulis (77 ± 0.3%), followed by P. chrysosporium (72 ± 0.8%). A. bisporus showed a DDA of 63.4%, comparable to previously reported values of 66.35% [33]. This suggests that the DDA values obtained for other mushroom species are characteristic of their respective types and can vary significantly across species [28]. The DDA from B. edulis and P. chrysosporium were comparable to the low molecular weight (75%–85%) and high molecular weight (70.76%) commercial crustacean chitosan [28].
Regarding application, chitosan films with a DDA of 53% and higher are cytocompatible with several cell lineages. However, the lower the DDA, the lower is cell adhesion and proliferation [34]. For medical applications and different cell growth studies, chitosan with a DDA above 70% is generally preferred [35,36]. This indicates that although commercial chitosan typically has a DDA range of 50% to 100% [10], locally sourced fungal chitosan could also be used for medical studies. Nevertheless, while B. edulis yielded the highest DDA of 77 ± 0.3%, ensuring a consistent supply of fungal chitosan may be best achieved with P. chrysosporium (DDA 72 ± 0.8%), which can be cultivated year-round under controlled conditions.
3.5 Degree of Polymerisation and Crystallinity Index
The degree of polymerisation, or molecular weight, affects the properties of chitosan. Higher molecular weight chitosan has lower water solubility, which limits the range of applications for which it can be used [10]. Fungal chitosan, however, has a viscosity—and thus a molecular weight—that is five times lower than commercially available crustacean chitosan, making it highly attractive for a wide range of applications [28].
Based on the Mark–Houwink–Sakurada equation, the molecular weight of chitosan from six different mushrooms was calculated, with results shown in Table 2. Molecular weight varied substantially among fungi, from the lowest of 8.75 kDa in L. aurantiacum to the highest of 59.18 kDa in B. edulis. Such wide variation has also been observed in other fungal species, ranging from 6.6 kDa in A.coerulea to 560 kDa in M. rouxii chitosan [37].
Chitosan is categorised by molecular weight as low (<150 kDa), medium (150–700 kDa), and high (>700 kDa) [38]. Based on this classification, all locally sourced fungi, as well as A. bisporus and P. chrysosporium, fall into the low molecular weight category. Numerous studies have investigated the impact of chitosan’s molecular weight on its antibacterial properties. While findings are somewhat controversial, most studies conclude that lower molecular weight chitosan exhibits enhanced antibacterial activity [39,40], with a molecular weight range between 10 and 100 kDA shown to be more effective than certain antibiotics [41].
Various hydrolysis methods have been developed to reduce the molecular weight of chitosan [10]. However, each additional processing step increases costs and environmental impact. Therefore, using fungal chitosan, which naturally has a lower molecular weight, offers several advantages over crustacean chitosan. Future studies should examine the antibacterial properties of chitosan derived from locally sourced fungi.
The degree of crystallinity is summarised in Table 2. Among chitosan derived from fungal biomass, A bisporus had the highest crystallinity index (62.7%), while B. edulis showed the lowest (36.9%). P. chrysosporium, with chitosan extracted from its fruiting rather than mycelial biomass, exhibited the lowest values of all the samples (33.9%). The degree of crystallinity varied across fungal species, aligning with values reported for other mushrooms, such as 49.07% in Amanita phalloides [42] and 63.2%–70% (for chitin) for A. bisporus [30].
Fungal chitosan generally has lower crystallinity than crustacean chitosan [37]. Low crystallinity enhances properties such as sorption capacity, accessibility of primary free amino groups, and solubility [30]. In paper fabrication, cellulose chains are organised in crystalline microfibrils with amorphous regions on the surface or as amorphous hydrophilic structures, which substantially affect the barrier properties of the material [43]. Therefore, chitosan with a higher degree of crystallinity would be preferable for paper applications requiring additional strength. Conversely, chitosan with a lower degree of crystallinity would be better suited for paper packaging requiring greater flexibility.
3.6 Determination of Paper Properties
Two control groups were used for the mechanical testing of paper: one consisting of 100% regenerated fibres (WP) and another comprising a 50/50 blend of regenerated and hemp fibres (WPHF). The decision to incorporate 50% hemp fibres was based on our previous research, which demonstrated that adding hemp fibres enhances the mechanical properties of paper handsheets [23].
For test groups, chitosan from A. bisporus (Ab), B. edulis (Be) and P. chrysosporium (Pc) was selected and compared to crustacean-derived chitosan (C) with a DDA of 90%. The selection of fungal chitosan was based on several criteria: A. bisporus exhibited one of the lowest molecular weights and DDA levels, along with a high degree of crystallinity. Its waste materials are widely available due to its popularity in the food industry. B. edulis showed the highest chitosan yield, DDA and molecular weight. In addition, it is the most common mushroom species in the Baltic region. P. chysosporium had the lowest degree of crystallinity and one of the lowest molecular weights. It can be easily cultivated in laboratory conditions with minimal resources and maintenance, making it readily accessible.
Paper samples were tested in dry and wet conditions for stretch at break and tensile index. As illustrated in Fig. 2, stretch at the break in the dry state for both control samples was the lowest among all groups, measuring 1.27 ± 0.05% for 100% regenerated paper (WP) and 0.89 ± 0.04% for regenerated/hemp fibre blend (WPHF). In general, the incorporation of fungal chitosan significantly improved stretch resistance in both WP and WPHF paper groups. There were no significant differences among various chitosan types. However, while adding crustacean chitosan improved stretch at break, its performance did not significantly differ from the control. Among the WP group, the best results were observed with B. edulis chitosan, improving stretch at break by 57% (1.99 ± 0.13%). In the WPHF group A. bisporus achieved the highest improvement, enhancing stretch at break by 139% and reaching 2.13 ± 0.07%, significantly outperforming all other chitosan types.
Figure 2: Stretch at break in the dry and wet states. WP—regenerated paper, WPHF—regenerated paper with hemp fibres, Ab—A. bisporus, Pc—P. chrysosporium, Be—B. edulis, C—crustacean chitosan. *p < 0.05; **p < 0.01; ****p < 0.0001 (ANOVA with Tukey’s post hoc test). Data are represented as ± SEM
In the wet state, the stretch at break for WP was 1.32 ± 0.13%. Adding chitosan significantly improved stretch at break across all the groups except for P. chrysosporium, with the highest increase observed in the B. edulis group, which showed a 124% improvement (2.96 ± 0.23%). Similarly, in the WPHF group (0.83 ± 0.06%), chitosan addition significantly improved stretch at the break across, achieving a maximum of 21% improvement when B. edulis chitosan was added (2.61 ± 0.09%). Although commercial chitosan improved stretch at break, reaching 1.76 ± 0.06%, this increase was significantly lower than that achieved with fungal chitosan groups.
Tensile index values are presented in Fig. 3. In the dry state, WP sample tensile index was 16.78 ± 0.38 Nm/g−1. Unlike crustacean chitosan, fungal chitosan significantly enhanced the tensile index. The best results were achieved with P. chysosporium, reaching 124% improvement (37.56 ± 1.92 Nm/g−1), closely followed by a 112% improvement with B. edulis (35.52 ± 0.94 Nm/g−1). Adding hemp fibres to the regenerated paper significantly improved the tensile index (by 131%), reaching 38.79 ± 1 Nm/g−1 (p = 0.002) compared to the plain WP sample. The addition of chitosan to the WPHF sample significantly improved the tensile index in the dry state, yielding similar results of 48.68 ± 0.91 Nm/g−1 for A. bisporus, 47.65 ± 1.23 Nm/g−1 for B. edulis and 48.99 ± 0.98 Nm/g−1 for crustacean chitosan, which provided a 26% improvement. Although there were no differences among the fungal types, all values were significantly higher than those with crustacean chitosan.
Figure 3: Tensile index in dry and wet states. WP—regenerated paper, WPHF—regenerated paper with hemp fibres, Ab—A. bisporus, Pc—P. chrysosporium, Be—B. edulis, C—crustacean chitosan. *p < 0.05; **p < 0.01; ****p < 0.0001 (Kruskal-Wallis, Mann-Whitney U test for dry state; ANOVA with Tukey’s post hoc test for wet state). Data are shown as ± SEM
In the wet state, the tensile index for the WP group was 2.00 ± 0.17 Nm/g−1, which was significantly lower than in any of the chitosan groups. The highest tensile index with a 236% improvement was recorded with chitosan from A. bisporus (6.72 ± 0.18 Nm/g−1), significantly higher than results from B. edulis (5.24 ± 0.21 Nm/g−1) or commercial chitosan (5.55 ± 0.21 Nm/g−1). Interestingly, in the WPHF group, the wet state tensile index reached only 1.33 ± 0.07 Nm/g−1. While chitosan addition significantly improved tensile index, B. edulis showed comparatively lower effects (5.73 ± 0.17 Nm/g−1) than A. bisporus (8.82 ± 0.08 Nm/g−1) or crustacean chitosan (8.05 ± 0.31 Nm/g−1). Therefore, A. bisporus achieved a 563% improvement over the control, outperforming all other tested chitosans.
While it has been shown that crustacean chitosan can improve tensile strength and strain at break for paper in wet conditions when applied as a coating [44], our results suggest that fungal chitosan surpassed the performance of crustacean chitosan. Depending on the desired paper properties, chitosan from B. edulis offered the best stretch at break performance. For the tensile index, all fungal origin chitosans performed well in the dry state, while A. bisporus chitosan achieved the best results in the wet state.
For burst strength (Fig. 4), the addition of chitosan from A. bisporus increased WP’s burst strength by 38%, while crustacean chitosan decreased it by 10%. Adding hemp fibres increased burst strength by 35%, with an additional 60% increase when A. bisporus chitosan was applied, yielding the best burst strength results.
Figure 4: Burst strength of paper handsheets. WP—regenerated paper, WPHF—regenerated paper with hemp fibres, Ab—A. bisporus, Be—B. edulis, C—crustacean chitosan. Data are represented as ±SEM
Air permeability is important in packaging development, particularly for food products (Fig. 5).
Figure 5: Air permeability. WP—regenerated paper, WPHF—regenerated paper with hemp fibres, Ab—A. bisporus, Be—B. edulis, C—crustacean chitosan. Data are represented as ±SEM
Using only regenerated fibres, the air permeability of handsheets was 1210 ± 25 mL/min. Adding chitosan from A. bisporus had no effect, while chitosan from B. edulis decreased air permeability by 31%, reaching 834.3 ± 113.7 mL/min. Conversely, crustacean chitosan increased air permeability by 14%, reaching 11380 ± 83.5 mL/min.
For the WPHF group, adding hemp fibres to paper made of regenerated fibres naturally decreased air permeability by 92%, reaching 95.5 mL/min. Hemp fibres were selected based on our previous study showing that handsheets made solely of hemp fibres achieved an air permeability of 32 ± 2 mL/min [45]. Only chitosan from B. edulis further reduced air permeability by 22%, reaching the lowest value among all tested samples at 74 ± 13.2 mL/min.
3.7 Paper Surface Morphology (SEM)
The morphology of the paper was evaluated using SEM (Fig. 6), with B. edulis selected as the chitosan source. Regenerated paper (WP, A) displayed large pores between cellulose fibres (indicated by asterisks), which became less prominent when 50% of hemp fibres were added (WPHF, C). Incorporating chitosan into the WP (B) or WPHF (D) paper mass filled these voids between fibres, reducing their prevalence and creating a smoother surface. This contributed to better barrier and mechanical properties in both types of papers.
Figure 6: SEM surface views at 1000× of WP (A), WP+Be (B), WPHF (C), and WPHF+Be (D). WP—regenerated paper, WPHF—regenerated paper with hemp fibres, Be—B. edulis
The observed morphological and mechanical changes in the handsheets can be attributed to the structural similarities between chitosan and cellulose, which promote interactions between both polysaccharides [46]. Chitosan forms hydrogen bonds and/or strong electrostatic interactions with cellulose fibres, filling the pores in the substrate material [43,44,47,48], strengthening it and improving stress distribution throughout the paper structure [40,49]. Similar improvements have been observed when the paper was coated with a chitosan layer [40,44,48,50] or when it was integrated directly into the paper matrix [23]. However, all the former studies have used only marine-sourced chitosan. Fungal chitosan has primarily been studied for biofilm applications rather than for enhancing the mechanical properties of paper packaging. This study is, therefore, the first to incorporate fungal chitosan into the cellulose fibre matrix, demonstrating significant improvements in the mechanical properties of paper.
The study aimed to evaluate the chitosan yield from locally grown mushrooms and to assess its viability as an alternative to commercially available crustacean chitosan in paper manufacturing. Mechanical properties of paper incorporating either fungal or crustacean chitosan were compared. Results demonstrated that fungal chitosan consistently outperformed crustacean chitosan, significantly enhancing tensile index and elongation at break (in both wet and dry states) and burst strength, while reducing air permeability. Although fungal chitosan performance varied among species, the findings suggest that widely cultivated A. bisporus or locally abundant B. edulis, which exhibited the highest chitosan yield, could serve as viable alternatives to crustacean chitosan, depending on the desired paper properties.
Acknowledgement: The authors thank the Latvian State Institute of Wood Chemistry for support by providing scientific infrastructure for this research.
Funding Statement: This research has been funded by the Latvian Council of Science Project No. lzp-2023/1-0123 “Development of Biopolymer-Based Hydrophobic Multi-Layer Packaging Material from Biomass Waste Streams”.
Author Contributions: The authors confirm their contributions to the paper as follows: study conception and design: Laura Andze, Juris Zoldners; data collection: Ulla Milbreta, Marite Skute, Ilze Irbe; analysis and interpretation of results: Ulla Milbreta, Laura Andze, Inese Filipova; draft manuscript preparation: Ulla Milbreta; review and editing: Ulla Milbreta, Laura Andze. All authors reviewed the results and approved the final version of the manuscript.
Availability of Data and Materials: Data that support the findings can be obtained from the corresponding author upon request.
Ethics Approval: The article contains no studies involving human or animal subjects.
Conflicts of Interest: The authors declare no conflicts of interest to report regarding the present study.
References
1. European Commission–Press Release. European green deal: putting an end to wasteful packaging, boosting reuse and recycling. europa.eu. 2022. Available from: https://ec.europa.eu/commission/presscorner/detail/en/ip_22_7155. [Accessed 2024]. [Google Scholar]
2. Wang F-J, Wang L-Q, Zhang X-C, Ma S-F, Zhao Z-C. Enhancement of oil resistance of cellulose packaging paper for food application by coating with materials derived from natural polymers. J Food Eng. 2022;332(1):111039. doi:10.1016/j.jfoodeng.2022.111039. [Google Scholar] [CrossRef]
3. Andze L, Zoldners J, Rozenberga L, Filipova I, Skute M, Laka M, et al. Effect of molecular chitosan on recovered paper properties described by a mathematic model. Cellul Chemist Technol. 2017;52:873–81. [Google Scholar]
4. Andze L, Laka M, Filipova I, Rozenberga L, Grinfelds U, Zoldners J, et al. Effect of chitosan on properties of paper for packaging. Cellul Chemist Technol. 2017;51:67–73. [Google Scholar]
5. Wrońska N, Katir N, Nowak-Lange M, El Kadib A, Lisowska K. Biodegradable chitosan-based films as an alternative to plastic packaging. Foods. 2023;12(18):3519. doi:10.3390/foods12183519. [Google Scholar] [PubMed] [CrossRef]
6. Adibi A, Trinh BM, Mekonnen TH. Recent progress in sustainable barrier paper coating for food packaging applications. Prog Org Coat. 2023;181:107566. doi:10.1016/j.porgcoat.2023.107566. [Google Scholar] [CrossRef]
7. Wang H, Qian J, Ding F. Emerging chitosan-based films for food packaging applications. J Agric Food Chem. 2018;66(2):395–413. doi:10.1021/acs.jafc.7b04528. [Google Scholar] [PubMed] [CrossRef]
8. Leceta I, Guerrero P, de la Caba K. Functional properties of chitosan-based films. Carbohydr Polym. 2013;93(1):339–46. doi:10.1016/j.carbpol.2012.04.031. [Google Scholar] [PubMed] [CrossRef]
9. Milbreta U, Andze L, Filipova I, Dortins E. Effect of nanofibrillated cellulose on alginate and chitosan film properties as potential barrier coatings for paper food packaging. BioResources. 2024;19(2):3375–89. doi:10.15376/biores. [Google Scholar] [CrossRef]
10. Gonçalves C, Ferreira N, Lourenço L. Production of low molecular weight chitosan and chitooligosaccharides (COSa review. Polymers. 2021;13(15):2466. doi:10.3390/polym13152466. [Google Scholar] [PubMed] [CrossRef]
11. Ghormade V, Pathan EK, Deshpande MV. Can fungi compete with marine sources for chitosan production? Int J Biol Macromol. 2017;104:1415–21. doi:10.1016/j.ijbiomac.2017.01.112. [Google Scholar] [PubMed] [CrossRef]
12. Hazmi AT, Ahmad FB, Maziati Akmal MH, Md Ralib AA, Binti Ali F. Fungal chitosan for potential application in piezoelectric energy harvesting: review on experimental procedure of chitosan extraction. Alex Eng J. 2023;67:105–16. doi:10.1016/j.aej.2022.08.020. [Google Scholar] [CrossRef]
13. Irbe I, Andze L, Blumfelde M, Filipova I, Verovkins A, Zoldners J. Harvesting mycelial biomass of selected basidiomycetes for chitosan biopolymer extraction. Polymers. 2023;15(17):3548. doi:10.3390/polym15173548. [Google Scholar] [PubMed] [CrossRef]
14. Aranaz I, Alcántara AR, Civera MC, Arias C, Elorza B, Heras Caballero A, et al. An overview of its properties and applications. Polymers. 2021;13(19):3256. doi:10.3390/polym13193256. [Google Scholar] [PubMed] [CrossRef]
15. Nawawi WMFBW, Jones M, Murphy RJ, Lee KY, Kontturi E, Bismarck A. Nanomaterials derived from fungal sources—is it the new hype? Biomacromolecules. 2020;21(1):30–55. doi:10.1021/acs.biomac.9b01141. [Google Scholar] [PubMed] [CrossRef]
16. Vrabič-Brodnjak U, Yavorov N, Lasheva V, Todorova D. Chitosan-coated packaging papers—strength and thermal stability. Coatings. 2023;13(5):828. doi:10.3390/coatings13050828. [Google Scholar] [CrossRef]
17. Bhardwaj S, Bhardwaj N, Negi Y. Enhancement of processability, surface, and mechanical properties of paper based on rice straw pulp using biopolymers for packaging applications. TAPPI J. 2019;18:431–40. doi:10.32964/TJournal. [Google Scholar] [CrossRef]
18. Khantayanuwong S, Khemarom C, Salaemae S. Effects of shrimp chitosan on the physical properties of handsheets. Agric Nat Resour. 2017;51(1):53–6. doi:10.1016/j.anres.2016.07.006. [Google Scholar] [CrossRef]
19. Abo Elsoud MM, El Kady EM. Current trends in fungal biosynthesis of chitin and chitosan. Bullet Nat Res Cent. 2019;43(1):59. doi:10.1186/s42269-019-0105-y. [Google Scholar] [CrossRef]
20. Filipova I, Andze L, Skute M, Zoldners J, Irbe I, Dabolina I. Improving recycled paper materials through the incorporation of hemp, wood virgin cellulose fibers, and nanofibers. Fibers. 2023;11(12):101. doi:10.3390/fib11120101. [Google Scholar] [CrossRef]
21. ISO 16948:2015; Solid biofuels. Determination of total content of carbon, hydrogen and nitrogen. International Organization for Standartization, Geneva, Switzerland; 2015. Available from: https://www.iso.org/standard/58004.html. [Accessed 2024]. [Google Scholar]
22. Czechowska-Biskup R, Jarosińska D, Rokita B, Ulański P, Rosiak J. Determination of degree of deacetylation of chitosan—comparision of methods. Prog Chem Appl Chitin Deriv. 2012;2012:5–20. [Google Scholar]
23. Andze L, Skute M, Zoldners J, Andzs M, Sirmulis G, Irbe I, et al. Enhancing paper packaging’s wet strength using the synergy between chitosan and nanofibrillated cellulose additives. Polymers. 2024;16(2):227. doi:10.3390/polym16020227. [Google Scholar] [PubMed] [CrossRef]
24. ISO 5269-2: 2004. Pulps—preparation of laboratory sheets for physical testing—part 2: Rapid-Köthen method. International Organization for Standartization, Geneva, Switzerland; 2004. Available from: https://www.iso.org/standard/39341.html. [Accessed 2024]. [Google Scholar]
25. ISO 1924-2: 2008. Paper and board—determination of tensile properties—part 2: Constant rate of elongation method (20 mm/min). International Organization for Standartization, Geneva, Switzerland; 2008. Available from: https://www.iso.org/standard/41397.html. [Accessed 2024]. [Google Scholar]
26. ISO 2758: 2014. Paper—determination of bursting strength. International Organization for Standartization, Geneva, Switzerland; 2014. Available from: https://www.iso.org/standard/61487.html. [Accessed 2024]. [Google Scholar]
27. ISO 5636-3: 2013. Paper and board determination of air permeance (medium range). Part 3: Bendtsen method. International Organization for Standartization, Geneva, Switzerland; 2013. Available from: https://www.iso.org/standard/52431.html. [Accessed 2024]. [Google Scholar]
28. Żukiewicz-Sobczak W, Sobczak P, Zawiślak K, Zagórski J, Wojtyła-Buciora P, Wojtyła A. Physical and chemical properties comparison of fungal and crustaceous chitosan. J Health Inequal. 2015;1(1):7–14. doi:10.5114/jhi.2015.56820. [Google Scholar] [CrossRef]
29. Ssekatawa K, Byarugaba DK, Wampande EM, Moja TN, Nxumalo E, Maaza M, et al. Isolation and characterization of chitosan from Ugandan edible mushrooms, Nile perch scales and banana weevils for biomedical applications. Sci Rep. 2021;11(1):4116. doi:10.1038/s41598-021-81880-7. [Google Scholar] [PubMed] [CrossRef]
30. Alimi BA, Pathania S, Wilson J, Duffy B, Frias JMC. Extraction, quantification, characterization, and application in food packaging of chitin and chitosan from mushrooms: a review. Int J Biol Macromol. 2023;237:124195. doi:10.1016/j.ijbiomac.2023.124195. [Google Scholar] [PubMed] [CrossRef]
31. Yen MT, Mau JL. Physico-chemical characterization of fungal chitosan from shiitake stipes. LWT-Food Sci Technol. 2007;40(3):472–9. doi:10.1016/j.lwt.2006.01.002. [Google Scholar] [CrossRef]
32. Mohd Affandy MA, Rovina K. Characterization of chitosan derived from mushroom sources: physicochemical, morphological, thermal analysis. Sustain Chem Pharm. 2024;40:101624. doi:10.1016/j.scp.2024.101624. [Google Scholar] [CrossRef]
33. Wu J, Niu Y, Jiao Y, Chen Q. Fungal chitosan from Agaricus bisporus (Lange) Sing. Chaidam increased the stability and antioxidant activity of liposomes modified with biosurfactants and loading betulinic acid. Int J Biol Macromol. 2019;123:291–9. doi:10.1016/j.ijbiomac.2018.11.062. [Google Scholar] [PubMed] [CrossRef]
34. Chatelet C, Damour O, Domard A. Influence of the degree of acetylation on some biological properties of chitosan films. Biomaterials. 2001;22(3):261–8. doi:10.1016/S0142-9612(00)00183-6. [Google Scholar] [PubMed] [CrossRef]
35. Murugesan S, Scheibel T. Chitosan-based nanocomposites for medical applications. J Polym Sci. 2021;59(15):1610–42. doi:10.1002/pol.20210251. [Google Scholar] [CrossRef]
36. Foster LJR, Ho S, Hook J, Basuki M, Marçal H. Chitosan as a biomaterial: influence of degree of deacetylation on its physiochemical. Mat Biolog Propert. PLoS One. 2015;10(8):e0135153. [Google Scholar]
37. Wang WP, Du YM, Wang XY. Physical properties of fungal chitosan. World J Microbiol Biotechnol. 2008;24:2717–20. doi:10.1007/s11274-008-9755-x. [Google Scholar] [CrossRef]
38. Román-Doval R, Torres-Arellanes SP, Tenorio-Barajas AY, Gómez-Sánchez A, Valencia-Lazcano AA. Chitosan: properties and its application in agriculture in context of molecular weight. Polymers. 2023;15(13):2867. doi:10.3390/polym15132867. [Google Scholar] [PubMed] [CrossRef]
39. Sarfraz MH, Hayat S, Siddique MH, Aslam B, Ashraf A, Saqalein M, et al. Chitosan based coatings and films: a perspective on antimicrobial, antioxidant, and intelligent food packaging. Prog Org Coat. 2024;188:108235. doi:10.1016/j.porgcoat.2024.108235. [Google Scholar] [CrossRef]
40. Tanpichai S, Witayakran S, Wootthikanokkhan J, Srimarut Y, Woraprayote W, Malila Y. Mechanical and antibacterial properties of the chitosan coated cellulose paper for packaging applications: effects of molecular weight types and concentrations of chitosan. Int J Biol Macromol. 2020;155:1510–9. doi:10.1016/j.ijbiomac.2019.11.128. [Google Scholar] [PubMed] [CrossRef]
41. El-sayed ST, Ali AM, Omar NI. A comparative evaluation of antimicrobial activity of chitooligosaccharides with broad spectrum antibiotics on growth of some pathogenic microorganisms. Biocatal Agric Biotechnol. 2019;22:101382. doi:10.1016/j.bcab.2019.101382. [Google Scholar] [CrossRef]
42. Hemmami H, Ben Amor I, Zeghoud S, Ben Amor A, Laouini SE, Alsalme A, et al. Chitosan extraction from Amanita phalloides: yield, crystallinity, degree of deacetylation, azo dye removal and antibacterial properties. Front Chem. 2024;12:1353524. doi:10.3389/fchem.2024.1353524. [Google Scholar] [PubMed] [CrossRef]
43. Harikrishnan MP, Thampi A, Lal AMN, Warrier AS, Basil M, Kothakota A. Effect of chitosan-based bio coating on mechanical, structural and physical characteristics of microfiber based paper packaging: an alternative to wood pulp/plastic packaging. Int J Biol Macromol. 2023;253:126888. doi:10.1016/j.ijbiomac.2023.126888. [Google Scholar] [PubMed] [CrossRef]
44. Tanpichai S, Srimarut Y, Woraprayote W, Malila Y. Chitosan coating for the preparation of multilayer coated paper for food-contact packaging: wettability, mechanical properties, and overall migration. Int J Biol Macromol. 2022;213:534–45. doi:10.1016/j.ijbiomac.2022.05.193. [Google Scholar] [PubMed] [CrossRef]
45. Filipova I, Irbe I, Spade M, Skute M, Dāboliņa I, Baltiņa I, et al. Mechanical and air permeability performance of novel biobased materials from fungal hyphae and cellulose fibers. Materials. 2021;14(1):136. [Google Scholar]
46. Muxika A, Etxabide A, Uranga J, Guerrero P, de la Caba K. Chitosan as a bioactive polymer: processing, properties and applications. Int J Biol Macromol. 2017;105:1358–68. doi:10.1016/j.ijbiomac.2017.07.087. [Google Scholar] [PubMed] [CrossRef]
47. Kjellgren H, Gällstedt M, Engström G, Järnström L. Barrier and surface properties of chitosan-coated greaseproof paper. Carbohydr Polym. 2006;65(4):453–60. doi:10.1016/j.carbpol.2006.02.005. [Google Scholar] [CrossRef]
48. Fernandes SCM, Freire CSR, Silvestre AJD, Neto CP, Gandini A, Desbriéres J, et al. A study of the distribution of chitosan onto and within a paper sheet using a fluorescent chitosan derivative. Carbohydr Polym. 2009;78(4):760–6. doi:10.1016/j.carbpol.2009.06.012. [Google Scholar] [CrossRef]
49. Bhardwaj S, Bhardwaj NK, Negi YS. Surface coating of chitosan of different degree of acetylation on non surface sized writing and printing grade paper. Carbohydr Polym. 2021;269:117674. doi:10.1016/j.carbpol.2021.117674. [Google Scholar] [PubMed] [CrossRef]
50. Amorin-da-Silva BC, Zambuzi GC, Francisco KR, Verruma-Bernardi MR, Ceccato-Antonini SR. Chitosan-coated paper packaging for specialty coffee beans: coating characterization, bean and beverage analysis. Food Res Intern. 2024;188:114467. doi:10.1016/j.foodres.2024.114467. [Google Scholar] [PubMed] [CrossRef]
Cite This Article
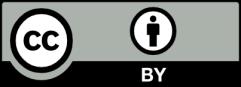
This work is licensed under a Creative Commons Attribution 4.0 International License , which permits unrestricted use, distribution, and reproduction in any medium, provided the original work is properly cited.