Open Access
REVIEW
A Review on Characteristics, Extraction Methods and Applications of Renewable Insect Protein
Research Centre of Biotechnology of the Russian Academy of Sciences, Moscow, 119071, Russia
* Corresponding Author: Adelya Khayrova. Email:
(This article belongs to the Special Issue: Special Issue in Celebration of JRM 10 Years)
Journal of Renewable Materials 2024, 12(5), 923-950. https://doi.org/10.32604/jrm.2024.050033
Received 25 January 2024; Accepted 18 April 2024; Issue published 17 July 2024
Abstract
Due to the expected rise in the world population, an increase in the requirements for quality and safety of food and feed is expected, which leads to the growing demand for new sources of sustainable and renewable protein. Insect protein is gaining importance as a renewable material for several reasons, reflecting its potential contributions to sustainability, resource efficiency, and environmental conservation. Some insect species are known to be able to efficiently convert organic waste into high-value products such as protein, requiring less land and water compared to traditional livestock. In addition, insect farming produces fewer greenhouse gas emissions, contributing to mitigating climate change. Insects are considered as a major potential alternative to animal or plant protein due to their many nutritional benefits, including high protein, mineral, and vitamin contents. On average, the protein content of insects ranges between 35% and 60% dry weight, which exceeds plant protein sources, such as cereal, soybeans, and lentils. As the acceptance of insect protein grows and technologies advance, the food and feed industries continue to explore and expand their applications, offering consumers diverse and sustainable protein choices. In this review, we discuss the recent findings relating to insect protein focusing on its characteristics, extraction methods, applications, and opportunities along with some trade-offs and uncertainties.Graphic Abstract
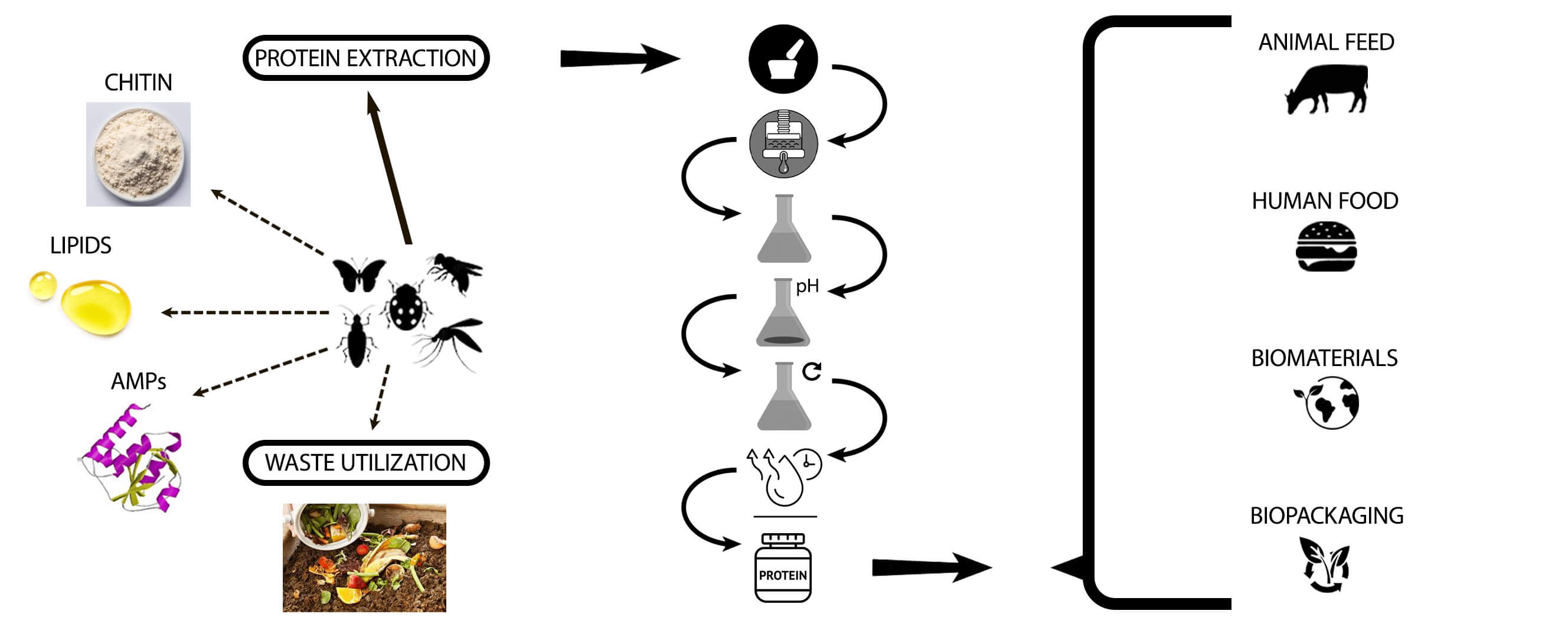
Keywords
As the world population continues to grow, coupled with escalating environmental pollution concerns, there is a rising need for eco-friendly and renewable protein sources. The ongoing struggle for land allocation between food crops and animal feed has persisted for some time. Nearly 50% of available arable land is already committed to feeding the global population, with a third of this land designated for livestock feed production [1]. It was estimated that livestock farming accounts for up to 19.6% of total anthropogenic greenhouse gas emissions [2], particularly, methane, contributing to global warming 28 times greater than carbon dioxide [3]. Meeting the future demand for animal feed and feed ingredients requires innovation towards more sustainable animal diets (Fig. 1). A wide range of novel protein sources including microalgae, macroalgae, and insects can be utilized for feeds.
Figure 1: Market forecast of animal feed market (in USD billion) according to research and markets (2023)
Insect rearing is a circular-economy process, resulting in waste being converted to an organic fertilizer [4,5], while insects serve as sources of value-added products [6,7]. In addition to environmental and socio-economic benefits, insects outperform animals such as poultry or cattle. They can be raised on smaller land areas, leading to a higher yield per hectare compared to conventional crops like soybeans, and emit fewer greenhouse gases and less ammonia per kilogram of meat produced in comparison to pigs and cattle [8]. Moreover, analysts estimate that alternative protein sources will account for 33% of global protein consumption by 2054, 11% of which will be insects [9].
In various regions across the globe, insects are already being employed in animal feed for aquaculture and poultry. For instance, many fish species feed on insects; therefore, their use in aquaculture comes naturally. Significant advantages have been linked to incorporating insects into animal nutrition. Insects can be cultivated using low-value agricultural by-products or organic waste from the food industry, resulting in high-quality protein that rivals fish and soybean meals in nutritional value [10]. Insects also serve as a rich source of amino acids, fatty acids, and micronutrients [11–13].
In addition to feed protein, insects represent a promising alternative protein source for the world’s growing population. Entomophagy, or human consumption of insects, has already been practiced in many parts of the world, especially in tropical regions. Multiple investigations have illustrated the various functional characteristics of insect proteins in food, including their capacity to create foam, serve as emulsifiers, and contribute to gel formation [14]. It was shown that insect proteins can either be used as food supplements to increase the nutritional quality of food products or as meat and dairy alternatives [15].
Another application of insect protein aims to replace traditional non-biodegradable and non-renewable petroleum sources with renewable and green alternatives, for example, in the production of bioplastics [16,17]. It is known that edible packaging can be prepared using biopolymers, such as carbohydrates, lipids, and proteins, which can be extracted from insects [18,19].
In this article, we review recent work on the use of industrially reared insects as a novel source of renewable protein considering the nutritional composition of insects, methods of insect protein extraction, and its future applications as well as prospects, opportunities, and potential challenges.
2 Insects: Characteristics and Nutritional Composition
Insects are the dominant life forms on the planet whose biomass exceeds the biomass of all invertebrates. Today insect farming is becoming increasingly popular due to the ability of insects to convert organic wastes into useful resources [20]. The nutritional composition of each insect varies depending on the species (Table 1). Some of the variables that could affect the nutritional content of insects include developmental stage, rearing techniques, diets, killing methods, and drying techniques. In addition, insect meal can be a source of high-value bioactive compounds such as peptides with immunostimulatory and anti-microbial effects and biopolymers (chitin, melanin) with potential effects on intestinal health and the immune system [6,21].
Due to the increased application of Black Soldier Fly (Hermetia illucens or BSF) larvae in organic waste utilisation (Fig. 2), BSF is becoming the focus of emerging research fronts [22]. The main benefit of H. illucens as well as other flies is its use as a tool for waste upcycling, while other insects are reared for feed application only. Furthermore, BSF is more advantageous than other insect species such as mealworms and crickets due to the higher feed conversion rate, short reproductive cycle, and high content of fat, protein, minerals, and vitamins [23,24]. Its survival rate and nitrogen and phosphorus composition are not significantly affected by a change in diet [25].
Figure 2: Final products of black soldier fly organic waste treatment (in percent)
• Proteins
Insect bodies are rich in protein–as a rule, the crude protein content of insect meals is high ranging from 42% to 63%, and is similar to soy meal [29]. Proteins’ suitability as animal feed relies on their amino acid composition. The high content of essential amino acids also ensures the high biodegradability of materials made out of insect proteins.
An imbalance in amino acids can lead to metabolic issues, necessitating the supplementation of specific amino acids [30]. For instance, fish require ten essential amino acids: arginine, histidine, isoleucine, leucine, lysine, methionine/cysteine, phenylalanine, threonine, tryptophan and valine, and H. illucens larvae contain all these essential amino acids [30]. The representation of essential amino acids in H. illucens is very similar to fish meal but the content is lower. As a result, H. illucens proteins can replace fish meal in aquaculture providing a more sustainable option. BSF larvae could also be used as feed in poultry and pig breeding due to their high protein content. For example, poultry requires 18%–20% crude protein, while pigs–13%–21% depending on their age [30]. These protein content requirements are hard to maintain by grain feed only.
It was shown that insect proteins satisfy the amino acid composition standards set by the World Health Organisation, demonstrating elevated levels of phenylalanine, tyrosine, tryptophan, lysine, and threonine [31]. For instance, one of the latest studies by Huang et al. [32] showed that protein extracted from H. illucens larvae exhibited a higher ratio of essential amino acids to total amino acids than that of egg protein. The amino acid analysis, therefore, indicates that protein extracted from H. illucens larvae can be utilized as an edible animal protein.
• Lipids
In contrast to the amino acid profile, the fatty acid content of insects can vary greatly with the diet. Therefore, it is possible to adjust the desired larval lipid composition depending on the intended purpose of the product. Usually insect fat content is higher at the larva and pupa stages, while at the adult stage, the fat content is relatively lower. Unlike the majority of animal fats, insect oil is liquid at room temperature, which accounts for a considerable number of polyunsaturated fatty acids (e.g., superworm, mealworm and cricket oil) [33,34]. For instance, in BSF they can be promoted when insects are fed on fish discards, coffee silverskin, and seaweeds [35].
Black soldier flies are rich in lipids (up to 49% on a dry matter basis) [29]. The quality of fats from H. illucens larvae is high and resembles the fatty acid content of products from plant sources, e.g., palm (kernel) and coconut oil. The high proportion of lauric acid (up to 60%), which is a raw material for many products in the cosmetic, cleaning, and detergent industries, is remarkable.
• Chitin
The mass production of insects can provide an alternative source for chitin and chitosan, its deacetylated derivative, as today the worldwide production of these biopolymers comes largely from crustacean shells. Chitin is present in insects at all stages of ontogenesis and plays an important role in their structure. In addition, in insects, chitin is associated with melanin, which is not found in crustaceans [6]. Melanin is acquired at later stages of insect development and can be extracted in the form of chitin-melanin complexes with enhanced biological activity either as a mechanical mixture (e.g., from bees [36]) or a covalent complex (e.g., from black soldier flies [7,37]).
• Micronutrients
Micronutrients (i.e., minerals and vitamins) are described as the nutrients required by an organism in trace amounts and play an important role in the nutritional value of feed. It was found that insects contain essential micronutrients such as iron, magnesium, calcium, phosphorous, selenium, zinc as well as vitamins A, B, D, E, and K [38].
BSF is considered to be the best source of minerals and calcium [24,39]. H. illucens larvae contain higher amounts of essential minerals compared to other insect species including iron, magnesium, calcium, copper, phosphorous, and zinc [40].
• Antimicrobial peptides (AMPs)
The greatest diversity of AMPs is found in insects [41,42]. Insects have a well-developed innate immune system with cellular and humoral defense responses, involving the production of AMPs secreted into the hemolymph [43,44]. AMPs are a class of small peptides that widely exist in nature and are an important part of the innate immune system among living organisms. They have a wide range of inhibitory effects against different microorganisms such as bacteria, fungi, parasites, and viruses [45]. AMPs, acting as potent antibiotics or fungicides, primarily target the cell envelope, notably the cell membrane. They also impact intracellular microbial targets, ultimately causing cell death [46–48]. BSF is a very resilient organism, and its larvae are fed on a variety of decomposing organic substrates typically inhabited by a range of microorganisms. Therefore, it can be expected that H. illucens larvae express AMPs and other substances possessing antibiotic activity in order to survive in these unfavorable conditions [30].
3 Methods for Obtaining Protein
There are a limited number of studies devoted to the extraction of insect proteins and their characterization. To date, the most prevalent method for protein extraction in insects is wet fractionation; however, dry fractionation can also be applied.
In the literature, protein isolation is usually performed by the following steps: homogenization, defatting, protein solubilization, isoelectric precipitation of the proteins, protein resolubilisation and drying [49]. Nevertheless, the parameters for each step may vary depending on the insect and its life stage and should be adjusted accordingly.
The aim of the homogenization process is to convert insects to a smaller particle size to increase the surface area between the insect particles and the extraction solvent. This leads to a more efficient process of protein extraction.
Defatting may be carried out by a solvent extraction step. Different lipid removal processes have been described in the literature such as petroleum ether [50–53], hexane [54,55], ethanol or hexane: isopropanol (3:2 (v/v)) [56], supercritical CO2 [57]. However, the use of organic solvents during the lipid removal step may result in losses of protein due to their affinity for the solvent. An alternative defatting method was applied to edible insect homogenates consisting of centrifugation of insect homogenates at 4°C and removal of the upper layer of lipid fraction from the supernatant [57].
The prevailing technique involves combining alkaline solubilization with isoelectric precipitation, although certain studies may choose either alkaline or acid solubilization separately. To summarise, in the alkaline solubilisation coupled with the isoelectric precipitation method, insect meal is initially solubilised under alkaline conditions to dissolve proteins. Subsequently, centrifugation is employed to separate the soluble proteins, with the resulting solution collected while discarding the pellet. Following this, the pH is adjusted to the isoelectric point to induce protein precipitation.
According to the literature, either water-soluble or non-water-soluble proteins can be isolated from insects. To obtain water-soluble proteins, alkaline extraction procedure is performed: the insect homogenate is adjusted to pH values higher than 7.0 to solubilize proteins in the aqueous phase [58–62]. This creates a high overall charge, which results in electrostatic repulsion allowing proteins to be soluble in aqueous phases.
To extract non-water soluble proteins, a centrifugation step on insect homogenates is conducted, and the pellet containing the non-water soluble protein fraction is retained [63,64]. For instance, the collection of various protein fractions from B. mori pupae was described by a sequential extraction using water, 5% NaCl, 0.1 M NaOH, and 70% ethanol solutions [65]. Very few studies, however, have characterized obtained insect protein isolates. Also, specific methods of extracting protein isolates are hard to scale-up and do not appear economically viable for commercial feed applications.
Alternatively, enzymatic hydrolysis can be carried out either directly on insect homogenates or insect protein isolates to generate bioactive peptides (BAPs) or to improve the techno-functional properties of the proteins. In some cases, proteins may be denatured by physical means, e.g., ultrasound prior to enzymatic hydrolysis [52,66].
In order to generate protein-derived BAPs, mammalian digestive enzymes and microbial enzymes are commonly used. The method of sequential hydrolysis with pepsin, trypsin and chymotrypsin (simulated gastrointestinal digestion) was applied to hydrolyze various insect species such as B. mori larvae and pupae [53,67], S. littoralis [63,64], adult A. annulipes, S. gregaria and L. migratoria, B. dubia, G. portentosa as well as T. molitor and Z. morio [68,69].
Plant-derived enzyme preparations during insect protein hydrolysis are rarely used. An example is papain hydrolysis of B. mori chrysalises [57]. Some papers do not disclose the origin of the enzyme preparation.
Another study explores a range of commercial enzymes of vegetal (papain), bacterial (PBL, dispase) and animal (pepsin, trypsin, pancreatin) origin to obtain protein hydrolysates from Alphitobius diaperinus and Hermetia illucens larvae [70].
The potential of microbial (lactic) fermentation of H. illucens biomasses with two different strains (L. rhamnosus and L. plantarum) is studied by Luparelli et al. [71].
Several protein extraction techniques can be applied including chemical and enzymatic methods (Table 2). Most of the studies focus on protein extraction from H. illucens larvae. In the study by Mshayisa et al. [61] two chemical techniques, alkaline solution and isoelectric precipitation (using 1 M NaOH and 1 M HCl) and alkaline extraction (using 1 M N NaOH), were investigated to extract protein concentrates from defatted H. illucens larval flour. The results indicated that alkaline and acid precipitation extractions of H. illucens larval concentrates led to improved nutritional and functional properties. The crude protein of H. illucens larval protein concentrate obtained by alkaline and acid precipitation was 73.35%, whereas for alkaline extraction the value was 68.47%.
Batish et al. [72] examined two methods: chemical extraction using 1 M NaOH and precipitation with 10% trichloroacetic acid solution in acetone as well as enzymatic hydrolysis with Alcalase, papain and pepsin. Enzymatic hydrolysis lasted for 2 h, and each mixture’s enzyme was deactivated by heating the solution in a water bath afterward. The heated suspension underwent centrifugation resulting in three distinct phases: a semisolid bottom phase containing insoluble protein and chitin, an intermediate liquid phase with protein hydrolysates, and a top liquid with the lipid fraction [72]. The intermediate supernatant phase was isolated and subjected to freeze-drying. The effects of both methods on the functional properties, antioxidant activity, amino acid composition, and protein structure of H. illucens larval protein were evaluated. Even though enzymatic hydrolysis offered a sustainable processing method, it reduced the functional properties in H. illucens hydrolysates.
Firmansyah et al. [73] carried out enzymatic hydrolysis of defatted H. illucens larvae using a bromelain enzyme. Defatted H. illucens larvae were dissolved in a phosphate buffer solution, followed by the addition of the enzyme. The hydrolysis reaction occurred in a water bath shaker for 3, 13.5 or 24 h, with termination of the reactions achieved through sample heating. For remaining H. illucens larvae samples, complete hydrolysis was achieved by applying 6 M HCl at 110°C for 24 h to determine the total free amino acid content [73]. Protein hydrolysate from H. illucens larvae was separated via centrifugation and freeze-dried. The authors reported that the obtained protein hydrolysate had a yield of 10.70% (on a weight basis), while the protein concentration varied between 240–310 μg/mL.
As the acceptance of insect protein grows and technologies advance, the food and feed industries continue to explore and expand their uses, offering consumers diverse and sustainable protein choices. The application of insect proteins will be dealt with in the following section.
Insect protein is commonly used as an ingredient in animal feed for poultry, livestock, and aquaculture. It provides a sustainable and protein-rich alternative to traditional feed sources, contributing to healthier and more efficient animal growth. Insect protein is also increasingly incorporated into pet food formulations, offering a nutrient-dense and eco-friendly protein source for companion animals.
As a rule, whole or powdered dried insects are applied as a feed source for animals. In some cases, the biomass can be separated by physical methods such as pressing or chemical methods such as extraction with organic solvents. These treatments yield protein and lipid fractions, and in certain instances, a chitin fraction [74]. The isolated protein can be subjected to a finer grinding and drying by convection, obtaining a fat-free meal that is used asalternative to fish meal [75]. However, this treatment can lead to the destruction of part of the protein by prolonged exposure to heat as well as the presence of chitin in the final product [76].
Table A1 summarises the literature data on insects investigated as a source of protein with a potential application in animal diets. Most studies (approximately 46%) apply insects in a partially defatted form, obtained through mechanical removal of fats by continuous screw press [77–79] and supplied from a commercial source. In some studies, chemical treatments using Soxhlet [80], solvent, e.g., ethyl alcohol [81,82] or enzymatic hydrolysis, e.g., using proteases and chitinases [83] were performed, which are likely to result in higher efficiency of fat (and other impurities) removal but can incur additional economic costs.
Dried powdered form (approximately 34%) is the second most frequently used form of insects for feed [84–86].
Whole dried insects (approximately 20%) are less often applied, however, still result in a significant number of studies, particularly in birds [87,88]. The least frequent form of application is live insects [89].
Insects are mostly used as a feed additive to animal diets [79,86,90–92] rather than a single component of feed and usually replace fish meal and soy protein. The application of insect proteins as a feed source will be dealt with in the following section.
4.1.1 Inclusion of Insects in Animal Diet
As demonstrated in Section 2, the nutritional properties of insects are very high, and they can successfully substitute for many traditional ingredients used in the production of feed such as fish meal and soy. Black soldier flies Hermetia illucens, yellow mealworms Tenebrio molitor, common housefly Musca domestica, and silkworms Bombyx mori have been recognised as major species for the commercial production of feed [25,93].
The highest proportion of research articles are devoted to the investigation of H. illucens as a future feed for animals, which could be explained by the increased use of BSF technology to upcycle agriculture and food industry waste into high-quality insect ingredients (Table 2). However, the majority of studies examine the partially defatted or dried powdered larvae as a feed source for animals rather than extracting a protein fraction. It should be noted that insects contain other substances such as lipids, chitin and melanin which was discussed earlier in Section 2. These can affect animal health and digestion (e.g., chitin) and should be taken into account.
The second largest proportion of articles is dedicated to the yellow mealworm (Tenebrio molitor). T. molitor is an insect species with fast growth and reproduction rates feeding on bread and grains. T. molitor has a high protein content (47%–63%) and a moderate lipid content (30%–41%), and its nutrient composition matches with animal requirements [29,94,95].
T. molitor is usually applied at the larval stage, H. illucens–at larval, prepupal and rarely pupal stages, while B. mori is used as pupae, G. sigillatus and B. lateralis–as imagoes (Table 2).
A large number of studies investigated insect protein in the diets of fish [96–98], poultry [87,89,99], pigs [100,101], and pets [102–104]. These findings demonstrate that insects can be applied as a universal feed source for various animals.
Insect-derived protein powders are gaining popularity as a nutritious and sustainable source of protein for human consumption. Some examples include nutritional supplements, functional foods, sports nutrition and bakery/confectionary products. Most of the papers focus on insect proteins as either supplements of food products to increase their nutritional quality or the reformulation of products aimed at the reduction or replacement of meat or milk proteins [105].
Table 3 summarises some key applications of insect protein in the food industry. In the largest proportion of studies (approximately 67%) insects are dried and ground into a fine powder. This insect powder or flour can be blended with traditional flours to create bakery goods or pasta with enhanced nutritional profiles [106–109].
Whole insects or defatted insects (e.g., T. molitor, Z. morio) can be incorporated into a wide range of processed foods, including burgers, sausages contributing to their protein content or used as meat substitutes [110–112].
Several studies have been conducted to assess the safety and food safety of insect protein, ensuring its acceptability and compliance for human consumption [128,129]. In a recent comprehensive review by J.C. Ribeiro et al. the authors delved into scientific advancements related to the allergic risks associated with consuming insects as food [129]. The study identified two primary risk groups susceptible to developing food allergies linked to insect consumption: individuals allergic to crustaceans and those consistently exposed to edible insects. Cross-reactivity, predominantly mediated through tropomyosin, was noted as a potential cause for allergic reactions, with T. molitor tropomyosin capable of eliciting allergic responses in animal models [130]. Moreover, the research demonstrated that individuals consistently exposed to T. molitor may become sensitized and subsequently develop a food allergy to this insect [131]. The study also identified different allergens (larval cuticle protein and cockroach allergen-like protein) depending on the route of sensitization [132]. These findings contribute valuable insights to enhance our understanding of the safety aspects surrounding insect protein consumption.
The manufacturing of plastics, particularly from petroleum-based sources is known to contribute to environmental, health, and sustainability concerns, leading to climate change. Hence, bioplastics, derived from renewable biomass sources, offer several benefits compared to traditional petroleum-based plastics. Insect proteins are explored for their potential in developing biodegradable plastics and bio-packaging.
Zhang et al. [133] explored the locust (Locusta migratoria) as a new protein source to develop edible film by solvent casting. Dehydrated adults of L. migratoria locusts were powdered and defatted by hexane. Protein extraction was performed by the standard alkaline dispersion and acid precipitation method. The authors reported that the optimal range of glycerol for locust protein film formation was 30%–50%. The FTIR and XRD analyses showed that there was good compatibility between protein and glycerol due to the hydrogen bonding interactions. The results revealed that the barrier and mechanical properties of edible insect films were similar to the unmodified cereal protein, which highlights the potential use of insect protein in food packaging and the future development of green biomaterials.
Zhang et al. [134] also developed a novel antimicrobial edible packaging based on a grasshopper protein/soy protein isolate blend by solution casting using xylose as a crosslinker and cinnamaldehyde as an antimicrobial agent. Insect protein was extracted by the alkaline dispersion and acid precipitation method. The authors stated that this novel insect protein-based composite film can be applied as a potential edible antimicrobial film for active packaging.
Qoirinisa et al. [135] produced edible films using grasshopper gelatine extracted by the alkaline dispersion and acid precipitation method using NaOH and HCl, respectively. The extraction process produced 55.2% raw gelatine powder from the dry weight of grasshoppers. The results revealed high foaming properties and high solubility of insect protein. It was concluded that insect protein can be applied in the manufacturing of edible films as environmentally friendly packaging.
Another study [136] explored the potential of the black soldier fly reared on food waste to develop bio-packaging film for food products. Protein (42%–44%) and chitin (9%–11%) contents of H. illucens prepupae were determined. The film was produced with 4% (w/v) black soldier fly prepupae flour in 1% (w/v) chitosan solution. The physical and mechanical properties of insect bio-packaging such as tensile strength, antioxidant activity, and water activity were evaluated. The study concluded that black soldier fly prepupae were a promising source for producing sustainable bio-packaging films for the food sector.
These diverse applications highlight the versatility and potential of insect protein across various sectors, providing solutions to challenges related to food security, environmental sustainability, and resource efficiency.
Despite the aforementioned advantages and predicted increased demand for insect protein as a potential food and feed source, little is known about possible risks. Several studies and reviews have pointed out potential challenges. These include concerns about chemical and microbiological safety, allergenicity in animals and humans, deficiencies in specific amino acids, and issues related to digestibility and taste, most of which are not yet fully characterized or understood [11,137–140].
Before incorporating insects into food products and animal feed, it is crucial to establish their safety. The growing concern is the accumulation of heavy metals in the feed, which poses a significant food safety risk, potentially harming animals and endangering human health by introducing toxic substances into the food chain [141–143]. This could be explained by the presence of chitin, which is an effective sorbent. As insects can grow on agricultural waste materials containing chemical contaminants such as pesticides and veterinary drug residues, it is important to evaluate the safety of insects in novel foods and animal feeds.
The breeding and rearing of insects might also have an impact on biodiversity, thus, the introduction of non-native species should be subjected to a risk assessment procedure [144]. Considering the utilization of suitable technologies for managing, processing, and storing insects after harvesting is essential. Such technologies may need adjustments to accommodate this new ingredient while ensuring the same level of safety in terms of identifying hazards, assessing risks, and tracing origins, all without compromising efficiency and product quality. Up-scaling of insect-rearing facilities into economically viable businesses is one of the major challenges.
Another issue is related to the reactions of farmers, stakeholders, and consumers towards the use of insects, which are likely to determine the future success of using insect-based feed for different species and acceptance of foods obtained from animals raised on insect-based feed. Several studies have investigated the acceptance of insects in animal feed and food products [145–147].
Achieving cost competitiveness in the commercialization of insect proteins presents a complex challenge that varies across different geographical regions. The initial investments in infrastructure, technology, and research for insect farming can be substantial, impacting the overall economic viability. Scaling up production to meet market demands without compromising quality further intensifies the challenge, requiring optimized breeding practices, efficient feed formulations, and automation technologies. Balancing these factors is crucial to achieving a competitive cost structure compared to traditional protein sources. Additionally, fluctuations in market prices for feedstocks and energy prices, uncertainties in regulatory compliance costs, and variations in consumer acceptance can impact the overall cost dynamics. Successful commercialization hinges on strategic investments, continuous optimization, and collaborative efforts across the value chain to enhance the cost-effectiveness of insect protein production and make it a sustainable and economically viable alternative in the global protein market [148].
An additional concern is the legal regulation of insects for food and feed applications, which differs across the world. For instance, in the European Union (EU), insects that are bred for either human consumption or animal feed are categorized as ‘farmed animals’ according to Regulation (EC) No. 1069/2009. This classification has specific implications for obtaining permission to use a feed (organic resource or substrate) for these farmed animals. Across the EU, all feed, including insects, must adhere to general rules, ensuring safety and preventing direct adverse effects on the environment or animal welfare, as stipulated in Regulation (EC) No. 767/2009 and Regulation (EC) No. 178/2002. Additionally, there are regulations governing feed hygiene (Regulation (EC) No. 183/2005) and setting maximum limits for certain undesirable substances in animal feed (Directive, 2002/32/EC).
The rapid development of research on the use of insect-based feed ingredients for farmed animals over the past decade led to the authorization of insect-processed animal protein in feed for farmed fish in 2017 (Regulation (EU) 2017/893). Currently, 7 insect species, including black soldier fly, common housefly, yellow mealworm, lesser mealworm, house cricket, banded cricket and field cricket, are permitted as feed for aquaculture, poultry, and swine animals in the EU. Nevertheless, there are no restrictions on the use of fats derived from insects. In some countries, e.g., China or South Korea, no limitation is applied.
Nowadays, insects are being explored intensively as future foods, and the EU has recently authorized four insect species intended to be marketed as a snack or as a food ingredient, in a number of food products: Tenebrio molitor larva (Regulation (EU) 2021/882 and Regulation (EU) 2022/169); Locusta migratoria (Regulation (EU) 2021/1975); Acheta domesticus (Regulation (EU) 2022/188); Alphitobius diaperinus (Regulation (EU) 2023/58).
6 Conclusions and Future Perspectives
In this review, insects were considered a novel source of renewable protein. The conventional natural resources used to produce food and feed are not enough to satisfy the growing demand for proteins due to the expected rise in world population and urbanization as well as the limited availability of land, fertilizers, energy, and water. Furthermore, the global prices of fish meal, maize, and soybean meal have significantly increased over the last few decades. The use of insects in food and feed has become a potential solution to these constraints.
This review of literature data demonstrates the use of insects as high-quality feed, food additives, and biop-ackaging materials. The majority of studies have focused on whole insects or defatted powdered meals when referring to insect protein, leaving behind chitin and other compounds present in insects, which can affect the properties of the final product.
Importantly, in the face of climate change, a shift to insect-based food and feed offers a sustainable solution for recycling biowaste and reducing greenhouse gas emissions. In addition, insect farming can create new jobs contributing to improved food security and income by providing a cheaper source of feed and organic fertilizer, reducing food-feed competition, and diversifying income-generating opportunities for insect-producing farmers and other actors.
Even though the potential of insects in food and feed is clear, the question of how to effectively rear and process insects into commercial products remains a challenge. The chemical and microbial safety, allergenicity, amino acid deficiency, digestibility, and taste of insect proteins are not yet fully understood. The safety assessment of insects in novel foods and feeds is still incomplete. Additionally, insect farming may have an impact on biodiversity. The acceptance of insects by farmers, stakeholders, and consumers may influence the future success of insect feed. Therefore, further research on protein extraction from insects is required. As the nutrient composition of insects varies with the type of insect, its stage in the life cycle, rearing conditions, and the extraction method should be evaluated and adjusted accordingly.
Acknowledgement: None.
Funding Statement: The authors received no specific funding for this study.
Author Contributions: The authors confirm contribution to the paper as follows: study conception and design: A. Khayrova, S. Lopatin, V. Varlamov; data collection, analysis and interpretation of results, draft manuscript preparation: A. Khayrova. All authors reviewed the results and approved the final version of the manuscript.
Availability of Data and Materials: Not applicable.
Conflicts of Interest: The authors declare that they have no conflicts of interest to report regarding the present study.
References
1. Thornton P, Gurney-Smith H, Wollenberg E. Alternative sources of protein for food and feed. Curr Opin Environ Sustain. 2023;62:101277. doi:10.1016/j.cosust.2023.101277. [Google Scholar] [CrossRef]
2. Xu X, Sharma P, Shu S, Lin TS, Ciais P, Tubiello FN, et al. Global greenhouse gas emissions from animal-based foods are twice those of plant-based foods. Nat Food. 2021;2(9):724–32. doi:10.1038/s43016-021-00358-x. [Google Scholar] [PubMed] [CrossRef]
3. Grossi G, Goglio P, Vitali A, Williams AG. Livestock and climate change: impact of livestock on climate and mitigation strategies. Anim Front. 2019;9(1):69–76. [Google Scholar] [PubMed]
4. Sheppard DC, Tomberlin JK, Joyce JA, Kiser BC, Sumner SM. Rearing methods for the black soldier fly (Diptera: Stratiomyidae). J Med Entomol. 2002;39(4):695–8. [Google Scholar] [PubMed]
5. Rumpold BA, Schlüter OK. Potential and challenges of insects as an innovative source for food and feed production. Innov Food Sci Emerg Technol. 2013;17:1–11. doi:10.1016/j.ifset.2012.11.005. [Google Scholar] [CrossRef]
6. Khayrova A, Lopatin S, Varlamov V. Obtaining chitin, chitosan and their melanin complexes from insects. Int J Biol Macromol. 2021;167:1319–28. doi:10.1016/j.ijbiomac.2020.11.086. [Google Scholar] [PubMed] [CrossRef]
7. Khairova AS, Lopatin SA, Varlamov VP, Bogdanova SA, Shigabieva YA, Knyazev AA. Chitosan-melanin polymer complex: a promising ingredient in emulsion compositions. Polym Sci. 2022;15(2):295–9. [Google Scholar]
8. Oonincx DGAB, van Itterbeeck J, Heetkamp MJW, van den Brand H, van Loon JJA, van Huis A. An exploration on greenhouse gas and ammonia production by insect species suitable for animal or human consumption. PLoS One. 2010;5(12):1–7. [Google Scholar]
9. Vinci G, Prencipe SA, Masiello L, Zaki MG. The application of life cycle assessment to evaluate the environmental impacts of edible insects as a protein source. Earth. 2022;3(3):925–38. [Google Scholar]
10. Kee PE, Cheng YS, Chang JS, Yim HS, Tan JCY, Lam SS, et al. Insect biorefinery: a circular economy concept for biowaste conversion to value-added products. Environ Res. 2023;221:115284. doi:10.1016/j.envres.2023.115284. [Google Scholar] [PubMed] [CrossRef]
11. Rumpold BA, Schlüter OK. Nutritional composition and safety aspects of edible insects. Mol Nutr Food Res. 2013;57(5):802–23. [Google Scholar] [PubMed]
12. Kierończyk B, Rawski M, Mikołajczak Z, Homska N, Jankowski J, Ognik K, et al. Available for millions of years but discovered through the last decade: insects as a source of nutrients and energy in animal diets. Anim Nutr. 2022;11:60–79. [Google Scholar] [PubMed]
13. Gasco L, Biancarosa I, Liland NS. From waste to feed: a review of recent knowledge on insects as producers of protein and fat for animal feeds. Curr Opin Green Sustain Chem. 2020;23:67–79. doi:10.1016/j.cogsc.2020.03.003. [Google Scholar] [CrossRef]
14. Queiroz LS, Nogueira Silva NF, Jessen F, Mohammadifar MA, Stephani R, Fernandes de Carvalho A, et al. Edible insect as an alternative protein source: a review on the chemistry and functionalities of proteins under different processing methods. Heliyon. 2023;9(4):e14831. [Google Scholar] [PubMed]
15. Lee HJ, Yong HI, Kim M, Choi YS, Jo C. Status of meat alternatives and their potential role in the future meat market—A review. Asian-Australasian J Anim Sci. 2020;33(10):1533–43. [Google Scholar]
16. Shlush E, Davidovich-Pinhas M. Bioplastics for food packaging. Trends Food Sci Technol. 2022;125:66–80. doi:10.1016/j.tifs.2022.04.026. [Google Scholar] [CrossRef]
17. Zhao X, Wang Y, Chen X, Yu X, Li W, Zhang S, et al. Sustainable bioplastics derived from renewable natural resources for food packaging. Matter. 2023;6(1):97–127. doi:10.1016/j.matt.2022.11.006. [Google Scholar] [CrossRef]
18. Weng S, Marcet I, Rendueles M, Díaz M. Insect-derived materials for food packaging—A review. Food Packag Shelf Life. 2023;38:101097. doi:10.1016/j.fpsl.2023.101097. [Google Scholar] [CrossRef]
19. Trajkovska Petkoska A, Daniloski D, D’Cunha NM, Naumovski N, Broach AT. Edible packaging: sustainable solutions and novel trends in food packaging. Food Res Int. 2021;140:109981. doi:10.1016/j.foodres.2020.109981. [Google Scholar] [PubMed] [CrossRef]
20. Castillo C, Hernández J. Insects in ruminant nutrition as an urgent measure in the light of the scarcity of raw feedstock. Res Vet Sci. 2023;155:124–5. [Google Scholar] [PubMed]
21. Ushakova N, Dontsov A, Sakina N, Bastrakov A, Ostrovsky M. Antioxidative properties of melanins and ommochromes from black soldier fly Hermetia illucens. Biomolecules. 2019;9(9):408. [Google Scholar] [PubMed]
22. Lu S, Taethaisong N, Meethip W, Surakhunthod J, Sinpru B, Sroichak T, et al. Nutritional composition of black soldier fly larvae (Hermetia illucens L.) and its potential uses as alternative protein sources in animal diets: a review. Insects. 2022;13(9):1–17. [Google Scholar]
23. Abd El-Hack ME, Shafi ME, Alghamdi WY, Abdelnour SA, Shehata AM, Noreldin AE, et al. Black soldier fly (Hermetia illucens) meal as a promising feed ingredient for poultry: a comprehensive review. Agriculture. 2020;10(8):1–31. [Google Scholar]
24. Spranghers T, Ottoboni M, Klootwijk C, Ovyn A, Deboosere S, de Meulenaer B, et al. Nutritional composition of black soldier fly (Hermetia illucens) prepupae reared on different organic waste substrates. J Sci Food Agric. 2017;97(8):2594–600. [Google Scholar] [PubMed]
25. Oonincx DGAB, van Broekhoven S, van Huis A, van Loon JJA. Feed conversion, survival and development, and composition of four insect species on diets composed of food by-products. PLoS One. 2015;10(12):e0144601. doi:10.1371/journal.pone.0144601. [Google Scholar] [PubMed] [CrossRef]
26. Khan S, Khan RU, Alam W, Sultan A. Evaluating the nutritive profile of three insect meals and their effects to replace soya bean in broiler diet. J Anim Physiol Anim Nutr. 2018;102(2):e662–8. [Google Scholar]
27. Zhang AJ, Qin QL, Zhang H, Wang HT, Li X, Miao L, et al. Preparation and characterisation of food-grade chitosan from housefly larvae. Czech J Food Sci. 2011;29(6):616–23. doi:10.17221/100/2010-CJFS. [Google Scholar] [CrossRef]
28. Battampara P, Nimisha Sathish T, Reddy R, Guna V, Nagananda GS, Reddy N, et al. Properties of chitin and chitosan extracted from silkworm pupae and egg shells. Int J Biol Macromol. 2020;161:1296–304. doi:10.1016/j.ijbiomac.2020.07.161. [Google Scholar] [PubMed] [CrossRef]
29. Makkar HPS, Tran G, Heuzé V, Ankers P. State-of-the-art on use of insects as animal feed. Anim Feed Sci Technol. 2014;197:1–33. doi:10.1016/j.anifeedsci.2014.07.008. [Google Scholar] [CrossRef]
30. Müller A, Wolf D, Gutzeit HO. The black soldier fly, Hermetia illucens—A promising source for sustainable production of proteins, lipids and bioactive substances. Zeitschrift fur Naturforsch-Sect C J Biosci. 2017;72(9–10):351–63. [Google Scholar]
31. van Huis A. Edible insects are the future? Proc Nutr Soc. 2016;75(3):294–305. [Google Scholar] [PubMed]
32. Huang W, Wang C, Chen Q, Chen F, Hu H, Li J, et al. Physicochemical, functional, and antioxidant properties of black soldier fly larvae protein. J Food Sci. 2023;89:259–75. [Google Scholar] [PubMed]
33. Womeni HM, Linder M, Tiencheu B, Mbiapo FT, Villeneuve P, Fanni J, et al. Oils of insects and larvae consumed in Africa: potential sources of polyunsaturated fatty acids. OCL-Ol Corps Gras Lipides. 2009;16(4):230–5. [Google Scholar]
34. Jayanegara A, Gustanti R, Ridwan R, Widyastuti Y. Fatty acid profiles of some insect oils and their effects on in vitro bovine rumen fermentation and methanogenesis. Ital J Anim Sci. 2020;19(1):1311–8. doi:10.1080/1828051X.2020.1841571. [Google Scholar] [CrossRef]
35. Rodrigues DP, Ameixa OMCC, Vázquez JA, Calado R. Improving the lipid profile of black soldier fly (Hermetia illucens) larvae for marine aquafeeds: current state of knowledge. Sustainability. 2022;14(11):6472. [Google Scholar]
36. Nemtsev SV, Zueva OY, Khismatullin MR, Albulov AI, Varlamov VP. Isolation of chitin and chitosan from honeybees. Appl Biochem Microbiol. 2004;40(1):39–43. [Google Scholar]
37. Khayrova A, Lopatin S, Varlamov V. Obtaining and study of physicochemical properties of chitin/chitosan-melanin complexes from Hermetia illucens. J Phys Conf Ser. 2021;1942(1):012003. [Google Scholar]
38. Liu X, Chen X, Wang H, Yang Q, Ur Rehman K, Li W, et al. Dynamic changes of nutrient composition throughout the entire life cycle of black soldier fly. PLoS One. 2017;12(8):1–21. [Google Scholar]
39. Mohan K, Rajan DK, Muralisankar T, Ganesan AR, Sathishkumar P, Revathi N. Use of black soldier fly (Hermetia illucens L.) larvae meal in aquafeeds for a sustainable aquaculture industry: a review of past and future needs. Aquaculture. 2022;553:738095. [Google Scholar]
40. Dierenfeld ES, King J. Digestibility and mineral availability of phoenix worms, Hermetia illucens, ingested by mountain chicken frogs, Leptodactylus fallax. J Herpetol Med Surg. 2008;18(3):100–5. [Google Scholar]
41. Eleftherianos I, Zhang W, Heryanto C, Mohamed A, Contreras G, Tettamanti G, et al. Diversity of insect antimicrobial peptides and proteins—A functional perspective: a review. Int J Biol Macromol. 2021;191:277–87. doi:10.1016/j.ijbiomac.2021.09.082. [Google Scholar] [PubMed] [CrossRef]
42. Hadj Saadoun J, Sogari G, Bernini V, Camorali C, Rossi F, Neviani E, et al. A critical review of intrinsic and extrinsic antimicrobial properties of insects. Trends Food Sci Technol. 2022;122:40–8. doi:10.1016/j.tifs.2022.02.018. [Google Scholar] [CrossRef]
43. Bulet P, Hetru C, Dimarcq JL, Hoffmann D. Antimicrobial peptides in insects; structure and function. Dev Comp Immunol. 1999;23(4–5):329–44. doi:10.1016/S0145-305X(99)00015-4.. [Google Scholar] [PubMed] [CrossRef]
44. Hoffmann JA, Reichhart JM. Drosophila innate immunity: an evolutionary perspective. Nat Immunol. 2002;3(2):121–6. doi:10.1038/ni0202-121. [Google Scholar] [PubMed] [CrossRef]
45. Huan Y, Kong Q, Mou H, Yi H. Antimicrobial peptides: classification, design, application and research progress in multiple fields. Front Microbiol. 2020;11:1–21. [Google Scholar]
46. Shai Y. Mode of action of membrane active antimicrobial peptides. Biopolymers. 2002;66(4):236–48. [Google Scholar] [PubMed]
47. Lohner K. New strategies for novel antibiotics: peptides targeting bacterial cell membranes. Gen Physiol Biophys. 2009;28(2):105–16. [Google Scholar] [PubMed]
48. Melo MN, Ferre R, Castanho MARB. Antimicrobial peptides: linking partition, activity and high membrane-bound concentrations. Nat Rev. Microbiol. 2009;7:245–50. [Google Scholar] [PubMed]
49. Nongonierma AB, FitzGerald RJ. Unlocking the biological potential of proteins from edible insects through enzymatic hydrolysis: a review. Innov Food Sci Emerg Technol. 2017;43:239–52. doi:10.1016/j.ifset.2017.08.014. [Google Scholar] [CrossRef]
50. Dai C, Ma H, Luo L, Yin X. Angiotensin I-converting enzyme (ACE) inhibitory peptide derived from Tenebrio molitor (L.) larva protein hydrolysate. Eur Food Res Technol. 2013;236(4):681–9. [Google Scholar]
51. Li X, Li Y, Huang X, Zheng J, Zhang F, Kan J. Identification and characterization of a novel angiotensin I-converting enzyme inhibitory peptide (ACEIP) from silkworm pupa. Food Sci Biotechnol. 2014;23(4):1017–23. [Google Scholar]
52. Jia J, Wu Q, Yan H, Gui Z. Purification and molecular docking study of a novel angiotensin-I converting enzyme (ACE) inhibitory peptide from alcalase hydrolysate of ultrasonic-pretreated silkworm pupa (Bombyx mori) protein. Process Biochem. 2015;50(5):876–83. doi:10.1016/j.procbio.2014.12.030. [Google Scholar] [CrossRef]
53. Wu Q, Jia J, Yan H, Du J, Gui Z. A novel angiotensin-І converting enzyme (ACE) inhibitory peptide from gastrointestinal protease hydrolysate of silkworm pupa (Bombyx mori) protein: biochemical characterization and molecular docking study. Peptides. 2015;68:17–24. doi:10.1016/j.peptides.2014.07.026. [Google Scholar] [PubMed] [CrossRef]
54. Longvah T, Mangthya K, Ramulu P. Nutrient composition and protein quality evaluation of eri silkworm (Samia ricinii) prepupae and pupae. Food Chem. 2011;128(2):400–3. doi:10.1016/j.foodchem.2011.03.041. [Google Scholar] [PubMed] [CrossRef]
55. Yi L, Lakemond CMM, Sagis LMC, Eisner-Schadler V, van Huis A, van Boekel MAJS. Extraction and characterisation of protein fractions from five insect species. Food Chem. 2013;141(4):3341–8. doi:10.1016/j.foodchem.2013.05.115.. [Google Scholar] [PubMed] [CrossRef]
56. Zhao X, Vázquez-Gutiérrez JL, Johansson DP, Landberg R, Langton M. Yellow mealworm protein for food purposes-extraction and functional properties. PLoS One. 2016 Feb 3;11(2):e0147791. doi:10.1371/journal.pone.0147791. [Google Scholar] [PubMed] [CrossRef]
57. Yang R, Zhao X, Kuang Z, Ye M, Luo G, Xiao G, et al. Optimization of antioxidant peptide production in the hydrolysis of silkworm (Bombyx mori L.) pupa protein using response surface methodology. J Food Agric Environ. 2013;11(1):952–6 [Google Scholar]
58. Azagoh C, Ducept F, Garcia R, Rakotozafy L, Cuvelier ME, Keller S, et al. Extraction and physicochemical characterization of Tenebrio molitor proteins. Food Res Int. 2016;88:24–31. doi:10.1016/j.foodres.2016.06.010. [Google Scholar] [PubMed] [CrossRef]
59. Bußler S, Rumpold BA, Jander E, Rawel HM, Schlüter OK. Recovery and techno-functionality of flours and proteins from two edible insect species: meal worm (Tenebrio molitor) and black soldier fly (Hermetia illucens) larvae. Heliyon. 2016;2(12):e00218. [Google Scholar]
60. Baigts-Allende D, Doost AS, Ramírez-Rodrigues M, Dewettinck K, van der Meeren P, de Meulenaer B, et al. Insect protein concentrates from Mexican edible insects: structural and functional characterization. LWT. 2021;152:112267. doi:10.1016/j.lwt.2021.112267. [Google Scholar] [CrossRef]
61. Mshayisa VV, van Wyk J, Zozo B. Nutritional, techno-functional and structural properties of black soldier fly (Hermetia illucens) larvae flours and protein concentrates. Foods. 2022;11(5):724. [Google Scholar] [PubMed]
62. Purschke B, Tanzmeister H, Meinlschmidt P, Baumgartner S, Lauter K, Jäger H. Recovery of soluble proteins from migratory locust (Locusta migratoria) and characterisation of their compositional and techno-functional properties. Food Res Int. 2018;106:271–9. doi:10.1016/j.foodres.2017.12.067. [Google Scholar] [PubMed] [CrossRef]
63. Vercruysse L, Smagghe G, Matsui T, van Camp J. Purification and identification of an angiotensin I converting enzyme (ACE) inhibitory peptide from the gastrointestinal hydrolysate of the cotton leafworm, Spodoptera littoralis. Process Biochem. 2008;43(8):900–4. doi:10.1016/j.procbio.2008.04.014. [Google Scholar] [CrossRef]
64. Vercruysse L, Smagghe G, Beckers T, Van CJ. Antioxidative and ACE inhibitory activities in enzymatic hydrolysates of the cotton leafworm, Spodoptera littoralis. Food Chem. 2009;114(1):38–43. doi:10.1016/j.foodchem.2008.09.011. [Google Scholar] [CrossRef]
65. Wang W, Wang N, Zhou Y, Zhang Y, Xu L, Xu J, et al. Isolation of a novel peptide from silkworm pupae protein components and interaction characteristics to angiotensin I-converting enzyme. Eur Food Res Technol. 2011;232(1):29–38. [Google Scholar]
66. Mintah BK, He R, Dabbour M, Xiang J, Jiang H, Agyekum AA, et al. Characterization of edible soldier fly protein and hydrolysate altered by multiple-frequency ultrasound: structural, physical, and functional attributes. Process Biochem. 2020;95:157–65. [Google Scholar]
67. Wu QY, Jia JQ, Tan GX, Xu JL, Gui ZZ. Physicochemical properties of silkworm larvae protein isolate and gastrointestinal hydrolysate bioactivities. Afr J Biotechnol. 2011;10(32):6145–53. [Google Scholar]
68. Zielińska E, Baraniak B, Karaś M, Rybczyńska K, Jakubczyk A. Selected species of edible insects as a source of nutrient composition. Food Res Int. 2015;77:460–6. doi:10.1016/j.foodres.2015.09.008. [Google Scholar] [CrossRef]
69. Zielińska E, Karaś M, Jakubczyk A. Antioxidant activity of predigested protein obtained from a range of farmed edible insects. Int J Food Sci Technol. 2017;52(2):306–12. [Google Scholar]
70. Leni G, Soetemans L, Jacobs J, Depraetere S, Gianotten N, Bastiaens L, et al. Protein hydrolysates from alphitobius diaperinus and Hermetia illucens larvae treated with commercial proteases. J Insects as Food Feed. 2020;6(4):393–404. [Google Scholar]
71. Luparelli AV, Jasmine HS, Lolli V, Lazzi C, Sforza S, Caligiani A. Dynamic changes in molecular composition of black soldier fly prepupae and derived biomasses with microbial fermentation. Food Chem X. 2022;14:100327. [Google Scholar] [PubMed]
72. Batish I, Brits D, Valencia P, Miyai C, Rafeeq S, Xu Y, et al. Effects of enzymatic hydrolysis on the functional properties, antioxidant activity and protein structure of black soldier fly (Hermetia illucens) protein. Insects. 2020;11(12):1–12. [Google Scholar]
73. Firmansyah M, Abduh MY. Production of protein hydrolysate containing antioxidant activity from Hermetia illucens. Heliyon. 2019;5(6):e02005. doi:10.1016/j.heliyon.2019.e02005. [Google Scholar] [PubMed] [CrossRef]
74. Caligiani A, Marseglia A, Leni G, Baldassarre S, Maistrello L, Dossena A, et al. Composition of black soldier fly prepupae and systematic approaches for extraction and fractionation of proteins, lipids and chitin. Food Res Int. 2018;105:812–20. doi:10.1016/j.foodres.2017.12.012. [Google Scholar] [PubMed] [CrossRef]
75. van Huis A, Oonincx DGAB. The environmental sustainability of insects as food and feed. A review. Agron Sustain Dev. 2017;37(5):43. [Google Scholar]
76. Nekrasov R, Zelenchenkova A, Chabaev M, Ivanov G, Antonov A, Pastukhova N. PSIII-37 dried black soldier fly larvae as a dietary supplement to the diet of growing pigs. J Anim Sci. 2018;96:314. doi:10.1093/jas/sky404.691. [Google Scholar] [PubMed] [CrossRef]
77. Fawole FJ, Adeoye AA, Tiamiyu LO, Ajala KI, Obadara SO, Ganiyu IO. Substituting fishmeal with Hermetia illucens in the diets of African catfish (Clarias gariepinuseffects on growth, nutrient utilization, haemato-physiological response, and oxidative stress biomarker. Aquaculture. 2020;518:734849. [Google Scholar]
78. Cappellozza S, Leonardi MG, Savoldelli S, Carminati D, Rizzolo A, Cortellino G, et al. A first attempt to produce proteins from insects by means of a circular economy. Animals. 2019;9(5):1–24. [Google Scholar]
79. Zotte AD, Singh Y, Michiels J, Cullere M. Black soldier fly (Hermetia illucens) as dietary source for laying quails: live performance, and egg physico-chemical quality, sensory profile and storage stability. Animals. 2019;9(3):1–20. [Google Scholar]
80. Li S, Ji H, Zhang B, Zhou J, Yu H. Defatted black soldier fly (Hermetia illucens) larvae meal in diets for juvenile Jian carp (Cyprinus carpio var. Jiangrowth performance, antioxidant enzyme activities, digestive enzyme activities, intestine and hepatopancreas histological structure. Aquaculture. 2017;477:62–70. [Google Scholar]
81. Wang G, Peng K, Hu J, Yi C, Chen X, Wu H, et al. Evaluation of defatted black soldier fly (Hermetia illucens L.) larvae meal as an alternative protein ingredient for juvenile Japanese seabass (Lateolabrax japonicus) diets. Aquaculture. 2019;507:144–54. doi:10.1016/j.aquaculture.2019.04.023. [Google Scholar] [CrossRef]
82. Cummins VC, Rawles SD, Thompson KR, Velasquez A, Kobayashi Y, Hager J, et al. Evaluation of black soldier fly (Hermetia illucens) larvae meal as partial or total replacement of marine fish meal in practical diets for Pacific white shrimp (Litopenaeus vannamei). Aquaculture. 2017;473:337–44. doi:10.1016/j.aquaculture.2017.02.022. [Google Scholar] [CrossRef]
83. Xu FM, Hou SW, Wang GX, Gong JY, Zhou L, Huang YH, et al. Effects of zymolytic black soldier fly (Hermetia illucens) pulp as dietary supplementation in largemouth bass (Micropterus salmoides). Aquac Reports. 2021;21:100823. doi:10.1016/j.aqrep.2021.100823. [Google Scholar] [CrossRef]
84. Roncarati A, Cappuccinelli R, Meligrana MCT, Anedda R, Uzzau S, Melotti P. Growing trial of gilthead sea bream (Sparus aurata) juveniles fed on chironomid meal as a partial substitution for fish meal. Animals. 2019;9(4):1–10. [Google Scholar]
85. Yamamoto FY, Suehs BA, Ellis M, Bowles PR, Older CE, Hume ME, et al. Dietary fishmeal replacement by black soldier fly larvae meals affected red drum (Sciaenops ocellatus) production performance and intestinal microbiota depending on what feed substrate the insect larvae were offered. Anim Feed Sci Technol. 2022;283:115179. doi:10.1016/j.anifeedsci.2021.115179. [Google Scholar] [CrossRef]
86. Mlaga KG, Agboka K, Attivi K, Tona K, Osseyi E. Assessment of the chemical characteristics and nutritional quality of meat from broiler chicken fed black soldier fly (Hermetia illucens) larvae meal. Heliyon. 2022;8(11):e11718. [Google Scholar] [PubMed]
87. Jankowski J, Kozłowski K, Zduńczyk Z, Stępniowska A, Ognik K, Kierończyk B, et al. The effect of dietary full-fat Hermetia illucens larvae meal on gut physiology and growth performance in young turkeys. Anim Feed Sci Technol. 2021;275:114879. [Google Scholar]
88. Volek Z, Zita L, Adámková A, Adámek M, Mlček J, Plachý V. Dietary inclusion of crickets (Acheta domesticus) and yellow mealworm meal (Tenebrio molitor) in comparison with soybean meal: effect on the growth, total tract apparent digestibility, and nitrogen balance of fattening rabbits. Animals. 2023;13(10):1637. [Google Scholar] [PubMed]
89. Elahi U, Wang J, Ma YB, Wu SG, Wu J, Qi GH, et al. Evaluation of yellow mealworm meal as a protein feedstuff in the diet of broiler chicks. Animals. 2020;10(2):1–15. [Google Scholar]
90. Bruni L, Pastorelli R, Viti C, Gasco L, Parisi G. Characterisation of the intestinal microbial communities of rainbow trout (Oncorhynchus mykiss) fed with Hermetia illucens (black soldier fly) partially defatted larva meal as partial dietary protein source. Aquaculture. 2018;487:56–63. doi:10.1016/j.aquaculture.2018.01.006. [Google Scholar] [CrossRef]
91. Dietz C, Liebert F. Does graded substitution of soy protein concentrate by an insect meal respond on growth and N-utilization in Nile tilapia (Oreochromis niloticus)? Aquac Reports. 2018;12:43–8. doi:10.1016/j.aqrep.2018.09.001. [Google Scholar] [CrossRef]
92. Gu J, Liang H, Ge X, Xia D, Pan L, Mi H, et al. A study of the potential effect of yellow mealworm (Tenebrio molitor) substitution for fish meal on growth, immune and antioxidant capacity in juvenile largemouth bass (Micropterus salmoides). Fish Shellfish Immunol. 2022;120:214–21. doi:10.1016/j.fsi.2021.11.024. [Google Scholar] [PubMed] [CrossRef]
93. van Huis A. Edible insects contributing to food security? Agric Food Secur. 2015;4(1):1–9. [Google Scholar]
94. Wu RA, Ding Q, Yin L, Chi X, Sun N, He R, et al. Comparison of the nutritional value of mysore thorn borer (Anoplophora chinensis) and mealworm larva (Tenebrio molitoramino acid, fatty acid, and element profiles. Food Chem. 2020;323:126818. doi:10.1016/j.foodchem.2020.126818. [Google Scholar] [PubMed] [CrossRef]
95. Ghosh S, Lee SM, Jung C, Meyer-Rochow VB. Nutritional composition of five commercial edible insects in South Korea. J Asia Pac Entomol. 2017;20(2):686–94. doi:10.1016/j.aspen.2017.04.003. [Google Scholar] [CrossRef]
96. Fontes TV, Oliveira KRB, Almeida ILG, Orlando TM, Borges RP, Costa DV, et al. Digestibility of insect meals for nile tilapia fingerlings. Animals. 2019;9(181):1–8. doi:10.3390/ani9040181. [Google Scholar] [PubMed] [CrossRef]
97. Mastoraki M, Katsika L, Enes P, Guerreiro I, Kotzamanis YP, Gasco L, et al. Insect meals in feeds for juvenile gilthead seabream (Sparus aurataeffects on growth, blood chemistry, hepatic metabolic enzymes, body composition and nutrient utilization. Aquaculture. 2022;561:738674. [Google Scholar]
98. Tran HQ, Nguyen TT, Prokešová MD, Matoušek J, Tomčala A, Van Doan H, et al. Insight into bioavailability of various insect meals for European perch (Perca fluviatilisa nutritional and stable isotopic evaluation. Aquaculture. 2023;563:738912. [Google Scholar]
99. Pietras M, Orczewska-Dudek S, Szczurek W, Pieszka M. Effect of dietary lupine seeds (Lupinus luteus L.) and different insect larvae meals as protein sources in broiler chicken diet on growth performance, carcass, and meat quality. Livest Sci. 2021;250:104537. doi:10.1016/j.livsci.2021.104537. [Google Scholar] [CrossRef]
100. Neumann C, Velten S, Liebert F. N balance studies emphasize the superior protein quality of pig diets at high inclusion level of algae meal (Spirulina platensis) or insect meal (Hermetia illucens) when adequate amino acid supplementation is ensured. Animals. 2018;8(10):1–14. [Google Scholar]
101. Malla N, Nørgaard JV, Lærke HN, Heckmann LHL, Roos N. Some insect species are good-quality protein sources for children and adults: digestible indispensable amino acid score (DIAAS) determined in growing pigs. J Nutr. 2022;152(4):1042–51. doi:10.1093/jn/nxac019. [Google Scholar] [PubMed] [CrossRef]
102. Lei XJ, Kim TH, Park JH, Kim IH. Evaluation of supplementation of defatted black soldier fly (Hermetia illucens) larvae meal in beagle dogs. Ann Anim Sci. 2019;19(3):767–77. [Google Scholar]
103. Kröger S, Heide C, Zentek J. Evaluation of an extruded diet for adult dogs containing larvae meal from the Black soldier fly (Hermetia illucens). Anim Feed Sci Technol. 2020;270:114699. [Google Scholar]
104. Penazzi L, Schiavone A, Russo N, Nery J, Valle E, Madrid J, et al. In vivo and in vitro digestibility of an extruded complete dog food containing black soldier fly (Hermetia illucens) larvae meal as protein source. Front Vet Sci. 2021;8:653411. [Google Scholar] [PubMed]
105. Pan J, Xu H, Cheng Y, Mintah BK, Dabbour M, Yang F, et al. Recent insight on edible insect protein: extraction, functional properties, allergenicity, bioactivity, and applications. Foods. 2022;11(19):1–21. [Google Scholar]
106. Montevecchi G, Licciardello F, Masino F, Miron LT, Antonelli A. Fortification of wheat flour with black soldier fly prepupae. Evaluation of technological and nutritional parameters of the intermediate doughs and final baked products. Innov Food Sci Emerg Technol. 2021;69:102666. doi:10.1016/j.ifset.2021.102666. [Google Scholar] [CrossRef]
107. Kowalski S, Mikulec A, Mickowska B, Skotnicka M, Mazurek A. Wheat bread supplementation with various edible insect flours. Influ Chem Compos Nutr Technol Aspects. LWT. 2022;159:113220. [Google Scholar]
108. Nissen L, Samaei SP, Babini E, Gianotti A. Gluten free sourdough bread enriched with cricket flour for protein fortification: antioxidant improvement and Volatilome characterization. Food Chem. 2020;333:127410. doi:10.1016/j.foodchem.2020.127410. [Google Scholar] [PubMed] [CrossRef]
109. Biró B, Fodor R, Szedljak I, Pásztor-Huszár K, Gere A. Buckwheat-pasta enriched with silkworm powder: technological analysis and sensory evaluation. LWT. 2019;116:108542. doi:10.1016/j.lwt.2019.108542. [Google Scholar] [CrossRef]
110. Scholliers J, Steen L, Fraeye I. Partial replacement of meat by superworm (Zophobas morio larvae) in cooked sausages: effect of heating temperature and insect: meat ratio on structure and physical stability. Innov Food Sci Emerg Technol. 2020;66:102535. doi:10.1016/j.ifset.2020.102535. [Google Scholar] [CrossRef]
111. Zhang F, Cao C, Kong B, Sun F, Shen X, Yao X, et al. Pre-dried mealworm larvae flour could partially replace lean meat in frankfurters: effect of pre-drying methods and replacement ratios. Meat Sci. 2022;188:108802. doi:10.1016/j.meatsci.2022.108802. [Google Scholar] [PubMed] [CrossRef]
112. Azzollini D, Wibisaphira T, Lakemond CMM, Fogliano V. Toward the design of insect-based meat analogue: the role of calcium and temperature in coagulation behavior of Alphitobius diaperinus proteins. LWT. 2019;100:75–82. doi:10.1016/j.lwt.2018.10.037. [Google Scholar] [CrossRef]
113. Kiiru SM, Kinyuru JN, Kiage BN, Martin A, Marel AK, Osen R. Extrusion texturization of cricket flour and soy protein isolate: influence of insect content, extrusion temperature, and moisture-level variation on textural properties. Food Sci Nutr. 2020;8(8):4112–20. [Google Scholar] [PubMed]
114. Stoops J, Vandeweyer D, Crauwels S, Verreth C, Boeckx H, van der Borght M, et al. Minced meat-like products from mealworm larvae (Tenebrio molitor and Alphitobius diaperinusmicrobial dynamics during production and storage. Innov Food Sci Emerg Technol. 2017;41:1–9. doi: 10.1016/j.ifset.2017.02.001. [Google Scholar] [CrossRef]
115. Kim HW, Setyabrata D, Lee YJ, Jones OG, Kim YHB. Pre-treated mealworm larvae and silkworm pupae as a novel protein ingredient in emulsion sausages. Innov Food Sci Emerg Technol. 2016;38:116–23. doi:10.1016/j.ifset.2016.09.023. [Google Scholar] [CrossRef]
116. Scholliers J, Steen L, Fraeye I. Structure and physical stability of hybrid model systems containing pork meat and superworm (Zophobas morio larvaethe influence of heating regime and insect: meat ratio. Innov Food Sci Emerg Technol. 2020;65:102452. doi:10.1016/j.ifset.2020.102452. [Google Scholar] [CrossRef]
117. Rossi S, Parrotta L, Del Duca S, Rosa MD, Patrignani F, Schluter O, et al. Effect of Yarrowia lipolytica RO25 cricket-based hydrolysates on sourdough quality parameters. LWT. 2021;148:111760. doi:10.1016/j.lwt.2021.111760. [Google Scholar] [CrossRef]
118. Osimani A, Milanović V, Cardinali F, Roncolini A, Garofalo C, Clementi F, et al. Bread enriched with cricket powder (Acheta domesticusa technological, microbiological and nutritional evaluation. Innov Food Sci Emerg Technol. 2018;48:150–63. [Google Scholar]
119. Roncolini A, Milanović V, Aquilanti L, Cardinali F, Garofalo C, Sabbatini R, et al. Lesser mealworm (Alphitobius diaperinus) powder as a novel baking ingredient for manufacturing high-protein, mineral-dense snacks. Food Res Int. 2020;131:109031. [Google Scholar] [PubMed]
120. da Rosa Machado C, Thys RCS. Cricket powder (Gryllus assimilis) as a new alternative protein source for gluten-free breads. Innov Food Sci Emerg Technol. 2019;56:102180. doi:10.1016/j.ifset.2019.102180. [Google Scholar] [CrossRef]
121. González CM, Garzón R, Rosell CM. Insects as ingredients for bakery goods. A comparison study of H. illucens, A. domestica and T. molitor flours. Innov Food Sci Emerg Technol. 2019;51:205–10. doi:10.1016/j.ifset.2018.03.021. [Google Scholar] [CrossRef]
122. de Oliveira LM, da Silva Lucas AJ, Cadaval CL, Mellado MS. Bread enriched with flour from cinereous cockroach (Nauphoeta cinerea). Innov Food Sci Emerg Technol. 2017;44:30–5. doi:10.1016/j.ifset.2017.08.015. [Google Scholar] [CrossRef]
123. Haber M, Mishyna M, Martinez JJI, Benjamin O. The influence of grasshopper (Schistocerca gregaria) powder enrichment on bread nutritional and sensorial properties. LWT. 2019;115:108395. doi:10.1016/j.lwt.2019.108395. [Google Scholar] [CrossRef]
124. Severini C, Azzollini D, Albenzio M, Derossi A. On printability, quality and nutritional properties of 3D printed cereal based snacks enriched with edible insects. Food Res Int. 2018;106:666–76. doi:10.1016/j.foodres.2018.01.034. [Google Scholar] [PubMed] [CrossRef]
125. Gaglio R, Barbera M, Tesoriere L, Osimani A, Busetta G, Matraxia M, et al. Sourdough ciabatta bread enriched with powdered insects: physicochemical, microbiological, and simulated intestinal digesta functional properties. Innov Food Sci Emerg Technol. 2021;72:102755. doi:10.1016/j.ifset.2021.102755. [Google Scholar] [CrossRef]
126. Akande AO, Jolayemi OS, Adelugba VA, Akande ST. Silkworm pupae (Bombyx mori) and locusts as alternative protein sources for high-energy biscuits. J Asia Pac Entomol. 2020;23(1):234–41. doi:10.1016/j.aspen.2020.01.003. [Google Scholar] [CrossRef]
127. Tello A, Aganovic K, Parniakov O, Carter A, Heinz V, Smetana S. Product development and environmental impact of an insect-based milk alternative. Futur Foods. 2021;4:100080. doi:10.1016/j.fufo.2021.100080. [Google Scholar] [CrossRef]
128. Traynor A, Burns DT, Wu D, Karoonuthaisiri N, Petchkongkaew A, Elliott CT. An analysis of emerging food safety and fraud risks of novel insect proteins within complex supply chains. npj Sci Food. 2024;8(1):7. [Google Scholar] [PubMed]
129. Ribeiro JC, Sousa-Pinto B, Fonseca J, Fonseca SC, Cunha LM. Edible insects and food safety: allergy. J Insects Food Feed. 2021;7(5):833–47. [Google Scholar]
130. Beaumont P, Courtois J, van der Brempt X, Tollenaere S. Food-induced anaphylaxis to Tenebrio molitor and allergens implicated. Rev Fr Allergol. 2019;59(5):389–93. doi:10.1016/j.reval.2019.06.001. [Google Scholar] [CrossRef]
131. Barre A, Pichereaux C, Velazquez E, Maudouit A, Simplicien M, Garnier L, et al. Insights into the allergenic potential of the edible yellow mealworm (Tenebrio molitor). Foods. 2019;8(10):1–17. [Google Scholar]
132. Romy G, Courtois J, Laouali S, van der Brempt X, Jacquier J, Cavalier E, et al. Sensitization profile of cricket food-allergic or cricket tolerant patients in an entomophagous population in Niamey, Niger. Clin Chim Acta. 2019;493:S98. doi:10.1016/j.cca.2019.03.209. [Google Scholar] [CrossRef]
133. Zhang Z, Fang C, Liu D, Zhou X, Wang D, Zhang W. Preparation and characterization of the protein edible film extracted from the migratory locust (Locusta migratoria). Food Packag Shelf Life. 2022;33:100899. doi:10.1016/j.fpsl.2022.100899. [Google Scholar] [CrossRef]
134. Zhang Z, Zhou X, Fang C, Wang D. Characterization of the antimicrobial edible film based on grasshopper protein/soy protein isolate/cinnamaldehyde blend crosslinked with xylose. Front Nutr. 2022;9:1–12. [Google Scholar]
135. Qoirinisa S, Arnamalia A, Permata ME, Ramdani RN. The Study on utilization of grasshoppers gelatine as edible film in optimizing environmentally friendly packaging. J Food Pharm Sci. 2022;10(1):620–5. [Google Scholar]
136. Chandran M, Vignesh S, Manickam Professor L, Anandakumar S, Manickam L. Utilization of black soldier fly (Hermetia illucens) prepupae flour in development of bio-packaging film. Pharma Innov J. 2021;11:335–40. [Google Scholar]
137. Klunder HC, Wolkers-Rooijackers J, Korpela JM, Nout MJR. Microbiological aspects of processing and storage of edible insects. Food Control. 2012;26(2):628–31. doi:10.1016/j.foodcont.2012.02.013. [Google Scholar] [CrossRef]
138. Charlton A. Safety and quality considerations of insects for feed and food. Insects Feed World. 2014;17:7–16. [Google Scholar]
139. Charlton AJ, Dickinson M, Wakefield ME, Fitches E, Kenis M, Han R, et al. Exploring the chemical safety of fly larvae as a source of protein for animal feed. J Insects Food Feed. 2015;1(1):7–16. [Google Scholar]
140. Henry M, Gasco L, Piccolo G, Fountoulaki E. Review on the use of insects in the diet of farmed fish: past and future. Anim Feed Sci Technol. 2015;203:1–22. doi:10.1016/j.anifeedsci.2015.03.001. [Google Scholar] [CrossRef]
141. Purschke B, Scheibelberger R, Axmann S, Adler A, Purschke B, Scheibelberger R, et al. Food additives & contaminants : part A impact of substrate contamination with mycotoxins, heavy metals and pesticides on the growth performance and composition of black soldier fly larvae (Hermetia illucens) for use in the feed and food value chain fly. Food Addit Contam Part A. 2017;34(8):1410–20. [Google Scholar]
142. Li W, Bischel HN. Are resource recovery insects safe for feed and food? A screening approach for bioaccumulative trace organic contaminants. Sci Total Environ. 2022;837:155850. [Google Scholar] [PubMed]
143. Malematja E, Manyelo TG, Sebola NA, Kolobe SD, Mabelebele M. The accumulation of heavy metals in feeder insects and their impact on animal production. Sci Total Environ. 2023;885:163716. doi:10.1016/j.scitotenv.2023.163716. [Google Scholar] [PubMed] [CrossRef]
144. de Clercq P, Mason PG, Babendreier D. Benefits and risks of exotic biological control agents. BioControl. 2011;56(4):681–98. [Google Scholar]
145. Weinreis Y, Baum CM, Smetana S. Insect production as a novel alternative to livestock farming: exploring interest and willingness to adopt among German farmers. Sustain Prod Consum. 2023;35:28–39. doi:10.1016/j.spc.2022.10.004. [Google Scholar] [CrossRef]
146. White KP, Al-Shawaf L, Lewis DMG, Wehbe YS. Food neophobia and disgust, but not hunger, predict willingness to eat insect protein. Pers Individ Dif. 2023;202:111944. doi:10.1016/j.paid.2022.111944. [Google Scholar] [CrossRef]
147. Puteri B, Jahnke B, Zander K. Booming the bugs: how can marketing help increase consumer acceptance of insect-based food in Western countries? Appetite. 2023;187:106594. doi:10.1016/j.appet.2023.106594. [Google Scholar] [PubMed] [CrossRef]
148. Veldkamp T, Meijer N, Alleweldt F, Deruytter D, van Campenhout L, Gasco L, et al. Overcoming technical and market barriers to enable sustainable large-scale production and consumption of insect proteins in Europe: a SUSINCHAIN perspective. Insects. 2022;13(3):281. [Google Scholar] [PubMed]
149. Chaklader MR, Howieson J, Foysal MJ, Fotedar R. Transformation of fish waste protein to Hermetia illucens protein improves the efficacy of poultry by-products in the culture of juvenile barramundi, Lates calcarifer. Sci Total Environ. 2021;796:149045. doi:10.1016/j.scitotenv.2021.149045. [Google Scholar] [PubMed] [CrossRef]
150. Stejskal V, Tran HQ, Prokesova M, Gebauer T, Giang PT, Gai F, et al. Partially defatted Hermetia illucens larva meal in diet of eurasian perch (Perca fluviatilis) juveniles. Animals. 2020;10(10):1–17. [Google Scholar]
151. Magalhães R, Sánchez-López A, Leal RS, Martínez-Llorens S, Oliva-Teles A, Peres H. Black soldier fly (Hermetia illucens) pre-pupae meal as a fish meal replacement in diets for European seabass (Dicentrarchus labrax). Aquaculture. 2017;476:79–85. [Google Scholar]
152. Gasco L, Henry M, Piccolo G, Marono S, Gai F, Renna M, et al. Tenebrio molitor meal in diets for European sea bass (Dicentrarchus labrax L.) juveniles: growth performance, whole body composition and in vivo apparent digestibility. Anim Feed Sci Technol. 2016;220:34–45. doi:10.1016/j.anifeedsci.2016.07.003. [Google Scholar] [CrossRef]
153. Yildirim-Aksoy M, Eljack R, Schrimsher C, Beck BH. Use of dietary frass from black soldier fly larvae, Hermetia illucens, in hybrid tilapia (Nile x Mozambique, Oreocromis niloticus x O. mozambique) diets improves growth and resistance to bacterial diseases. Aquac Reports. 2020;17:100373. doi:10.1016/j.aqrep.2020.100373. [Google Scholar] [CrossRef]
154. Fischer H, Romano N, Renukdas N, Kumar V, Sinha AK. Comparing black soldier fly (Hermetia illucens) larvae versus prepupae in the diets of largemouth bass, Micropterus salmoides: effects on their growth, biochemical composition, histopathology, and gene expression. Aquaculture. 2021;546:737323. doi:10.1016/j.aquaculture.2021.737323. [Google Scholar] [CrossRef]
155. Estévez A, Blanco B, Fernández L, Ferreira M, Soula M. Effects of alternative and sustainable ingredients, insect meal, microalgae and protein and lipid from tuna cooking water, on meagre (Argyrosomus regius) growth, food conversion and muscle and liver composition. Aquaculture. 2022;548:737549. [Google Scholar]
156. Panini RL, Freitas LEL, Guimarães AM, Rios C, da Silva MFO, Vieira FN, et al. Potential use of mealworms as an alternative protein source for Pacific white shrimp: digestibility and performance. Aquaculture. 2017;473:115–20. [Google Scholar]
157. Motte C, Rios A, Lefebvre T, Do H, Henry M, Jintasataporn O. Replacing fish meal with defatted insect meal (Yellow mealworm Tenebrio molitor) improves the growth and immunity of pacific white shrimp (Litopenaeus vannamei). Animals. 2019;9(5):258. [Google Scholar] [PubMed]
158. Huang B, Zhang S, Dong X, Chi S, Yang Q, Liu H, et al. Effects of fishmeal replacement by black soldier fly on growth performance, digestive enzyme activity, intestine morphology, intestinal flora and immune response of pearl gentian grouper (Epinephelus fuscoguttatus ♀ × Epinephelus lanceolatus ♂). Fish Shellfish Immunol. 2022;120:497–506. [Google Scholar] [PubMed]
159. Stejskal V, Tran HQ, Prokesová M, Zare M, Gebauer T, Policar T, et al. Defatted black soldier fly (Hermetia illucens) in pikeperch (Sander lucioperca) diets: effects on growth performance, nutrient digestibility, fillet quality, economic and environmental sustainability. Anim Nutr. 2023;12:7–19. doi:10.1016/j.aninu.2022.06.022. [Google Scholar] [PubMed] [CrossRef]
160. Stamer A, Wesselss S, Neidigk R, Hoerstgen-Schwark G. Black soldier fly (Hermetia illucens) larvae-meal as an example for a new feed ingredients ‘class in aquaculture diets. In: 18th IFOAM OWC Scientific Track: 4th ISOFAR Scientific Conference; 2014; Istanbul, Turkey. p. 13–5. [Google Scholar]
161. Cardinaletti G, Randazzo B, Messina M, Zarantoniello M, Giorgini E, Zimbelli A, et al. Effects of graded dietary inclusion level of full-fat hermetia illucens prepupae meal in practical diets for rainbow trout (Oncorhynchus mykiss). Animals. 2019;9(251):1–21. [Google Scholar]
162. Rimoldi S, Gini E, Iannini F, Gasco L, Terova G. The effects of dietary insect meal from Hermetia illucens prepupae on autochthonous gut microbiota of rainbow trout (Oncorhynchus mykiss). Animals. 2019;9(4):1–17. [Google Scholar]
163. Randazzo B, Zarantoniello M, Gioacchini G, Cardinaletti G, Belloni A, Giorgini E, et al. Physiological response of rainbow trout (Oncorhynchus mykiss) to graded levels of Hermetia illucens or poultry by-product meals as single or combined substitute ingredients to dietary plant proteins. Aquaculture. 2021;538:736550. [Google Scholar]
164. Rema P, Saravanan S, Armenjon B, Motte C, Dias J. Graded incorporation of defatted yellow mealworm (Tenebrio molitor) in rainbow trout (Oncorhynchus mykiss) diet improves growth performance and nutrient retention. Animals. 2019;9(4):187. [Google Scholar] [PubMed]
165. Chemello G, Renna M, Caimi C, Guerreiro I, Oliva-Teles A, Enes P, et al. Partially defatted tenebrio molitor larva meal in diets for grow-out rainbow trout, Oncorhynchus mykiss (Walbaumeffects on growth performance, diet digestibility and metabolic responses. Animals. 2020;10(2):229. [Google Scholar] [PubMed]
166. Muin H, Taufek NM. Evaluation of growth performance, feed efficiency and nutrient digestibility of red hybrid tilapia fed dietary inclusion of black soldier fly larvae (Hermetia illucens). Aquac Fish. 2024;46–51. [Google Scholar]
167. Hu Y, Huang Y, Tang T, Zhong L, Chu W, Dai Z, et al. Effect of partial black soldier fly (Hermetia illucens L.) larvae meal replacement of fish meal in practical diets on the growth, digestive enzyme and related gene expression for rice field eel (Monopterus albus). Aquac Reports. 2020;17:100345. doi:10.1016/j.aqrep.2020.100345. [Google Scholar] [CrossRef]
168. Hoffmann L, Rawski M, Pruszyńska-Oszmałek E, Kołodziejski P, Mazurkiewicz J. Environmentally sustainable feeding system for sea trout (Salmo trutta m. truttalive food and insect meal-based diets in larval rearing. Aquac Reports. 2021;21:100795. [Google Scholar]
169. Rawski M, Mazurkiewicz J, Kiero’nczyk B, Józefiak D. Black soldier fly full-fat larvae meal is more profitable than effects on aquaculture sustainability, economy and fish. Animals. 2021;11:1–13. [Google Scholar]
170. Caimi C, Gasco L, Biasato I, Malfatto V, Varello K, Prearo M, et al. Could dietary black soldier fly meal inclusion affect the liver and intestinal histological traits and the oxidative stress biomarkers of siberian sturgeon (Acipenser baerii) juveniles? Animals. 2020;10(1):155. [Google Scholar] [PubMed]
171. Caimi C, Renna M, Lussiana C, Bonaldo A, Gariglio M, Meneguz M, et al. First insights on black soldier fly (Hermetia illucens L.) larvae meal dietary administration in Siberian sturgeon (Acipenser baerii Brandt) juveniles. Aquaculture. 2020;515:734539. doi:10.1016/j.aquaculture.2019.734539. [Google Scholar] [CrossRef]
172. Villanueva-Gutiérrez E, Rodriguez-Armenta C, González-Félix ML, Perez-Velazquez M. Incorporating hydrolyzed soy protein or black soldier fly (Hermetia illucens) larvae meal into feeds for Totoaba macdonaldi. Aquaculture. 2022;554:738152. [Google Scholar]
173. Kroeckel S, Harjes AGE, Roth I, Katz H, Wuertz S, Susenbeth A, et al. When a turbot catches a fly: evaluation of a pre-pupae meal of the Black Soldier Fly (Hermetia illucens) as fish meal substitute-growth performance and chitin degradation in juvenile turbot (Psetta maxima). Aquaculture. 2012;364–365:345–52. doi:10.1016/j.aquaculture.2012.08.041. [Google Scholar] [CrossRef]
174. Xiao X, Jin P, Zheng L, Cai M, Yu Z, Yu J, et al. Effects of black soldier fly (Hermetia illucens) larvae meal protein as a fishmeal replacement on the growth and immune index of yellow catfish (Pelteobagrus fulvidraco). Aquac Res. 2018;49(4):1569–77. [Google Scholar]
175. Su J, Gong Y, Cao S, Lu F, Han D, Liu H, et al. Effects of dietary Tenebrio molitor meal on the growth performance, immune response and disease resistance of yellow catfish (Pelteobagrus fulvidraco). Fish Shellfish Immunol. 2017;69:59–66. doi: 10.1016/j.fsi.2017.08.008. [Google Scholar] [PubMed] [CrossRef]
176. Zarantoniello M, Randazzo B, Truzzi C, Giorgini E, Marcellucci C, Vargas-Abúndez JA, et al. A six-months study on black soldier Fly (Hermetia illucens) based diets in zebrafish. Sci Rep. 2019;9(1):1–12. [Google Scholar]
177. Loponte R, Nizza S, Bovera F, De Riu N, Fliegerova K, Lombardi P, et al. Growth performance, blood profiles and carcass traits of Barbary partridge (Alectoris barbara) fed two different insect larvae meals (Tenebrio molitor and Hermetia illucens). Res Vet Sci. 2017;115:183–8. doi:10.1016/j.rvsc.2017.04.017. [Google Scholar] [PubMed] [CrossRef]
178. Brede A, Wecke C, Liebert F. Does the optimal dietary methionine to cysteine ratio in diets for growing chickens respond to high inclusion rates of insect meal from Hermetia illucens? Animals. 2018;8(11):1–16. [Google Scholar]
179. Biasato I, Ferrocino I, Grego E, Dabbou S, Gai F, Gasco L, et al. Gut microbiota and mucin composition in female broiler chickens fed diets including yellow mealworm (Tenebrio molitor, L.). Animals. 2019;9(2):1–15. [Google Scholar]
180. de Marco M, Martínez S, Hernandez F, Madrid J, Gai F, Rotolo L, et al. Nutritional value of two insect larval meals (Tenebrio molitor and Hermetia illucens) for broiler chickens: apparent nutrient digestibility, apparent ileal amino acid digestibility and apparent metabolizable energy. Anim Feed Sci Technol. 2015;209:211–8. doi:10.1016/j.anifeedsci.2015.08.006. [Google Scholar] [CrossRef]
181. Józefiak A, Benzertiha A, Kierończyk B, Łukomska A, Wesołowska I, Rawski M. Improvement of cecal commensal microbiome following the insect additive into chicken diet. Animals. 2020;10(4):577. [Google Scholar]
182. Benzertiha A, Rawski M, Józefiak AKK, Jankowski J, Józefiak D. Tenebrio molitor and Zophobas morio full-fat meals in broiler chicken diets: effects on nutrients digestibility, digestive enzyme activities, and cecal microbiome. Animals. 2019;5(3):248–53. [Google Scholar]
183. Cullere M, Woods MJ, Van Emmenes L, Pieterse E, Hoffman LC, Zotte AD. Hermetia illucens larvae reared on different substrates in broiler quail diets: effect on physicochemical and sensory quality of the quail meat. Animals. 2019;9(8):525. [Google Scholar] [PubMed]
184. de Carvalho TSG, do Saad CEP, Esposito M, Faria PB, Alvarenga RR, Ferreira LG, et al. Reproductive characteristics of cockatiels (Nymphicus hollandicus) maintained in captivity and receiving Madagascar cockroach (Gromphadorhina portentosa) meal. Animals. 2019;9(6):312. [Google Scholar]
185. Sayed WAA, Ibrahim NS, Hatab MH, Zhu F, Rumpold BA. Comparative study of the use of insect meal from spodoptera littoralis and bactrocera zonata for feeding japanese quail chicks. Animals. 2019;9(4)136. [Google Scholar] [PubMed]
186. Attivi K, Mlaga KG, Agboka K, Tona K, Kouame YAE, Lin H. Effect of fish meal replacement by black Soldier Fly (Hermetia illucens) larvae meal on serum biochemical indices, thyroid hormone and zootechnical performance of laying chickens. J Appl Poult Res. 2022;31(3):100275. [Google Scholar]
187. Moniello G, Ariano A, Panettieri V, Tulli F, Olivotto I, Messina M, et al. Intestinal morphometry, enzymatic and microbial activity in laying hens fed different levels of a Hermetia illucens larvae meal and toxic elements content of the insect meal and diets. Animals. 2019;9(3):1–13. [Google Scholar]
188. Bovera F, Loponte R, Pero ME, Cutrignelli MI, Calabrò S, Musco N, et al. Laying performance, blood profiles, nutrient digestibility and inner organs traits of hens fed an insect meal from Hermetia illucens larvae. Res Vet Sci. 2018;120:86–93. doi:10.1016/j.rvsc.2018.09.006. [Google Scholar] [PubMed] [CrossRef]
189. Kawasaki K, Hashimoto Y, Hori A, Kawasaki T, Hirayasu H, Iwase SI, et al. Evaluation of black soldier fly (Hermetia illucens) larvae and pre-pupae raised on household organic waste, as potential ingredients for poultry feed. Animals. 2019;9(3):98. [Google Scholar] [PubMed]
190. Kovitvadhi A, Chundang P, Thongprajukaew K, Tirawattanawanich C, Srikachar S, Chotimanothum B. Potential of insect meals as protein sources for meat-type ducks based on in vitro digestibility. Animals. 2019;9(4):1–10. [Google Scholar]
191. Gariglio M, Dabbou S, Crispo M, Biasato I, Gai F, Gasco L, et al. Effects of the dietary inclusion of partially defatted black soldier fly (Hermetia illucens) meal on the blood chemistry and tissue (Spleen, Liver, Thymus, and Bursa of Fabricius) Histology of Muscovy Ducks (Cairina moschata domestica). Animals. 2019;9(6):307. [Google Scholar] [PubMed]
192. Kozłowski K, Ognik K, Stępniowska A, Juśkiewicz J, Zduńczyk Z, Kierończyk B, et al. Growth performance, immune status and intestinal fermentative processes of young turkeys fed diet with additive of full fat meals from Tenebrio molitor and Hermetia illucens. Anim Feed Sci Technol. 2021;278:114994. [Google Scholar]
193. Li M, Li M, Wang G, Liu C, Shang R, Chen Y, et al. Defatted black soldier fly (Hermetia illucens) larvae meal can partially replace fish meal in diets for adult Chinese soft-shelled turtles. Aquaculture. 2021;541:736758. [Google Scholar]
194. Bosch G, Vervoort JJM, Hendriks WH. In vitro digestibility and fermentability of selected insects for dog foods. Anim Feed Sci Technol. 2016;221:174–84. doi:10.1016/j.anifeedsci.2016.08.018. [Google Scholar] [CrossRef]
195. Yu M, Li Z, Chen W, Rong T, Wang G, Li J, et al. Use of Hermetia illucens larvae as a dietary protein source: effects on growth performance, carcass traits, and meat quality in finishing pigs. Meat Sci. 2019;158:107837. [Google Scholar] [PubMed]
196. Yu M, Li Z, Chen W, Rong T, Wang G, Ma X. Hermetia illucens larvae as a potential dietary protein source altered the microbiota and modulated mucosal immune status in the colon of finishing pigs. J Anim Sci Biotechnol. 2019;10(1):1–16. [Google Scholar]
197. Chia SY, Tanga CM, Osuga IM, Alaru AO, Mwangi DM, Githinji M, et al. Effect of dietary replacement of fishmeal by insect meal on growth performance, blood profiles and economics of growing pigs in Kenya. Animals. 2019;9(10):705. [Google Scholar] [PubMed]
Appendix
Cite This Article
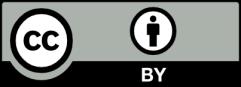
This work is licensed under a Creative Commons Attribution 4.0 International License , which permits unrestricted use, distribution, and reproduction in any medium, provided the original work is properly cited.