Open Access
ARTICLE
Alkali and Plasma-Treated Guadua angustifolia Bamboo Fibers: A Study on Reinforcement Potential for Polymeric Matrices
1 Facultad de Ingeniería, Universidad Nacional de Colombia, Bogotá, 11001, Colombia
2 Facultad de Ciencias, Universidad Nacional de Colombia, Bogotá, 11001, Colombia
* Corresponding Author: Patricia Luna. Email:
(This article belongs to the Special Issue: Natural Fibre Reinforced Polymer Composites: Processing, Manufacturing, Characterizations and Environmentally Friendly Applications)
Journal of Renewable Materials 2024, 12(8), 1399-1416. https://doi.org/10.32604/jrm.2024.052669
Received 10 April 2024; Accepted 11 June 2024; Issue published 06 September 2024
Abstract
This study focuses on treating Guadua angustifolia bamboo fibers to enhance their properties for reinforcement applications in composite materials. Chemical (alkali) and physical (dry etching plasma) treatments were used separately to augment compatibility of Guadua angustifolia fibers with various composite matrices. The influence of these treatments on the fibers’ performance, chemical composition, and surface morphology were analyzed. Statistical analysis indicated that alkali treatments reduced the tensile modulus of elasticity and strength of fibers by up to 40% and 20%, respectively, whereas plasma treatments maintain the fibers’ mechanical performance. FTIR spectroscopy revealed significant alterations in chemical composition due to alkali treatments, while plasma-treated fibers showed minimal changes. Surface examination through Scanning Electron Microscopy (SEM) revealed post-treatment modifications in both cases; alkali treatments served as a cleanser, eliminating lignin and hemicellulose from the fiber surface, whereas plasma treatments also produce rough surfaces. These results validate the impact of the treatments on the fiber mechanical performance, which opens up possibilities for using Guadua angustifolia fibers as an alternative reinforcement in composite manufacturing.Graphic Abstract
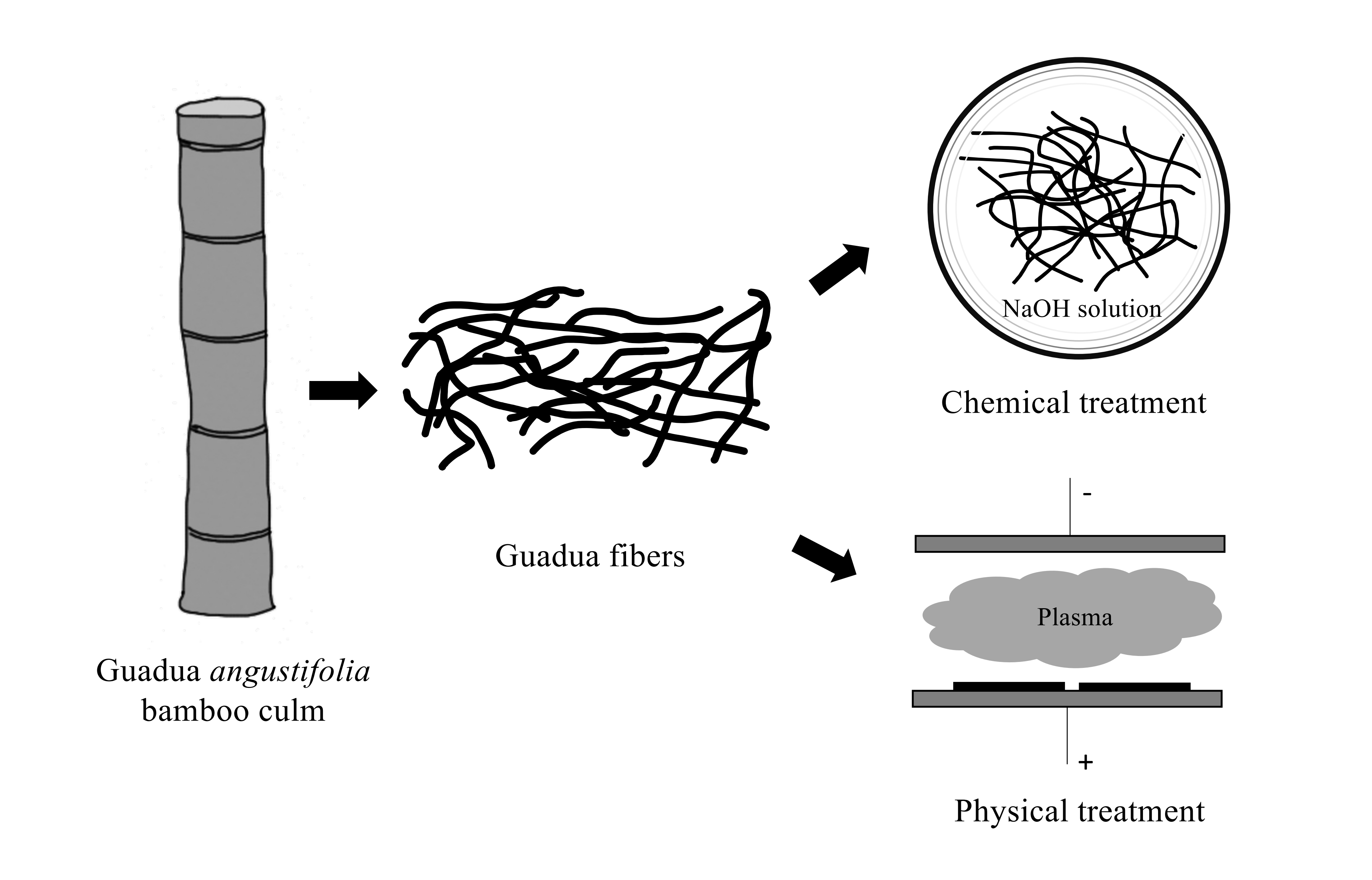
Keywords
Currently, natural fibers are extensively employed as reinforcement within the composite industry, mainly alongside polymer matrices [1–4] in uses that do not demand extensive load-bearing capabilities. Examples such as pineapple, cotton, fique, flax, and bamboo illustrate the widespread adoption of natural fibers for reinforcement purposes. Research by Peças et al. [5] indicates that cotton, pineapple, and bamboo are among the most frequently discussed natural fibers within the Science Direct database. This trend may be linked to the global distribution and accessibility of these plant sources, their immediate suitability for composite reinforcement applications and their inherent mechanical properties. With an annual growth rate projected at 9.59%, the market value of natural fiber composites is estimated to up to USD 41 billion by 2025 [6].
Bamboo is a no timber product belonging to the Gramineae (Poaceae) family, Bambusoideae subfamily, comprising 1575 species from 116 genera worldwide [7]. These plants thrive in both tropical and temperate climates globally, spanning a range of habitats from sea levels to elevated mountainous regions [8]. A particularly remarkable attribute of these forest resources is their rapid growth rate. Chin et al. [9] noted that due to their structural variation as to thermal and mechanical properties, bamboo has been used recently in the composite industry, showing great potential. However, the literature suggests that the most compelling characteristic, as reinforcement in composites, is their high strength-to-weight ratio [5,10].
Guadua angustifolia stands out as the leading bamboo species in Latin America, originating from Colombia, Venezuela, and Ecuador. In Colombia, approximately 51,000 ha of forest are covered with this bamboo type [11], which is integral to the folklore, ecosystem, social customs, and cultural development of some regions. In Colombia, Guadua angustifolia, in its raw (tubular) form, has been a construction material since ancient times, and its mechanical properties have been extensively investigated [12–14]. Laminated Guadua angustifolia has also been the subject of numerous studies [15,16]. In contrast to the extensive research on raw and laminated Guadua angustifolia, there is a lack of research on Guadua angustifolia fibers [17]. In this research, Guadua angustifolia was chosen as the study material, considering that in Colombia it is an abundant resource frequently used as a construction material.
The bamboo plant could be divided into two parts as shown in Fig. 1. The above-ground section includes the culm, branches, and leaves, whereas the underground section compromises the rhizome (Fig. 1a). The culm is comprised of alternating nodes and internodes (Fig. 1b). The wall thickness, external diameter, and internodal length generally vary in the culm. In Guadua angustifolia species, the wall thickness and external diameter decrease linearly from base to top. Regarding wall thickness, the variation ranges from 20 to 5 mm, while the external diameter varies from 100 to 30 mm. On the other hand, the length of the internodes at both the top of the culm and base, which is of shorter length than in the middle, spans from 150 to 350 mm [14]. From an anatomical perspective, the culm has vascular bundles within parenchyma tissue. As depicted in Fig. 1e, there is variation in the shape, size, and density of vascular bundles across the wall, with the outer section being smaller and denser than the middle and inner sections. Vascular bundles are composed of two large metaxylem vessels, one or two protoxylem elements, fiber bundles and phloem (Fig. 1f). As depicted in Fig. 1g, fiber bundles (fibers) consist of microfibrils or elementary fibers.
Figure 1: Parts of a bamboo plant: (a) plant; (b) culm; (c) and (d) wall; (e) distribution of the culm wall; (f) vascular bundle structure: (A) metaxylem vessel component, (B) protoxilem, (C) phloem, (D) fiber bundle (fiber), (E) parenchymal tissue; (g) microfibrils
Generally, in a bamboo culm, fibers have approximately 40% of the volume and 70% of the weight [18]. Torres et al. [19] carried out research to measure the volume of fibers in the Guadua angustofolia species using advanced field microscopy and high-resolution imaging techniques. They found that the fiber volume in the lower, middle and upper parts of the culm are 34%, 41% and 43%, respectively. Bamboo exhibits a tensile strength that generally exceeds that of wood by more than a factor of two, and its specific tensile strength exceeds that of steel by approximately three times. Furthermore, the elongation at break of bamboo fibers typically falls between 4%–10%, exceeding the values observed for both glass and carbon fibers, while being on par with those of PVA (polyvinyl alcohol) fibers [20].
From an environmental perspective, the principal advantages of using natural fibers as reinforcement include that they are renewable resources, their production consumes very little energy, and they contribute to CO2 absorption [21]. The technical advantages of natural fibers, including their high specific stiffness and strength, low density, and cost-efficiency, are particularly notable. Nonetheless, there are disadvantages, such as high variability in properties: mechanical strength, moisture absorption, and durability, and limited bonding in the fiber-matrix interphase [22,23]. Many researchers argue that the chief drawback of employing natural fibers in composite materials is the poor adhesion between phases [24–26], stemming from the fibers’ hydrophilic properties and the polymers’ hydrophobic characteristics [5,22]. This could be solved by modifying the physicochemical properties of natural fibers through chemical or physical methods [27].
The primary goal of chemical treatments is to modify the hydrophilic characteristics of fibers, which are a consequence of the significant amount of hydroxyl groups (-OH) within their chemical structure [28], predominantly found in hemicellulose and lignin [24]. Consequently, these treatments aim to remove these components [25,29,30], enhancing the chemical compatibility of fibers and polymers. Several studies employed alkali (NaOH) solutions for this purpose, achieving significant improvements in the adhesion between polymers and fibers [25,26,31–33]. However, the effect of such treatments on the mechanical performance of fibers is inconclusive. For instance, Sanchez et al. [17] reported a decrease of approximately 20% in tensile strength when bamboo fibers were treated with a 10% NaOH solution. Rajeshkumar et al. [34] treated Phoenix Sp. fibers using different concentrations (5%, 10% and 15%) for 1 h, and found that even though the tensile strength increased for all treatment conditions, a decrease in the modulus of elasticity was obtained for fibers using 5% and 15% NaOH. In contrast, Zidi et al. [32] examined multiple concentrations of NaOH (1%, 2%, 3%, 4%, and 5%) and various durations (from 1 to 5 h) on sisal fibers, noting improvements in tensile strength from each treatment. Furthermore, Zhang et al. [35] noted that a 6% NaOH treatment on bamboo fibers raised both their modulus of elasticity and tensile strength by 38% and 14%.
Physical methods are designed to modify the structural and surface properties of fibers to enhance bonding within composite phases [22,36], through processes such as plasma treatments, vacuum ultraviolet irradiation, ozone, and corona treatments, among others [37]. Plasma formation occurs via highly energetic procedures that involve subjecting a gas to electric discharges or heating it to elevated temperatures. If a potential difference is applied and there is a gas flowing, the gas breaks down into positive ions and electrons that can conduct electricity and initiate a gas discharge. This discharge takes place if an electric field strength in the electrodes suffices to ionize the gas, a phenomenon termed breakdown. The resulting ionized gas, or plasma, consists of positive ions and electrons [38]. Cold plasmas are characterized by electrons with temperatures higher than those of positive ions [39], are frequently used in materials science. Cold plasma is usually produced under low (10−4 to 10−2 kPa) or atmospheric pressures with power sources such as radio frequency (RF), direct current (DC), or microwave (MW), and is frequently boosted by supplementary electric or magnetic fields [40]. DC discharges are commonly utilized because of their simplicity, while MW and RF discharges offer better atom ionization [38].
Cold plasmas are mainly used for surface functionalization, thin film deposition, and etching [41]. These plasmas primarily modify surface properties with a minor impact on bulk properties [36,40,42–44].
Research has shown that plasma treatments (etching) can improve the bonding of natural fibers to polymeric matrices, although these studies are fewer than those for alkali treatments. Plasma gases such as oxygen, helium, argon, or nitrogen have been employed to treat various natural fibers [23,45], effectively enhancing their interfacial adhesion to different matrices [46–50]. These treatments have also been shown to improve the fibers’ mechanical performance, including increases in the tensile strength and the elastic modulus [51–53].
The principal objective of this research is to furnish technical data to advance the utilization of Guadua angustifolia fibers in composite materials. As previously discussed, it is imperative to alter the fibers’ physicochemical properties to enhance the interfacial bonding within the composite phases. To this end, both chemical and physical treatments have been implemented in this study. Subsequently, the modified fibers were subjected to through physical and chemical characterization to evaluate their suitability as an alternative reinforcement within the composite industry.
2.1 Guadua angustifolia Fibers
In this research, Guadua angustifolia culms aged between 3 and 6 years were selected, whose fibers were obtained from the lower part of the plant. The fibers were obtained using an initial chemical-mechanical process, schematically depicted in Fig. 2. The extraction process began by splitting the Guadua angustifolia culms into longitudinal strips (Fig. 2b). The purpose of the chemical process was to soften the material, which was accomplished by immersing the strips for 3 h in a 2.5% sodium hydroxide solution, previously heated to 80°C; the temperature was maintained during the immersion period (Fig. 2c). Following immersion, the strips were thoroughly rinsed with tap water. The fibers were then isolated through a mechanical process using the crushing machine described in [54], and illustrated in Fig. 2d. After extraction, all fibers were meticulously cleansed with drinking water and they were left to dry at laboratory conditions. The average length of extracted fibers was 91.64 mm.
Figure 2: General chemical-mechanical process to obtain Guadua angustifolia bamboo fibers: (a) Guadua angustifolia culm; (b) longitudinal strips; (c) strips immersed in 2.5% NaOH solution heated to 80°C; (d) crushing machine
Chemical and physical treatments were applied to Guadua angustifolia fibers. Given the potential use of these fibers in the composite industry, these treatments aimed at altering the fibers’ physicochemical properties and improving the bonding between composite phases.
2.2.1 Alkali Treatments (Chemical Approach)
In this study, alkali treatments were employed to decrease the hydrophilicity of the extracted fibers. Accordingly, the fibers were bathed in NaOH solutions using 1%, 2.5%, 5%, and 10% concentrations for 1 h. Following this period, the fibers were intensely bathed using clean water.
2.2.2 Dry Etching Plasma Treatments (Physical Approach)
A dry etching plasma treatment was used to change the surface roughness of fibers. This was accomplished by physically bombarding the surface of the fibers to remove material [38]. The treatments were conducted using a DC etching device (Bal-tec SCD 050), which operates with argon gas to generate plasma. Plasma treatments were carried out applying a current of 30 ± 3 mA, a pressure of 10−2 kPa and a voltage of 500 ± 10 V with exposure times of 400, 1000, 1500 and 2000 s.
2.3 Tensile Tests on Treated Fibers
Tensile tests were performed to assess how the treatment conditions impacted the mechanical response of Guadua angustifolia fibers. To accurately capture the mechanical properties, 16 replicas were investigated. The sample size accounted for an expected error of 20 MPa in fiber strength, with a 90% confidence level. Tensile tests were carried out following the ASTM C1557-14, employing a Shimadzu universal testing machine using a 50 N load cell. A loading rate of 1.5 mm/min was used, achieving fiber failure within the standard-recommended 30 s. Samples were placed on paper frames to prevent axial load misalignment during testing, as depicted in Fig. 3. The gauge length was set to 40 mm, and the frame was carefully cut after the sample was secured in the testing grips.
Figure 3: Fiber setup for mechanical characterization of treated Guadua angustifolia fibers
Tensile strength
The fiber strain
The system compliance was established as per ASTM C1557-14. This value is essential for calculating fiber elongation from the total displacement of the cross-head during mechanical tests. According to the standard,
2.5 Tests for Water Absorption
For water absorption tests, approximately 1.5 g of fibers (randomly chosen) were immersed in 200 ml of tap water for time intervals of 0, 1, 2, 3, 6, 12, and 24 h. After immersions, the fibers were centrifuged for 1min to remove excess water. The wet weight was then examined, and the sample was kept in an oven at the temperature of 50°C for 24 h. The drying process was considered complete when consecutive weight measurements, taken at no less than 2-h intervals, differed by no more than 0.010 g. All weights were measured using a precision of 0.001 g. The absorption was quantified as moisture content (MC) using Eq. (4), where
2.6 Fourier Transform Infrared Spectroscopy (FTIR)
FTIR was employed to obtain the fiber functional groups; all samples were conditioned in the laboratory environment overnight. FTIR spectra were acquired through an FT-IR Nicolete iS 10 spectrometer (Thermo Fisher Scientific, Waltham, MA, USA), with 40 scans per spectrum across a range of 4000 to 800 cm−1.
Applying a Tescan Vega 3 SB scanning-electron-microscope (Brno, Czech Republic) in secondary electron mode, the fiber surface morphology was examined.
3.1 System Compliance Calculation
Fig. 4 shows the system compliance for the testing machine. A linear least-square regression was performed on the data points, extrapolated to intersect with the vertical axis. According to ASTM 1557-14, the intercept point corresponds to the
Figure 4: Determination of the system compliance utilized for the testing machine and Guadua angustifolia fibers
For the system compliance tests, Table 1 shows the elastic modulus
By way of illustration, Fig. 5 depicts typical stress-strain curves for Guadua angustifolia fibers, both “as-measured” (raw data) and adjusted for machine compliance. Peaks observed in the curves correspond to the failure of individual fibers within the bundle. It is evident that, at a given stress level, accounting for the system compliance leads to an increased strain reading. This effect translates into higher modulus values when derived from the compliance-corrected data as opposed to those calculated from raw data. Moreover, the stress-strain curves in Fig. 5 exhibit a characteristically brittle nature, which is a recognized attribute of natural fibers, as corroborated by others [56].
Figure 5: Stress-strain curve for Guadua angustifolia fibers, raw and corrected data
An ANOVA evaluation was conducted to obtain notable differences among the mechanical properties corresponding to different gage lengths. Prior to the ANOVA test, the normality and homoscedasticity of each dataset were verified. A significance level of 0.05 was adopted for all cases. The analysis indicated that elastic modulus and tensile strength are not functions of gage length. The variability observed in mechanical properties for a gage length could be attributed to the heterogeneous microstructure of Guadua angustifolia fibers and potential damage suffered during the extraction process, as noted by [57]. Conversely, the strain-to-failure results were consistently higher for the 10 mm gage length and showed a higher variability for gage lengths bigger than 20 mm. This phenomenon, where tensile strength and modulus are independent of gage length while strain-to-failure depends on it, can be explained by the notion that material strength is governed by the average defect size, which remains consistent over all gage lengths, whereas the number of defects (more prevalent in longer fibers) affects the strain-to-failure results [29].
3.2 Results and Discussion on Treated Guadua angustifolia Fibers
This section presents the results for Guadua angustifolia fibers subjected to alkali and dry etching plasma treatments. The following designations were used: H1, H2.5, H5, and H10 denote alkali-treated fibers with the respective NaOH solution concentrations, while P400, P100, P1500, and P2000 indicate plasma-treated fibers with their corresponding exposure times.
Fig. 6 summarizes the elastic modulus, average tensile strength, and strain to failure, with the standard deviation represented by error bars for each sample group.
Figure 6: Mechanical properties of Guadua angustifolia fibers: (a) tensile strength, (b) elastic modulus, (c) strain to failure
As highlighted earlier, Guadua angustifolia fibers exhibit considerable variability in mechanical properties, a characteristic feature of natural fibers. This variability stems primarily from three factors [58]: testing parameters/conditions, intrinsic plane attributes, and cross-sectional area measurements. Since this study maintained consistent extraction parameters and conditions across all tests, and employed a precise methodology for measuring cross-sectional areas, the variation can be predominantly ascribed to innate differences in Guadua angustifolia culms and fiber microstructure, as well as inherent experimental errors. As for tensile strength, as depicted in Fig. 6a, untreated fibers showed a lower standard deviation compared to treated fibers, which may indicate anisotropic alterations in the fiber’s microstructure due to the treatments. Fig. 6b,c does not exhibit notable differences in standard deviations across the various treatments, regarding the elastic modulus and strain to failure.
Fig. 6 demonstrates that both treatments alter the mechanical properties of Guadua angustifolia fibers. Specifically, Fig. 6a shows that both alkali and dry etching plasma treatments lead to a tensile strength reduction, with the alkali treatment resulting causing the most significant decrease. The variations in strain-to-failure and modulus of elasticity are presented in Fig. 6b,c, respectively, do not follow a consistent pattern. Generally, alkali treatments have been found to reduce both the strain-to-failure and stiffness of the fibers, whereas plasma treatments have a less dramatic effect on these properties.
A statistical analysis like that described in the preceding section was conducted to detect significant differences in mechanical performance between treated and untreated fibers. Regarding tensile strength, the ANOVA highlighted disparities among some of the treatment groups: no significant differences were found between the average strength of untreated, H1, H2.5 and all dry etching plasma-treated fibers. However, the tensile strengths for the H5 and H10 treatments were significantly lower in comparison to other groups. The findings agree with earlier studies that applied similar treatment methods [59]. The ANOVA also indicated no substantial differences between the elastic modulus and strain to failure of fibers. The application of alkali treatments can lead to the deterioration of crystalline cellulose, the primary determinant of tensile strength in natural fibers [17]. The results suggest that such degradation becomes notable at alkali solution concentrations of 5% and above. The outcomes from the dry etching plasma treatment confirm that this method does not significantly alter the bulk properties of the material [36,40,42,43].
Fig. 7 shows the moisture content (MC), measured after immersion periods for both alkali and plasma-treated fibers. The absorption process can be divided into two distinct phases for all samples: an initial rapid absorption phase from 0 to 6 h, and a subsequent plateau phase from 6 to 24 h where water uptake stabilizes.
Figure 7: Water absorption as moisture content of treated Guadua angustifolia fibers
The data from alkali-treated fibers indicate that such treatments mitigate MC at all immersion intervals, with the degree of reduction intensifying proportionally to the NaOH solution concentration. This agrees with the findings of other research on other natural fibers subjected to NaOH treatments [60]. Chemical methods are focused on diminishing the hydrophilic characteristics of fibers by removing lignin and hemicellulose. As a result, chemical treatments lead to less hydrophilic fibers, with MC reductions for 24 h immersions of approximately 15%, 18% and 30% for H2.5, H5 and H10 treated fibers, respectively. Remarkably, H1 specimens exhibited lower MCs than untreated fibers for immersions times up to 12 h, yet presented an increase in the final MC, a phenomenon possibly linked to anomalously high relative humidity at the time of dry weight recording.
Contrastingly, the MC for plasma-treated samples was comparable to the untreated fibers. Thus, it is noted that plasma treatments applied here did not significantly alter the hydrophilic properties of investigated fibers. Supporting literature suggests that although plasma treatments may induce complex surface alterations, they tend not to modify the bulk properties of the material.
Fig. 8 presents the normalized FTIR spectra for both alkali-treated and dry-etching-plasma-treated Guadua angustifolia fibers, facilitating the comparison between treatments. Fig. 8a shows that chemical treatments modify the structure of fibers. The wide band from 3600 to 3200 cm−1, associated with hydroxyl group stretching, shows alterations suggestive of lignin and hemicellulose and removal in the alkali-treated fibers [61–63]. In the region from 2919 to 2850 cm−1, the spectral patterns for H2.5, H5 and H10 fibers show a marked reduction in hemicellulose content after treatment [64]. The peaks between 1510 and 1450 cm−1, associated to bending vibrations of lignin’s aromatic C-H bonds, demonstrate a decrease for H5 and H10 fibers, signifying a partial lignin removal [60]. Moreover, the distinct peak at 1421 cm−1, attributed to cellulose bending, and the notable peak from 1100 to 1000 cm−1, associated with C-O stretching and C-H vibration in cellulose, exhibit changes across all alkali-treated fibers due to alkali exposure [65]. These alterations suggest a reduction in the lignin and hemicellulose content, with a concurrent growth in the cellulose exposure, corroborating the observed water absorption behaviors: alkali treatment diminishes fiber’s hydrophilicity. Higher concentrations of the alkali solution enhance the removal of hemicellulose and lignin, which, adversely impacts the stress transfer between elementary fibers [66], thereby decreasing the tensile strength in H5 and H10 samples as seen in tensile tests.
Figure 8: FTIR spectroscopy for treated Guadua angustifolia fibers: (a) alkali-treated fibers, (b) dry-etching-plasma treated fibers
In contrast, the FTIR spectra for dry etching plasma-treated fibers, shown in Fig. 8b, remain relatively unchanged, implying that plasma does not significantly alter the fibers’ composition. This observation agrees with other studies [51]. The changes observed at 3395 and 1240 cm−1 are related to the potential surface removal of hemicellulose and lignin by ion bombardment [67]. Additionally, the pronounced peak shift in the range of 1100 and 1000 cm−1 with increasing exposure time suggests cellulose exposure due to the etching effect. The intensity band around 900 cm−1, linked to the binding of sugar units in hemicellulose [68], decreases in the P1000, P1500 and P2000 spectra, probably because of the material removal effect of plasma on the surface of fibers [69,70]. Thus, it can be argued that plasma treatments, while impacting the fiber surface, do not substantially modify the bulk chemical composition of Guadua angustifolia fibers, which is consistent with mechanical and water absorption results between untreated and plasma-treated fibers.
Fig. 9 shows the surface morphology of alkali-treated Guadua angustifolia fibers. Through scanning electron micrographs, it is evident that a Guadua angustifolia fiber comprises numerous elementary fibers [62,71]. The surface irregularities observed in untreated fibers, characterized by the presence of waxes, hemicellulose, lignin, and oils (Fig. 9a), are reduced after alkali treatment [72,73]. Post-treatment, the fiber surface appears cleaner with fewer irregularities, as shown in Fig. 9b through 9e. This effect is the result of the hemicellulose and lignin removal, corroborated by FTIR and water absorption findings. The pits visible in Fig. 9c,d are indicative of parenchyma cells [74–76]. Additionally, in Fig. 9d,e, it is observable that fibers subjected to 5% and 10% NaOH concentrations have begun to separate into individual elementary fibers. The cohesive function of hemicellulose and lignin, binding elementary cellulose fibers, is compromised upon their removal, thereby diminishing the loading transfer capacity between fibers. As previously noted, a reduced presence of lignin and hemicellulose correlates with the decreased tensile strength of H5 and H10 fibers as demonstrated by the tensile tests.
Figure 9: SEM images for alkali-treated Guadua angustifolia fibers: (a) non-treated, (b) H1, (c) H2.5, (d) H5, (e) H10
The surface of the plasma-treated fibers is depicted in Fig. 10. Regardless of treatment conditions, all plasma-treated fibers display a rough surface with occasional cracks. Compared to untreated fibers (Fig. 10a), plasma-treated fibers (Fig. 10b through 10e) exhibit fewer surface irregularities, which is attributed to the material removal from the ion bombardment effect observed in other studies [77,78]. P400 fibers (Fig. 10b) clearly show crack formation, which becomes more pronounced and continuous in P1000 fibers (Fig. 10c). In P1500 fibers (Fig. 10d), the cracks appear shallower than those in P1000 fibers. With P2000 fibers (Fig. 10e), there is a noticeable reduction in crack visibility, and some areas of the surface begin to smooth out. The sputter-etching mechanism, as described in [79], starts with atomic removal from the material surface, leading to isolated microcracks formation. As ion bombardment is prolonged, these microcracks grow and eventually form large “islands”. With prolonged treatment, these islands coalesce, resulting in a more uniform surface.
Figure 10: SEM images for dry etching plasma treated Guadua angustifolia fibers: (a) non-treated, (b) P400, (c) P1000, (d) P1500, (e) P2000
This research investigated the effects of various treatments on Guadua angustifolia bamboo fibers to enhance their properties as reinforcement in composite materials. The results show that alkali treatments affect the fibers’ performance and “clean” the fibers’ surface. In contrast, plasma treatments do not alter the fibers’ performance but modify their surface. The main findings of this research are detailed below:
1. Tensile Properties vs. Gage Length: Guadua angustifolia fiber’s strain-to-failure decreases when increasing the gage length, while tensile strength and the elastic modulus are not influenced by gage length. It is understood by considering that the average flaw size does not depend on gage length; however, the number of flaws is likely to increase with material volume. Therefore, while the average flaw size may dictate strength and modulus, the prevalence of flaws crucially influences strain-to-failure as crack propagation through these flaws determines the failure strain.
2. Effects of Alkali Treatments: NaOH treatment leads to a reduction of the fiber’s tensile strength while maintaining unaltered strain-to-failure and modulus of elasticity. Additionally, these treatments modify the fibers’ hydrophilic properties by reducing their moisture levels as the concentration of the solution rises. FTIR spectroscopy analyses suggest that this reduction in hydrophilicity is due to the removal of lignin and hemicellulose from fibers. However, the removal of these sticky components adversely affects the fibers’ mechanical strength by diminishing the stress transfer within the elementary fibers. Morphological analyses confirm that the alkali treatments act as “cleaners” by eliminating hemicellulose, lignin and waxes. Furthermore, treatment with 5% NaOH and higher results in the release of individual fibers.
3. Effects of Plasma Treatments: Applied dry-etching-plasma does not compromise the tensile properties of natural fibers, and appears to primarily affect the fibers’ surface properties. Furthermore, plasma treatments do not alter the fibers’ water absorption properties. FTIR spectroscopy analyses reveal no significant changes in fibers’ chemical composition, supporting the unaltered properties after dry etching processes. The morphological analyses show that the fibers’ surface roughness increases, leading to the formation of crack “islands” that expand with prolonged treatment duration. Over extended periods, these “islands” tend to coalesce, resulting in a more continuous surface.
In the next phase of research on this subject, the Guadua angustifolia fibers will be applied as the reinforcement of composite materials, and the effects of fiber treatments on the performance of composites will be evaluated.
Acknowledgement: The authors would like to thank everyone who has provided a lot of support in completing this research, especially the support provided by Science, Technology and Innovation Colombian Ministry (Ministerio de Ciencia, Tecnología e Innovación de Colombia).
Funding Statement: Science, Technology and Innovation Colombian Ministry (Ministerio de Ciencia, Tecnología e Innovación de Colombia), through Funding 6172.
Author Contributions: The authors confirm contribution to the paper as follows: study conception and design: Patricia Luna, Juan Lizarazo-Marriaga, Alvaro Mariño; data collection: Patricia Luna; analysis and interpretation of results: Patricia Luna, Juan Lizarazo-Marriaga, Alvaro Mariño; draft manuscript preparation: Patricia Luna, Juan Lizarazo-Marriaga. All authors reviewed the results and approved the final version of the manuscript.
Availability of Data and Materials: The authors confirm that the data supporting the findings of this study are available within the article.
Conflicts of Interest: The authors declare that they have no conflicts of interest to report regarding the present study.
References
1. Alsubari S, Zuhri MYM, Sapuan SM, Ishak MR, Ilyas RA, Asyraf MRM. Potential of natural fiber reinforced polymer composites in sandwich structures: a review on its mechanical properties. Polymers. 2021;13(3):1–20. doi:10.3390/polym13030423. [Google Scholar] [PubMed] [CrossRef]
2. Mulenga TK, Ude AU, Vivekanandhan C. Techniques for modelling and optimizing the mechanical properties of natural fiber composites: a review. Fibers. 2021;9(1):1–17. doi:10.3390/fib9010006. [Google Scholar] [CrossRef]
3. Zhan J, Li J, Wang G, Guan Y, Zhao G, Lin J, et al. Review on the performances, foaming and injection molding simulation of natural fiber composites. Polym Compos. 2021;42(3):1305–24. doi:10.1002/pc.25902. [Google Scholar] [CrossRef]
4. Kuram E. Advances in development of green composites based on natural fibers: a review. Emergent Mater. 2022;5(3):811–31. doi:10.1007/s42247-021-00279-2. [Google Scholar] [CrossRef]
5. Peças P, Carvalho H, Salman H, Marco L. Natural fibres composites and their applications: a review. J Compos Sci. 2018;2(4):1–20. doi:10.3390/jcs2040066. [Google Scholar] [CrossRef]
6. Sun Z, Duan Y, An H, Wang X, Liang S, Li N. Research progress and application of natural fiber composites. J Nat Fibers. 2023;20(2):44. doi:10.1080/15440478.2023.2206591. [Google Scholar] [CrossRef]
7. Sonarkhan MP, Singh L, Sungkaew S, Souvannakhoummane K, Thul ST. Silica and secondary metabolites as chemophenetic markers for characterization of bamboo species in relation to genetic and morphometric analysis. Mol Biol Rep. 2021;48(5):4487–95. doi:10.1007/s11033-021-06469-9. [Google Scholar] [PubMed] [CrossRef]
8. Singh G, Richa, Sharma ML. Bamboo–A miracle plant. Int J Curr Res Biosci Plant Biol. 2017;4(1):110–2. doi:10.20546/ijcrbp.2017.401.013. [Google Scholar] [CrossRef]
9. Chin SC, Tee KF, Tong FS, Ong HR, Gimbun J. Thermal and mechanical properties of bamboo fiber reinforced composites. Mater Today Commun. 2020;23:100876. doi:10.1016/j.mtcomm.2019.100876. [Google Scholar] [CrossRef]
10. Scherer JF, Bom RP. Determination of shear modulus in bamboo fibers composite in torsion tests determination of shear modulus in bamboo fibers composite in torsion tests. Mater Res Express. 2019;6(3):035310. [Google Scholar]
11. Gomez JP, Velez JPA, Pinzon MA, Arango JAM, Muriel AP. Chemical characterization and antiradical properties of pyroligneous acid from a preserved bamboo, Guadua angustifolia Kunth. Braz Arch Biol Technol. 2021;64(66):1–13. doi:10.1590/1678-4324-2021190730. [Google Scholar] [CrossRef]
12. Acosta R, Montoya JA, Welling J. Determination of the suitable shape for tensile tests parallel to the fibers in Guadua angustifolia Kunth specimens. BioResources. 2021;16(2):3214–23. doi:10.15376/biores.16.2.3214-3223. [Google Scholar] [CrossRef]
13. Padilla J, Guachamín-Acero W, Guerrero VH, Monar W, Pontón PI, Guamán MV. Dataset on the mechanical and physical characterization of the Ecuadorian Guadua angustifolia kunth bamboo culms belonging to “Caña Mansa” biotype. Data Brief. 2024;52(4):109969. doi:10.1016/j.dib.2023.109969. [Google Scholar] [PubMed] [CrossRef]
14. Rivera-Segura R, Moya R, Gaitán-Alvarez J, Granados-Gamboa M. Analysis of the physical-mechanical properties of Dendrocalamus asper (Schult.) versus two Guadua angustifolia (Kunth) varieties, south and caribe in the Atlantic Region of Costa Rica. Adv Bamboo Sci. 2024;6:100057. doi:10.1016/j.bamboo.2024.100057. [Google Scholar] [CrossRef]
15. Estrada M, Linero DL, Takeuchi C. Numerical model of cracking pattern in laminated bamboo specimens under tensile and shear loads. Frattura ed Integrita Strutturale. 2019;13(48):348–56. doi:10.3221/IGF-ESIS.48.33. [Google Scholar] [CrossRef]
16. Takeuchi CP, Estrada M, Linero DL. The elastic modulus and poisson’s ratio of laminated bamboo Guadua angustifolia. Key Eng Mater. 2016;668:126–33. doi:10.4028/www.scientific.net/KEM.668.126. [Google Scholar] [CrossRef]
17. Sánchez M, Patiño W, Cárdenas J. Methods of surface treatment and its effect on the physical and mechanical properties of guadua fibers. Scientia et Technica. 2020;25(1):183–8. [Google Scholar]
18. Chen X, Wang X, Luo X, Chen L, Li Y, Xu J, et al. Bamboo as a naturally-optimized fiber-reinforced composite: interfacial mechanical properties and failure mechanisms. Compos B: Eng. 2024;279:111458. doi:10.1016/j.compositesb.2024.111458. [Google Scholar] [CrossRef]
19. Torres E, Plata A, Díaz G, Takeuchi C. Analysis of the fiber density in bamboo Guadua angustifolia Kunth by extended field microscopy and high resolution images processing. Key Eng Mater. 2014;600:10–4 doi:10.4028/www.scientific.net/KEM.600.10. [Google Scholar] [CrossRef]
20. Gao X, Zhu D, Fan S, Rahman MZ, Guo S, Chen F. Structural and mechanical properties of bamboo fiber bundle and fiber/bundle reinforced composites: a review. J Mater Res Technol. 2022;19(1):1162–90. doi:10.1016/j.jmrt.2022.05.077. [Google Scholar] [CrossRef]
21. Feng Y, Hao H, Lu H, Chow CL, Lau D. Exploring the development and applications of sustainable natural fiber composites: a review from a nanoscale perspective. Compos B: Eng. 2024;276:111369. doi:10.1016/j.compositesb.2024.111369. [Google Scholar] [CrossRef]
22. Halip JA, Hua LS, Ashaari Z, Tahir MP, Chen LW, Anwar Uyup MK. Effect of treatment on water absorption behavior of natural fiber-reinforced polymer composites Mechanical and Physical Testing of Biocomposites, Fibre-Reinforced Composites and Hybrid Composites. Elsevier Ltd.; 2019. doi:10.1016/B978-0-08-102292-4.00008-4. [Google Scholar] [CrossRef]
23. Hosseini SB, Gaff M, Li H, Hui D. Effect of fiber treatment on physical and mechanical properties of natural fiber-reinforced composites: a review. Rev Adv Mater Sci. 2023;62(1). doi:10.1515/rams-2023-0131. [Google Scholar] [CrossRef]
24. Mousavi SR, Zamani MH, Estaji S, Tayouri MI, Arjmand M, Jafari SH, et al. Mechanical properties of bamboo fiber-reinforced polymer composites: a review of recent case studies. J Mater Sci. 2022;57(5):3143–67. doi:10.1007/s10853-021-06854-6. [Google Scholar] [CrossRef]
25. Acosta R, Trujillo GC, Luddey J, Arévalo M. Synthesis and mechanical behavior of composite material reinforced with guadua fiber and with a polyurethane or polyester matrix. BioResources. 2021;16(4):8049–59. [Google Scholar]
26. Pramudi G, Raharjo WW, Ariawan D, Ubaidillah, Arifin Z. Utilization of bamboo fiber in the development of environmentally friendly composite–A review. IOP Conf Ser: Mater Sci Eng. 2021;1096(1):012038. doi:10.1088/1757-899x/1096/1/012038. [Google Scholar] [CrossRef]
27. Gholampour A, Ozbakkaloglu T. A review of natural fiber composites: properties, modification and processing techniques, characterization, applications. J Mater Sci. 2020;55(3):829–92. doi:10.1007/s10853-019-03990-y. [Google Scholar] [CrossRef]
28. Raharjo R, Bangun Darmadi D, Gapsari F, Setyarini PH, Widodo TD. Characterization of bromelain enzyme treated Bamboo petung fiber (BPF) for composite reinforcement. Case Stud Chem Environ Eng. 2024;9(1):100683. doi:10.1016/j.cscee.2024.100683. [Google Scholar] [CrossRef]
29. Pandey C, Goyat V, Goel S. Advances in materials and mechanical engineering. 2021. Available from: http://www.springer.com/series/11693. [Accessed 2024] [Google Scholar]
30. Tenazoa C, Savastano H, Charca S, Quintana M, Flores E. The effect of Alkali treatment on chemical and physical properties of Ichu and Cabuya fibers. J Nat Fibers. 2021;18(7):923–36. doi:10.1080/15440478.2019.1675211. [Google Scholar] [CrossRef]
31. dos Santos AJG, Ribeiro MM, Corrêa AC, Rodrigues JS, Silva DS, Junio RFP, et al. Morphological, chemical and mechanical properties of hybrid polyester composites reinforced with bamboo fibers and kaolin waste. J Mater Res Technol. 2024;30(45):1–15. doi:10.1016/j.jmrt.2024.03.003. [Google Scholar] [CrossRef]
32. Zidi S, Miraoui I, Jaballi S. Investigation of sisal fiber cords impact to improve the mechanical properties of plaster-based composites. Euro-Med J Environ Integr. 2024;9(2):579–90. doi:10.1007/s41207-024-00502-6. [Google Scholar] [CrossRef]
33. Kelkar BU, Shukla SR, Nagraik P, Paul BN. Structural bamboo composites: a review of processing, factors affecting properties and recent advances. Adv Bamboo Sci. 2023;3(6):100026. doi:10.1016/j.bamboo.2023.100026. [Google Scholar] [CrossRef]
34. Rajeshkumar G, Hariharan V, Scalici T. Effect of NaOH treatment on properties of phoenix Sp. fiber. J Nat Fibers. 2016;13(6):702–13. doi:10.1080/15440478.2015.1130005. [Google Scholar] [CrossRef]
35. Zhang K, Wang F, Liang W, Wang Z, Duan Z, Yang B. Thermal and mechanical properties of bamboo fiber reinforced epoxy composites. Polymers. 2018;8(6):608. doi:10.3390/polym10060608. [Google Scholar] [PubMed] [CrossRef]
36. Koohestani B, Darban AK, Mokhtari P, Yilmaz E, Darezereshki E. Comparison of different natural fiber treatments: a literature review. Int J Environ Sci Technol. 2019;16(1):629–42. doi:10.1007/s13762-018-1890-9. [Google Scholar] [CrossRef]
37. Jagadeesh P, Puttegowda M, Mavinkere Rangappa S, Siengchin S. A review on extraction, chemical treatment, characterization of natural fibers and its composites for potential applications. Polym Compos. 2021;42(12):6239–64. doi:10.1002/pc.26312. [Google Scholar] [CrossRef]
38. Nijdam S, Teunissen J, Ebert U. The physics of streamer discharge phenomena. Plasma Sources Sci Technol. 2020;29(10):103001. doi:10.1088/1361-6595/abaa05. [Google Scholar] [CrossRef]
39. Nadi A, Boukhriss A, Bentis A, Jabrane E, Gmouh S. Evolution in the surface modification of textiles: a review. Text Prog. 2018;50(2):67–108. doi:10.1080/00405167.2018.1533659. [Google Scholar] [CrossRef]
40. Gupta US, Dhamarikar M, Dharkar A, Chaturvedi S, Kumrawat A, Giri N, et al. Plasma modification of natural fiber: a review. Mater Today: Proc. 2020;43(3):451–7. doi:10.1016/j.matpr.2020.11.973. [Google Scholar] [CrossRef]
41. Di L, Zhang J, Zhang X, Wang H, Li H, Li Y, et al. Cold plasma treatment of catalytic materials: a review. J Phys D: Appl Phys. 2021;54(33):333001. doi:10.1088/1361-6463/ac0269. [Google Scholar] [CrossRef]
42. Gunwant D. Moisture resistance treatments of natural fiber-reinforced composites: a review. Compos Interfaces. 2024;32(1):1–69. doi:10.1080/09276440.2024.2303543. [Google Scholar] [CrossRef]
43. Anjumol KS, Sumesh KR, Vackova T, Hanna JM, Thomas S, Spatenka P. Effect of plasma treatment on the morphology, mechanical, and wetting properties of polyethylene/banana fiber composites. Biomass Convers Biorefin. 2023;23:100525. doi:10.1007/s13399-023-04884-5. [Google Scholar] [CrossRef]
44. Jahami A, Zeaiter N, Cheaib M. Reviewing the potential: a comprehensive review of natural fibers (NFs) in structural concrete and their multifaceted influences. In: Innovative infrastructure solutions. Springer International Publishing; 2024. vol. 9. doi:10.1007/s41062-024-01384-x. [Google Scholar] [CrossRef]
45. Rout S, Mallick B, Parida C. Cold plasma as a competent approach to treat solid fiber of Ichnocarpus frutescens: PIXE, XRD, Raman, FT-IR, and SEM analysis. Braz J Phys. 2023;53(2):1–15. doi:10.1007/s13538-023-01256-2. [Google Scholar] [CrossRef]
46. Habr J, Lenfeld P, Behalek L, Boruvka M, Brdlik P. Mechanical properties of biopolymer composite with natural fibers surface modified by low-temperature plasma. MM Sci J. 2020;2020(October):4007–14. doi:10.17973/MMSJ.2020_10_2020039. [Google Scholar] [CrossRef]
47. Sari PS, Thomas S, Spatenka P, Ghanam Z, Jenikova Z. Effect of plasma modification of polyethylene on natural fibre composites prepared via rotational moulding. Compos B: Eng. 2019;177(107344doi:10.1016/j.compositesb.2019.107344. [Google Scholar] [CrossRef]
48. Gerullis S, Pfuch A, Beier OI, Kretzschmar BSM, Beyer M, Fischer S. Plasma treatment of cellulose: investigation on molecular changes using spectroscopic methods and chemical derivatization. Cellulose. 2022;29(13):7163–76. doi:10.1007/s10570-022-04718-z. [Google Scholar] [CrossRef]
49. Gupta US, Tiwari S, Sharma U. Influence of low-pressure Ar plasma modification of Musa sapientum banana fibers on banana fiber reinforced epoxy composite. Compos Interfaces. 2023;30(8):877–98. doi:10.1080/09276440.2023.2179243. [Google Scholar] [CrossRef]
50. Shakir MH, Singh AK. An investigation on the mechanical properties, surface treatments and applications of abaca fibre-reinforced composites. Adv Mater Process Technol. 2023;1–26. doi:10.1080/2374068X.2023.2247701. [Google Scholar] [CrossRef]
51. Sawangrat C, Thipchai P, Kaewapai K, Jantanasakulwong K, Suhr J, Wattanachai P, et al. Surface modification and mechanical properties improvement of bamboo fibers using dielectric barrier discharge plasma treatment. Polymers. 2023;15(7):1711. doi:10.3390/polym15071711. [Google Scholar] [PubMed] [CrossRef]
52. Gupta US, Tiwari S. Mechanical and surface characterization of sisal fibers after cold glow discharge argon plasma treatment. Biomass Convers Biorefin. 2024;14(8):9163–77. doi:10.1007/s13399-022-03247-w. [Google Scholar] [CrossRef]
53. Gupta US, Tiwari S, Sharma U. Cold glow discharge nitrogen plasma treatment of banana and sisal fiber for mechanical and surface characterization improvement. J Indian Acad Wood Sci. 2023;20(1):37–50. doi:10.1007/s13196-023-00310-5. [Google Scholar] [CrossRef]
54. Luna P, Lizarazo-Marriaga J. An extraction methodology of Guadua angustifolia bamboo fibers. In: 6th Amazon & Pacific Green Materials Congress and Sustainable Construction Materials LAT-RILEM Conference; 2016; Cali, Colombia. [Google Scholar]
55. Luna P, Lizarazo-Marriaga J. Proposed method for determining cross-sectional area of Guadua angustifolia bamboo fibers. J Nat Fibers. 2017;16(2):253–62. doi:10.1080/15440478.2017.1414655. [Google Scholar] [CrossRef]
56. Adusumalli RB, Venkateshan KC, Kunchi C, Vadlamani SR. Tensile testing of single fibres. Procedia Structural Integrity. 2019;14:150–7. doi:10.1016/j.prostr.2019.05.020. [Google Scholar] [CrossRef]
57. Alves ME, Castro TV, da Fonseca O, de Andrade F, Dias Toledo Filho R. The effect of fiber morphology on the tensile strength of natural fibers. J Mater Res Technol. 2013;2(2):149–57. doi:10.1016/j.jmrt.2013.02.003. [Google Scholar] [CrossRef]
58. de Andrade F, Chawla N, Toledo Filho RD. Tensile behavior of high performance natural (sisal) fibers. Compos Sci Technol. 2008;68(15–16):3438–43. [Google Scholar]
59. Luna P, Lizarazo-Marriaga J, Mariño A. Guadua angustifolia bamboo fibers as reinforcement of polymeric matrices: an exploratory study. Constr Build Mater. 2016;116:93–7. doi:10.1016/j.conbuildmat.2016.04.139.doi:. [Google Scholar] [CrossRef]
60. Asres MA, Zerayohannes G, Zekaria A, Nuramo DA. Experimental study of Yushania alpina bamboo fiber. Mater Res Express. 2024;11(4):045101. doi:10.1088/2053-1591/ad3b67. [Google Scholar] [CrossRef]
61. Ramadevi P, Sampathkumar D, Srinivasa CV, Bennehalli B. Effect of alkali treatment on water absorption of single cellulosic abaca fiber. BioResources. 2012;7(3):3515–24. doi:10.15376/biores.7.3.3515-3524. [Google Scholar] [CrossRef]
62. Phong NT, Fujii T, Chuong B, Okubo K. Study on how to effectively extract bamboo fibers from raw bamboo and wastewater treatment. J Mater Sci Res. 2012;1(1):144–55. doi:10.5539/jmsr.v1n1p144. [Google Scholar] [CrossRef]
63. Benyahia A, Merrouche A, Rokbi M, Kouadri Z. Study the effect of alkali treatment of natural fibers on the mechanical behavior of the composite unsaturated polyester-fiber. Mech Indus. 2014;15(1):69–73. doi:10.1051/meca/2013082. [Google Scholar] [CrossRef]
64. Zhou Y, Long C, Huang J, Deng Z, Cao T. Effect of surface treatments on properties of natural fiber reinforced poly(lactic acid) composites. J Reinforced Plast Compos. 2013 Jun 10;32(18):1348–58. doi:10.1177/0731684413492452. [Google Scholar] [CrossRef]
65. Siakeng R, Jawaid M, Ariffin H, Salit MS. Effects of surface treatments on tensile, thermal and fibre-matrix bond strength of coir and pineapple leaf fibres with poly lactic acid. J Bionic Eng. 2018;15(6):1035–46. doi:10.1007/s42235-018-0091-z. [Google Scholar] [CrossRef]
66. Oushabi A, Sair S, Oudrhiri Hassani F, Abboud Y, Tanane O, El Bouari A. The effect of alkali treatment on mechanical, morphological and thermal properties of date palm fibers (DPFsstudy of the interface of DPF-Polyurethane composite. S Afr J Chem Eng. 2017;23:116–23. doi:10.1016/j.sajce.2017.04.005. [Google Scholar] [CrossRef]
67. Gholami M, Ahmadi MS, Tavanaie MA, Khajeh Mehrizi M. Effect of oxygen plasma treatment on tensile strength of date palm fibers and their interfacial adhesion with epoxy matrix. Sci Eng Compos Mater. 2018;25(5):993–1001. doi:10.1515/secm-2017-0102. [Google Scholar] [CrossRef]
68. Fan M, Dai D, Huang B. Fourier transform infrared spectroscopy for natural fibres. InTech. 2012:45–68. doi:10.5772/2659. [Google Scholar] [CrossRef]
69. Bozaci E, Sever K, Sarikanat M, Seki Y, Demir A, Ozdogan E, et al. Effects of the atmospheric plasma treatments on surface and mechanical properties of flax fiber and adhesion between fiber-matrix for composite materials. Compos B: Eng. 2013;45(1):565–72. doi:10.1016/j.compositesb.2012.09.042. [Google Scholar] [CrossRef]
70. Scalici T, Fiore V, Valenza A. Effect of plasma treatment on the properties of Arundo Donax L. leaf fibres and its bio-based epoxy composites: a preliminary study. Compos B: Eng. 2016. doi:10.1016/j.compositesb.2016.03.053. [Google Scholar] [CrossRef]
71. Wang F, Shao J, Keer LM, Li L, Zhang J. The effect of elementary fibre variability on bamboo fibre strength. Mater Design. 2015;75(2):136–42. doi:10.1016/j.matdes.2015.03.019. [Google Scholar] [CrossRef]
72. Espitía-Nery ME, Corredor-Pulido DE, Rodríguez-Ramírez NJ, Calderón-Bustos JN. Morphological and nanomechanical characterization of Guadua Angustifolia kunth fiber by means of SEM and AFM. Dyna. 2018;85(206):148–54. doi:10.15446/dyna.v85n206.70889. [Google Scholar] [CrossRef]
73. Luna P, Lizarazo-Marriaga J, Mariño A. Preliminary study on the compatibilization techniques of natural fibers as reinforcement of polymeric matrices. In: Sustainable Construction Materials and Technologies (SCMT4). Las Vegas, USA; 2016. p. 1–10. [Google Scholar]
74. Habibi MK, Lu Y. Crack propagation in bamboo’s hierarchical cellular structure. Sci Rep. 2014;4(5598):1–7. doi:10.1038/srep05598. [Google Scholar] [PubMed] [CrossRef]
75. Chou CS, Chen CY, Lin SH, Lu WH, Wu P. Preparation of TiO2/bamboo-charcoal-powder composite particles and their applications in dye-sensitized solar cells. Adv Powder Technol. 2015;26(3):711–7. doi:10.1016/j.apt.2014.12.013. [Google Scholar] [CrossRef]
76. Zhu J, Shan Y, Wang T, Sun H, Zhao Z, Mei L, et al. A hyperaccumulation pathway to three-dimensional hierarchical porous nanocomposites for highly robust high-power electrodes. Nat Commun. 2016;7:1–10. doi:10.1038/ncomms13432. [Google Scholar] [PubMed] [CrossRef]
77. Luna P, Mariño A, Lizarazo-Marriaga J, Beltrán O. Dry etching plasma applied to fique fibers: influence on their mechanical properties and surface appearance. Proc Eng. 2017;200:141–7. doi:10.1016/j.proeng.2017.07.021. [Google Scholar] [CrossRef]
78. Potluri S, Sangeetha K, Santhosh R, Nivas G, Mahendran R. Effect of low-pressure plasma on bamboo rice and its flour. J Food Process Preserv. 2018;e13846:1–7. doi:10.1111/jfpp.13846. [Google Scholar] [CrossRef]
79. Bogaerts A, Neyts E, Gijbels R, Van der Mullen J. Gas discharge plasmas and their applications. Spectrochim Acta–Part B Atom Spectr. 2002;57(4):609–58. doi:10.1016/S0584-8547(01)00406-2. [Google Scholar] [CrossRef]
Cite This Article
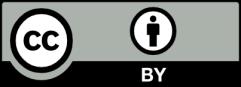
This work is licensed under a Creative Commons Attribution 4.0 International License , which permits unrestricted use, distribution, and reproduction in any medium, provided the original work is properly cited.