Open Access
REVIEW
Renewable Polymers in Biomedical Applications: From the Bench to the Market
1 Department of Health and Biological Sciences, University of Araraquara—UNIARA, Araraquara, 14801-340, Brazil
2 Laboratory of Materials (LabMat) and Laboratory of Biopolymers (LaBioPol), Federal Institute of Education, Science and Technology of São Paulo Campus Itapetininga (IFSP), Itapetininga, 18.202‑000, Brazil
3 Instituto Tecnológico de Buenos Aires, Cidade Autônoma de Buenos Aires, C1437FBC, Argentina
4 Conselho Nacional de Pesquisas Científicas e Técnicas (CONICET), Cidade Autônoma de Buenos Aires, C1425FQB, Argentina
5 Tecnologia YPF (Y-TEC), Berisso, Buenos Aires, 1923, Argentina
* Corresponding Author: Eliane Trovatti. Email:
(This article belongs to the Special Issue: Special Issue in Celebration of JRM 10 Years)
Journal of Renewable Materials 2024, 12(4), 643-666. https://doi.org/10.32604/jrm.2024.048957
Received 22 December 2023; Accepted 30 January 2024; Issue published 12 June 2024
Abstract
Polymers from renewable resources have been used for a long time in biomedical applications and found an irreplaceable role in some of them. Their uses have been increasing because of their attractive properties, contributing to the improvement of life quality, mainly in drug release systems and in regenerative medicine. Formulations using natural polymer, nano and microscale particles preparation, composites, blends and chemical modification strategies have been used to improve their properties for clinical application. Although many studies have been carried out with these natural polymers, the way to reach the market is long and only very few of them become commercially available. Vegetable cellulose, bacterial cellulose, chitosan, poly(lactic acid) and starch can be found among the most studied polymers for biological applications, some with several derivatives already established in the market, and others with potential for such. In this scenario this work aims to describe the properties and potential of these renewable polymers for biomedical applications, the routes from the bench to the market, and the perspectives for future developments.Keywords
Nomenclature
PLLA | Poly(L-lactic acid) |
PDLA | Poly(D-lactic acid) |
PDLLA | Poly(D,L-lactic acid) |
HA | Hydroxyapatite |
PGA | Poly(glycolic acid) |
An increasing demand for new biomaterials for application in the health field has been identified in the last few years. New materials for use in regenerative medicine, tissue engineering, cell therapy and drug delivery can improve the treatments and shorten the recovery time, improving the patient life quality. Several polymers are used in the pharmaceutical industry for the preparation of drug delivery systems. These materials are well established in the market and the advances in the field are growing because of the benefits provided by these systems. Materials for permanent or temporary replacement of damaged tissues represent a great demand in the biomedical field, as well those for cell therapy and tissue engineering, however, few products are available in the market. The research for the development of new materials for use in these areas has undergone great progress, however there is much to be explored and improved in search of property improvements [1,2]. The properties of polymers and biomaterials depend on their chemical structure, directly related to their degradation, mechanical behavior, biocompatibility and toxicity of their degradation products [2,3]. In this scenario, this work addresses the main properties and applications of some polymers that have been widely researched and applied in pharmaceutical, biomedical and regenerative medicine. It starts with vegetable cellulose, a natural polymer with well-established function and application in the pharmaceutical industry, followed by bacterial cellulose, a cellulose produced by bacteria, which has been extensively researched in the biomedical field, with some commercial products for health care available in the market. Starch, is also well established commercially for use in the pharmaceutical industry and extensively studied for applications in regenerative. Chitosan, is also extensively studied for biomedical applications, mainly due to its antimicrobial activity, and poly(lactic acid), a biodegradable polymer, mainly used commercially in the health field for the confection of body degradable devices. All these polymers are under development and improvement for practical applications.
Cellulose is the primary polymeric component of plant cell walls, abundantly found in the biosphere. Historically, cellulose has played a crucial role in the development of human society, including the crucial uses of paper and textiles in our daily lives. Sophisticated technologies have been employed to modify this polysaccharide in order to enhance its properties and expand its range of applications. In the biomedical and health fields, the pharmaceutical industry, in special, benefits from the products generated in its production chains, ranging from simple derivatives like cotton for personal hygiene to chemically modified derivatives used in advanced drug delivery systems [4,5].
Cellulose is a linear homopolymer composed of D-glucose units, with the repeating basic unit being anhydrocellobiose. This unit is formed by the coupling of two glucose rings, which are connected by β-1,4-glycosidic linkages. In its native state, cellulose consists of a mixture of macromolecules with several chain lengths [5]. The linearity and a large number of free hydroxyl functional groups are responsible for the intra- and intermolecular interactions that impart its unique properties, including its high mechanical strength and the lack of solubility in water [4,5].
In plants, cellulose is associated with lignin and hemicelluloses. For use in the biomedical field and other applications, it is necessary to separate it from these macromolecules, normally using a process known as pulping. Pulping involves a series of chemical and/or mechanical treatments. Pulping is followed by bleaching, refining and drying. The chemical-based steps in this sequence present a challenge in maintaining the morphology and features of cellulose, thereby impacting cellulose fiber properties, including aspects such as opacity, softness, and tear strength [6].
Cellulose has a low cost and is readily available. It is biodegradable in nature because the action of the microbial enzymes, however, is extremely slow degradable within the human body, which does not synthezize cellulase enzymes. It is highly biocompatible, poorly soluble in water or other common solvents, and displays a total lack of thermoplasticity. These properties can be useful or represent limitations for certain biomedical applications. Maybe its major disadvantage is its poor processability because the limited solubility generated by the strong intra and intermolecular bonds and the lack of thermoplasticity [7]. Cellulose can be used in its native chemical structure, with few products in the market, such as the microcrystalline cellulose, or chemically modified, which represents most of its based products in the market.
Several cellulose-based materials have been studied for future applications in the biomedical field. For instance, the use of cellulose nanocomposite to prepare contact lens, wound dressings, hydrogels for three-dimensional printing, bone tissue engineering and medical implants, membranes for osseointegration and hemodialysis, among others [7,8] Carboxymethyl cellulose (CMC) is the first and major cellulose derivative, already in the market because it is essential for the pharmaceutical industry, CMC and its based composites have been also described for applications in the biomedical field, including tissue engineering, wound dressing, and drug delivery [9].
An interesting application of chemically modified cellulose in the biomedical field is the use of its oxidized derivative, useful for loading and releasing cells aiming to improve tissue regeneration. The cellulose oxidized with 2,2,6,6-tetramethylpiperidine-1-oxyl (TEMPO) reagent generates a nanofibrillar structure, which keeps the pristine biocompatibility and displays high water affinity, giving rise to high viscous dispersions at very low solid content because the carboxylic acid inserted into its structure, Figs. 1a and 1b. These carboxylic groups at the surface of the cellulose nanofibrils can easily induce their assembly into beads (insert, Fig. 1c) or fibers (Fig. 1e) when cross-linked with cationic charged counter-ions, by electrostatic interactions. These cellulose beads or fibers, when loaded with cells, are capable of keeping them alive. This data indicates its potential as cells carrier for encapsulating and releasing cells as the material is degraded inside the body [10–12]. Figs. 1c–1f show the images of the beads (Fig. 1c-insert) and the fibers (Fig. 1e) prepared from TEMPO oxidized cellulose nanofibers and calcium chloride, and their respective microscopic images showing the cells inside the beads at light field microscopy (Fig. 2c), the nuclei of the cells marked with the fluorescent marker DAPI (Fig. 1d) and the microscopic image of the fiber loaded with cells (indicated by arrows) at light field microscopy (Fig. 1f).
Figure 1: Chemical structure of cellulose (a and its oxidized derivative (b), picture of the oxidized cellulose beads (c-insert), its corresponding light field microscopy (c; scale bar = 50 µm) and the microscopic image of the cell nuclei marked with the fluorescent marker DAPI (d; scale bar = 50 µm), picture of oxidized cellulose fibers (e), and its corresponding microscopic image showing the cells (indicated by arrows) inside the beads using light field microscopy (f; scale bar = 500 µm). (f) was reproduced with permission from [11]. Copyright © SPRINGER NATURE, 2024)
Figure 2: Picture of the bacterial cellulose membrane in its wet state (a), the nanometric structure of the biofilm with the bacteria within the fibers (b), the purified nanofibers at two magnifications (c and d)
Pure unmodified cellulose can be found commercially in several shapes, sizes and degrees of crystallinity. Microcrystalline cellulose is a refined pulp, with the appearance of a white, free-flowing powder, which stands out as the most common and widely utilized form, particularly as an excipient in pharmaceutical formulations. It is inert and is not absorbed by gut tract, which, in large amounts can show a laxative effect. Microcrystalline cellulose acts as a compressibility enhancer, binder in wet and dry granulation processes, thickener and viscosity builder in liquid forms and free-flowing agents in solid dosage forms [13].
The commercial use of cellulose in the biomedical field lays on the pharmaceutical industry, in which its chemically modified derivatives dominate the market and are well-established because of their irreplaceable properties.
The chemical modifications of cellulose are useful to tailor its properties for specific applications. Solubility and thermoplasticity, for instance, are crucial for viscosity modification and coating, respectively, in the pharmaceutical industry, and these properties can be reached by chemical modifications of their native structure. Two main approaches are used for chemical modifications of cellulose, the chemical reaction in bulk and the surface chemical modification. In both cases, the main functional groups available for chemical reactions are the hydroxyl, namely those at C2, C3, and C6 positions of the glucose ring (Fig. 1a). The hydroxyl group at C6 is the most reactive because of the lowest steric hindrance at this position [14].
The bulk reactions lead to the deep modification of the entire fiber, changing its semicrystalline structure and morphology [15]. It is commonly used to prepare thermoplastic cellulose derivatives such as esters and ethers [16,17]. Solubility, crystallinity, and thermoplasticity depend on the degree of substitution, substituent and degree of polymerization of the reaction product [14].
Examples of cellulose ethers are methyl cellulose (MC), ethyl cellulose (EC), hydroxyethyl cellulose (HEC), hydroxypropyl cellulose (HPC), hydroxypropylmethyl cellulose (HPMC), carboxymethyl cellulose (CMC) and sodium carboxymethyl cellulose (NaCMC) [18]. Their solubility, viscosity in aqueous solution, stability (against biodegradation, heat, hydrolysis and oxidation) and features of the thermoplastic film depend on their properties, mainly molecular weight and degree of substitution [13]. Ester derivatives are normally insoluble in water and are filmogenic, thus, widely used in controlled release preparations such as osmotic and enteric coated drug delivery systems [18,19].
The modification in suspension of the cellulose fibers, microfibrils, or nanocrystals results in a thin outer layer modification at its surface, keeping most of its properties and tailoring its solubility and mechanical properties [20]. Many research and reports about surface cellulose modifications and its applications have been available in the last years, however, its based products are not commercially available, indicating a demand for its use and industrial production.
Cellulose is also synthesized by animals such as tunicates and urochordates, and by microorganisms, such as fungi and bacteria [21–23], in small amounts, as a structural component.
Bacterial cellulose (BC) chemical structure is exactly the same as vegetable cellulose. It is produced and secreted extracellularly by the bacteria as nanofibers. The association of the nanofiber leads to the formation of a thick protective biofilm to the microorganism in nature. Interestingly, some bacteria secrete BC in so large amounts, led it to become a food tradition in southern Asia [16] known as nata de coco, however, its report in the literature was found for the first time in 1886 [24]. Nata de coco is produced in high amounts, corresponding to the most common BC based product in the market. Bacteria from the Acetobacteraceae family, specially Gluconobacter, Gluconacetobacter, and Komagataeibacter genera [25–29]. The picture of the bacterial cellulose membrane, the nanometric structure of the biofilm with the bacteria lying within the fibers, the nanofibers purified, free of bacteria in two magnifications and can be seen in Figs. 2a–2d, respectively.
BC biocompatibility, surface compatibility, inert nature and mechanical properties [13], lead it to be studied for application in health care [6,12]. BC exhibits features similar to natural tissues [30] including high mechanical strength, hypo allergenicity, biocompatibility, liquid absorption capacity [26,31], porosity [26,32], the capability of accelerating healing while protecting against external pathogens [27,29,33]. Its biocompatibility, inert nature and mechanical properties are interesting for application in drug delivery systems [27,33,34]. BC has been widely studied for wound dressing development for the treatment of burn injuries because it can decrease the transepidermal water loss and can improve tissue regeneration [14,35]. The scheme in Fig. 3 shows the biomedical application of BC.
Figure 3: Schematic representation of the biomedical application of BC
In the drug delivery field, BC and its derivatives show potential for topical formulation administration. Examples include antimicrobial drugs such as ceftriaxone [31,36], tetracycline [37], amoxicillin [38] and rifampicin [26]. BC based blends with chitosan [39] and chitosan-Ag [40], BC loaded with silver metal complexes with antibacterial and antitumor activities [41,42], silver nanoparticle [43–46], its derivatives [47–49], silver-montmorillonite [50], diclofenac [51], ibuprofen or lidocaine [52]. Other studies report the use of BC as a transdermal delivery system as insulin [53], and oral administration of anticancer drugs such as docetaxel, paclitaxel, and etoposide [54].
Several other applications and materials based on BC have been studied. For instance, Ciecholewska-Juśko et al. [55] developed a cross-linked BC impregnated with octenidine dihydrochloride (OCT) based antiseptic. The material displayed antibiofilm/antibacterial activity, exudate absorption, and absence of cytotoxicity. Its antimicrobial activity extended for up to 7 days, with a reduction of planktonic and biofilm cells. Huang et al. [56] have prepared scaffolds for bone repair using BC loaded with pegylated gold nanoparticles. Their experiments indicated osteogenic activity and good bone repair performance in a rabbit model of femoral defect by autophagy mechanism. A recent in vivo study in a pig model indicated the potential utility of BC as a biological implant for the circulatory system. Poly-vinyl alcohol and hyaluronic acid were used to improve the properties of BC. The results demonstrated that the material exhibited greater durability than animal tissue, good biocompatibility, apyrogenicity, low thrombogenicity, and a low hemolysis index. These findings suggest its potential application as an arterial prosthesis, heart valve prosthesis, or a patch in the pericardial sac in human reconstructive surgery [57].
The products based on BC commercially available for application in the medical field are normally membranes for wound dressing. Examples are Biofill® and Gengiflex®, used respectively for surgery and dental implants, and wound healing for burns and ulcers [58]. Membracell®, Bionext®, and Xcell® products comprise BC membranes for temporary skin substitutes. They are free of drugs and used for healing, providing a physical barrier against adverse environmental effects [59]. Several BC based wound dressings have been commercialized under the trademarks NanodermTM, Bionext, Membracell, Suprasorb, Biofill, Gengiflex and Xcell. Other products, such as EXFILL, DERMAFILL, and CUTICELL EPIGRAFT, Biocel, Bionext®, Membracel® and FibDex® have also emerged, indicating the growing of BC application in the medical field, as well as its potential for further development [60,61].
Starch is a biodegradable polysaccharide from renewable sources, stored in grains and vegetables as an energy source. Vegetables rich in starch include corn, wheat, rice, potato, and cassava. The starch chemical structure comprises functional groups of amylose and amylopectin. The chemical structure of these polysaccharides is shown in Fig. 4. It has been widely used in the pharmaceutical industry due to its physical and chemical properties. In pharmaceutical formulations, it is used as tablets and capsules, as diluent, binder, and lubricant [62,63].
Figure 4: Chemical structure of starch composed by the natural polymers (a) amylose, linear structure with α(1,4) glycosidic bonds and (b) amylopectin, branched structure with α(1,4) and α(1,6) glycosidic bonds [64]
Thermoplastic starch (TPS) is a starch derivative produced by adding a plasticizer to starch under temperature. TPS emerged in the 1990s as a new class of bioplastics derived from renewable and biodegradable sources. It has been much investigated because it is easily modified and displays potential features for use in the packaging industry and biomedical field [65,66]. The main application of thermoplastic starch is the production of biodegradable packaging. Fig. 5 shows the biodegradation potential of thermoplastic starch produced by reactive extrusion with 30% plasticizer and analyzed through mass loss over time under exposure in simulated soil. TPS can be applied as a packaging material specific for biomedical devices and medicines, adding the character of biodegradability and renewable sources to reduce the environmental impact in this area as well [67,68].
Figure 5: Biodegradability of thermoplastic starch on simulated soil exposition with 95% relative humidity and 35°C for nearly four weeks
Despite the advances in the development of TPS compositions, its application is often limited, in some cases, due to its low mechanical strength, high sensitivity to water, chances of its properties due to crystallization during storage, and high melting viscosity during processing. These drawbacks can be overcome by combining TPS with other polymers or incorporating particles or nanoparticles [69–71]. In short, TPS properties can be modified by addition of plasticizers, applying temperature, and shear with or without modifying agents. Its properties can be also tailored in blends, composites, and nanocomposites. TPS is used to prepare films, hydrogels, and 3D printing filaments. Fig. 6 shows the scheme for the preparation of thermoplastic starch from starch, an example of its modification and application of its derivatives products.
Figure 6: Scheme for obtaining thermoplastic starch, its modification and examples of applications and products
TPS displays desired properties such as biocompatibility, biodegradability, low toxicity, low cost and ease of processing, which make it suitable for biomedical uses. Fig. 7 shows the most explored applications of TPS in biomedicine, including drug delivery systems, tissue engineering, wound dressing, and surgical materials.
Figure 7: Biomedical applications and main properties of TPS
Starch-based materials can be used in the production of drug delivery systems to encapsulate and release active substances, to prepare films and gels and hydrogels to be used in the biomedical field, as well to prepare TS, which increases the starch applications range. Studies reporting the use of starch to produce hydrogels, nanocomposite films, and coatings, followed by the applications of TPS are described below.
Starch is described as one of the most biocompatible and ecologically correct polysaccharides for synthesizing advanced materials, such as pH-sensitive smart films. Nanoparticles can be incorporated into starch-based air gel, improving its properties for biomedical purposes due to its easy preparation, porous structure, high surface area, and low density [72]. Starch is also applied in tissue engineering scaffolds, biosensors, wound dressing, and other biomedical devices [73,74].
Biodegradable hydrogels are used to develop new matrices for drug delivery with plasticizers and nanoparticles. It improves the mechanical and release properties of the hydrogel for drug delivery. An example is the modification of starch with glycolic compounds and nanoparticles to improve quercetin release, reaching about 57% in 8 h [75].
Hydrogel beads based on starch and carboxymethylcellulose (CMC) nanocomposites with ZnO nanoparticles, crosslinked in solution with FeCl3, were loaded with the anticancer drug doxorubicin. The morphology and behavior of the material were studied by researchers who verified the effectiveness of drug release as a function of the concentration of nanoparticles, placing the CMC/Starch/ZnO nanocomposite beads like a new bio-compatible carrier for controlled delivery of anticancer drugs [76]. A starch-based nanocomposite hydrogel graphitized with itaconic acid in the presence of Fe3O4 nanoparticles was applied as a pH-sensitive magnetic hydrogel to release Guaifenesin, which is used in wound healing. The structure of hydrogels and drug release behavior in response to pH variation and magnetic field application were studied, providing a promising platform for drug delivery systems and enabling the administration of magnetically activated drug delivery [77].
Nanofibers based on starch can be produced by electrospin for application in skin or cartilage tissue engineering. The biodegradability, biocompatibility, and mechanical properties of biomaterials used in wound healing must be improved to promote the fixation and proliferation of the cells. Core-shell nanofibers of polyurethane/starch (PU/St) with the addition of hyaluronic acid (HA) were produced with coaxial electrospinning and characterized in terms of morphology, structure, and porosity, in addition to evaluating their biocompatibility in vitro and in vivo. The study suggests that core-shell PU/St (HA) nanofibers may be an appropriate candidate for wound healing in cutaneous tissue engineering. One of the significant challenges in the area is the healing of joint cartilage, which is a load-bearing connective tissue with a low regeneration rate and slow metabolism. Studies focus on developing fibrous structures for cartilage tissue engineering, which could potentially improve the healing process of cartilage tissue. Fibrous scaffolds of poly(hydroxybutyrate) and starch reinforced with halloysite nanotubes had their properties evaluated in vitro and proved to be promising for application in the area, but more studies are needed to fully evaluate their potential and limitations [78,79].
Films and fibers based on starch are viable materials for the production of wound dressings, primarily owing to their vapor permeability, hydrophilicity, and biocompatibility. These materials facilitate a moist environment, expediting the healing process, and can be applied and removed without causing trauma to the wound site. Nanocomposites comprising silver sulfadiazine-halloysite/cassava-loaded starch biodegradable films exhibited satisfactory mechanical properties and permeability, rendering them suitable for use as wound dressings. Furthermore, these films demonstrated antimicrobial efficacy against bacteria commonly found in hospitals, namely Escherichia coli, Pseudomonas aeruginosa, and Staphylococcus aureus. Considering the frequent replacement of several layers of gauze and cotton in the treatment of burns and wounds using silver sulfadiazine, the starch-based nanocomposite film presents a more practical and efficient solution for this treatment [80].
The materials used to prepare wound dressings have been modernized over time, and nanofibers based on electrospinning biopolymers represent a potential solution for rapid wound healing due to their porous structure and high surface area to volume ratio. Nanofibers based on starch and thermoplastic polyurethane, prepared by electrospinning and crosslinked with glutaraldehyde, were characterized and showed better healing properties than traditional cotton-based dressings [81].
Starch hydrogels are also applied to wound dressings. Hybrid hydrogels of starch and gelatin loaded with silver nanoparticles were studied and the results indicated that the porous hydrogel acted as an organic nanoreactor for the generation and stabilization of silver nanoparticles. The process avoids the use of toxic reducing agents and acts as a stabilizing agent for silver nanoparticles. The nanocomposite hydrogel showed adequate mechanical properties, is hydrophilic, biodegradable, non-cytotoxic, and hemocompatible, being useful as a curative material for wounds [82].
Meanwhile, TPS in the form of film can be effectively applied in drug release, being produced simply via casting. Researchers described the chlorhexidine extended release up to 500 h using TPS films, which showed antimicrobial potential for inhibiting the growth of Staphylococcus aureus [83].
TPS is widely used in tissue engineering as a scaffold, providing a biocompatible and biodegradable matrix to support the adhesion and proliferation of cells. The most studied properties of starch are the porosity, mechanical resistance, and degradation rate and processing in order to determine the specific requirements in the area. TPS is also used to form blends with other polymers for application in tissue engineering. Studies are carried out to optimize the mechanical, morphological, and biological properties of scaffolds based on TPS blends with other biodegradable polymers such as polylactic acid (PLA) and polyhydroxy butyrate, for example. The advantage of TPS, when added to other polymers for the confection of scaffolds, is that it has hydroxyl groups in its structure. It has a highly hydrophilic character, which helps in the rate of biodegradation of the end product. The production of scaffolds via additive manufacture, particularly for use in 3D printers, has been highlighted because of the improved control over architecture and porosity, leading to a more precise direction of cell growth. Producing structures with high geometric control and porosity represents one of the biggest challenges in this field. Tubular structures, such as those for urethra or blood vessels repair, as an example.
TPS/PLA/PHB blend filament for 3D printing can be used to produce scaffolds that create a conducive environment for cell proliferation. These scaffolds exhibit a highly porous structure, and the modulation of scaffold swelling is achieved by manipulating the concentration and viscosity of TPS. The controlled porous modulation is advantageous because its swelling can enlarge the pore size and facilitate the migration of proliferating cells. However, it is crucial to avoid excessive water absorption to prevent any adverse impact on the mechanical properties and supportive function of the scaffold [84]. Starch can also be a polymeric matrix for the fabrication of three-dimensional scaffolds via sol-gel and 3D printing techniques. As an example, scaffolds composed of starch and gellan gum have been evaluated for their efficacy in supporting the growth of Schwann cells. These scaffolds show promise for potential applications in the creation of artificial implants intended for peripheral nerve regeneration [85].
TPS/β-tricalcium phosphate (β-TCP) nanocomposites have also been described for the preparation of scaffolds. The composites were prepared by extrusion and molded by injection, improving the tensile strength and modulus of TPS. In addition to mechanical properties, bioactivity was investigated through hydroxyapatite nucleation, and no negative effects were observed in the toxicity test. The recently reported results open the way for further studies of these nanocomposites in application as bone tissue engineering material [86].
TPS can be used in the composition of surgical sutures such as wires and implants for bone repair, improving patient outcomes. It can also be used in preparation of absorbable polymer blends as an alternative to metallic orthopedic implants for plates and screws. The advantage of replacement of the non-degradable implants is to avoid surgical procedures to remove them. Polycaprolactone (PCL) is a biodegradable polymer that could be used for such; however, its surface chemistry does not promote cell adhesion. PCL blends with other polymers, such as TPS, among others, have been shown potential applications for the preparation of biocompatible and absorbable materials for the replacement of low-load fracture fixation devices, such as maxillofacial surgery, orthopedics, and neurosurgery [87].
Surgical threads have been produced using natural biopolymers, specifically Nopal mucilage combined with starch and plasticizers. The outcome was the achievement of threads with consistent diameter, excellent elasticity, and effective capillarity [88].
Table 1 shows recent references, starting from 2020, related to the studies on starch or TPS applications in the four previously described fields. It includes the application of starch-based materials in drug-delivery systems and wound dressings, widely used in this field in the form of microparticles and nanoparticles, hydrogels and aerogels while TPS is used in the form of films and coatings, scaffolds, sutures, plates and screws.
The use of starch is firmly established in the pharmaceutical industry, particularly in the formulation of controlled drug delivery systems. While thermoplastic starch has demonstrated significant applications in the biomedical field, including materials for tissue engineering, wound dressing, and surgical materials, the corresponding studies are relatively recent. However, it is challenging to identify these products as commercially available, despite their promising potential in various biomedical applications. Moreover, while exhibiting biocompatibility, keeping the thermoplastic starch stable at large-scale processing may represent a challenge, as well as the regulatory hurdles within the market. Also, because of concerns related to competition and the safeguarding of intellectual property, companies often share information about innovations in the technologies and functions of the devices, without providing details regarding composition innovations.
Chitosan (poly β (1→4)-2-amino-2-deoxy-D-glucopyranose), Fig. 8 is a polysaccharide generated by partial deacetylation of chitin, found in the shells of insects and crustaceans [89,90]. Fig. 8 shows the chemical structure of chitin (a) and chitosan (b).
Figure 8: Chemical structure of chitin (a) and the partially deacetylated chitosan (b)
Chitosan is the only polycationic polysaccharide directly derived from a natural source. Its polycationic nature results from predominantly positively charged amino groups at pH values below 6.5 [91]. It is biocompatible, biodegradable, non-toxic and displays antimicrobial activity, triggering the interest for its application in the biomedical field.
The antimicrobial properties of chitosan add value to its use as wound dressing, surpassing the function of traditional dressings that only provide a protective barrier function to the wounded area. Chitosan-based wound dressing can be prepared as films or hydrogels. The films are thin, flexible and low obtrusive, increasing the patient acceptance [92]. Hydrogels are formed by a three-dimensional network capable of absorbing and keeping water, and displays good plasticity and elasticity. The hydrogels can provide a moist environment for wound healing, absorbing wound exudate and are easily removable without secondary trauma [93].
In order to enhance chitosan antimicrobial activity, antibiotics have been successfully encapsulated into its based hydrogels or nanocomposites designed for wound dressing [94–96]. For instance, amoxicillin was incorporated in a composite material based on N-carboxyethyl chitosan and oxidized hyaluronic acid-graft-aniline tetramer polymer, which avoided the wound infection [97]. Nanocomposites based on chitosan, polyethylene oxide nanofibers, silver and zinc oxide nanoparticles exhibited high antioxidant effect and antibacterial activity against Staphylococcus aureus, Escherichia coli, and Pseudomonas aeruginosa [98]. The all-natural, injectable, self-healing hydrogel dressing composed of hydroxypropyltrimethyl ammonium chloride chitosan and dialdehyde modified bacterial cellulose displayed increased antibacterial properties without incorporation of antibiotics [99].
Chitosan derivatives loaded with antiseptic are also described in the literature, for instance, the polyvinyl alcohol chitosan-based wound dressing. Two distinct formulations were prepared and the dressings were loaded with chlorhexidine and polyhexanide. The application of these dressings in the treatment of wounds in a dog led to faster recovery than conventional treatment, suggesting that the material can be a promising alternative for wound care [100].
A cross-linked hydrogel comprising carboxymethyl chitosan, oxidized dextran, and poly-γ-glutamic acid can be utilized as an antimicrobial and hemostatic agent for diffuse wounds. In the hydrogel, poly-γ-glutamic acid effectively removed the surface moisture of the wound to enhance the surface adhesion. In vivo studies showed that the hydrogel significantly inhibited bacterial growth and promoted wound healing [101].
Chitosan-based materials have been extensively studied for drug delivery. Chitosan limitations such as the low solubility in physiological fluids, poor affinity for hydrophobic drugs, and the burst release attributed to its high swelling ratio [102,103], can be overcome by chemical modifications or by the preparation of vesicular or nanoparticles systems, widening its applications in this field.
Chitosan-based formulations with antitumor activity particularly interesting, given the worldwide cancer contribution to premature mortality [104–106] the antitumor activity of zero-valent selenium nanoparticles stabilized by chitosan and oligosaccharides [107]. Also, iron oxide nanoparticles coated with chitosan-[2-(methacryloyloxy) ethyl] trimethyl ammonium chloride can be used as a carrier for the hydrophobic antitumor drug paclitaxel. Boronated chitosan/alginate nanoparticles can be used as a mucoadhesive system for the delivery of paclitaxel for cervical cancer treatment [108,109].
The injectable hydrogel based on chitosan grafted to polyurethane side chains, can be used to encapsulate and release antibacterial tetracycline hydrochloride and anti-cancerous doxorubicin hydrochloride. The pH-responsive lignin/ chitosan nanoparticles loaded with docetaxel and curcumin showed efficient release of antitumoral drugs at weakly acidic environment stimulated by the tumor [102].
There are also many formulations for drug delivery of antibiotics developed with the aim of improving their efficiency and overcoming bacterial resistance [110]. In this context, ciprofloxacin loaded with polydopamine nanoparticles combined with glycol-chitosan was developed to create an injectable near-infrared light-triggerable thermo-sensitive hydrogel. This hydrogel was designed to enable on-demand release of antibiotics and hyperthermia-assisted bacterial inactivation, aiming to address bacterial infections and facilitate wound healing [111,112]. A controlled release system for the delivery of the contraception drug levonorgestrel was also developed. The drug was encapsulated into cross-linked chitosan microspheres, which were incorporated into a matrix of physically cross-linked PVA, achieving a long-term and controlled release system [113].
The large number of patents on chitosan-based materials for the controlled release of drugs highlights the concentrated efforts directed towards entering the pharmaceutical market [114–117]. However, besides its promising properties for medical and healthcare applications, few commercial products including chitosan or its derivatives are available. Wound dressings, nasal sprays or gels and hemostatic represent the most important types of its based commercial products. Wound dressing stands out considering the number of products that have already managed to pass the tests required to be accepted for use in humans and are commercially available, for instance, HemCon® (HemCon Medical Technologies, Inc., Portland, Oregon, USA), Opticell® (Medline Industries LP, IL, USA), QuikClot® (Z-Medica Corporation, Wallingford, Wayne, USA), Axiostat® (Axio Biosolutions Pvt Ltd, Ahmedabad, India), ChitoDerm® (Trusetal Verbandstoffwerk GmbH, Holte-Stukenbrock, Germany) and CELOX™ (SAM Medical, Tualatin, Tel Aviv, Israel) [118,119]. Chitosan can bind to the negatively charged surface of red blood cells promoting clotting, which explains its use for external control of hemorrhage.
Lactic acid, (2-hydroxypropionic acid), is the monomer used as the precursor for the synthesis of PLA. Lactic acid is an organic acid, widely used in the food, pharmaceutical, cosmetic, textile, leather and chemical industries [120]. It is one of the simplest chiral molecules, found in the form of two stereoisomers, L- or D-lactic acid, differentiated by the effect of polarized light [121]. It can be produced by chemical synthesis or microbial fermentation.
The chemical synthesis of lactic acid is mainly based on the hydrolysis of lactonitrile, a petrochemical derivative, by the action of strong acids, which provides the racemic mixture of D- and L-lactic acid [122].
The production of lactic acid by biotechnological processes can be performed by bacteria, such as those from the genus Lactobacillus [123]. When synthesized by live organisms, the biological route leads preferentially to L-lactic acid. The interest in fermentative lactic acid production has increased because the prospects related to the production of the lactic acid-based polymer, PLA, which is fully biodegradable in nature, thus representing a great environmental appeal. The main advantages of synthesis by fermentation over chemical synthesis, are (i) the low environmental impact process and high yield, and (ii) the use of renewable resources raw materials, such as starch, cellulose, sugar cane, among others, as nutrient source for the microorganisms [124].
PLA is an aliphatic polyester, as shown in Fig. 9, with the chemical formula (C3H4O2)n. It has two stereoisomers: poly(L-lactic acid), (PLLA) and poly(D-lactic acid), (PDLA). The racemic mixture generates poly(D,L-lactic acid) (PDLLA) [125]. PDLA and PLLA are mirror images of each other, both optically pure and semi-crystalline [126], while PDLLA is racemic, amorphous and optically inactive [127].
Figure 9: Chemical structure of poly(lactic acid)
The synthesis of PLA can occur by several chemical routes including polycondensation, direct polymerization or ring-opening polymerization. The most commonly used routes for the production of PLA are polycondensation and ring-opening polymerization [128]. The polycondensation process is carried out in vacuum, under high temperature and with the use of solvents for the extraction of water produced by the condensation reaction. Ring-opening polymerization starts with the cyclic lactic acid dimer, the lactide, in the presence of a catalyst. This process generates a low molecular weight polymer. As any other synthetic route, the conditions such as time, temperature and catalyst concentration can be controlled aiming to change the proportions and sequences of D- and L-lactic units in the polymer Fig. 10 [129,130]. Polycondensation of lactic acid results in a low molecular weight polymer, while ring-opening polymerization leads to the formation of higher molecular weight polymers.
Figure 10: Synthesis routes of PLA
Due to its mechanical properties, thermoplastic processability and biological properties, such as biocompatibility and hydrolytic biodegradation, PLA is a promising polymer [131] for replacement of conventional polymers in everyday use, and for biological applications. The properties of PLA depend on the isomeric components resulting from the chirality of lactic acid, where the two asymmetric centers exist in four different forms. These properties can be modified by varying the isomers (L (+)/D (−)) and the homo (D (−), L (+)), the processing temperature, the annealing time and the molecular mass [132]. PLA can be amorphous or crystalline depending on the stereochemistry and its thermal history. Fully amorphous material can be synthesized by the relative increase of the D isomer (>20%), whereas crystalline material is obtained when the amount of D is <2%. This polymer is relatively rigid with glass transition temperature between 60°C–70°C and melting temperature between 170°C–180°C [131].
Polyesters, including PLA, can be hydrolytically degradable. Hydrolysis of PLA breaks its ester bonds, generating carboxyl and hydroxyl end groups as depicted in Fig. 11 [133]. In living organisms, the lactic acid generated by PLA degradation is incorporated into the citric acid cycle, resulting, at the end of the metabolic process, in by-products such as carbon dioxide and water [134]. Degradation rates are determined by factors such as implant site, molar mass, stereochemical composition, crystallinity and morphology [135].
Figure 11: Scheme of the hydrolytic degradation of PLA
PLA is a promising polymer, however, certain properties including poor ductility, slow degradation rate and high hydrophobicity limit its applications. Others, such as biocompatibility and non-toxic degradation products, make it an attractive option for the biomedical industry. It finds uses as a surgical implant material, drug delivery system and as porous support for cell and tissues growth, particularly in the field of regenerative medicine [136].
PLA can be associated with other biomaterials aiming to improve its properties and increase its range of applications. It has been applied in bioprinting, for the preparation of scaffolds for tissue repair of meniscus, for guiding bone regeneration, for regeneration of peripheral nerve and spinal cord injuries, for the production of bioabsorbable screws, cardiac stents, suture threads, dermal fillers, hernia mesh and drug delivery, among others [137–140].
Although research covers a wide range of applications, there are currently not many PLA biomaterials on the market. However, in addition to the products currently available on the market, because it has the versatility to be mixed or copolymerized with other polymeric or non-polymeric components, the future of PLA in the biomedical field is focused on the production of implants, especially in the development of additive manufacturing (AM) devices [141–143]. Table 2 describes the biomaterials used in the medical field, as well as their applications and trade names.
The described polymers are widely studied because of their potential for the development of advanced materials based on new technologies and new requirements in health care. New diseases require new treatments and new materials, and classical diseases require new technologies and new options of treatments. Cellulose and its derivatives are widely used in the pharmaceutical industry for drug delivery and coatings. Even though its use is well consolidated, the research is growing because of the versatility of this material including its properties in natura and the properties of its derivatives.
Starch is also used in the pharmaceutical industry, mainly for drug delivery. In its native form, starch has long been used due to its availability and properties. The use of thermoplastic starch broadens the range of applications of starch because it can be shaped into films, gels, aerogels, fibers, and can also be employed in the preparation of blends and composites. Several scientific studies investigate the application of these materials as biomedical products for tissue engineering, wound dressing, and surgical material. However, its industrial production is limited, and advancements are needed to scale up the processes.
There are few chitosan-based products on the market. The number of researches on this topic is increasing, primarily because of its antimicrobial properties and low solubility in water.
PLA is biodegradable and displays good properties for processing. It can be processed as other thermoplastic materials, which is interesting for the industry and has been increasing the range of available products in the market, particularly in the biomedical field.
The large number of published patents concerning products for biomedical applications including these, and other polymers and their derivatives, indicates the interest in developing products with potential to reach the market. It is crucial to acknowledge, however, that the transition from research and development to the commercialization of new medical products is a time-consuming process that can span several years. Not all research findings necessarily culminate in successful market products, as the feasibility and safety of these products require rigorous evaluation and clinical testing before introduction to the market.
The future perspectives are strictly linked to the rapid advances in the scale up and manufacture of these polymers, including the additive manufacture technology, increasing the potential application of TPS, PLA and other biocompatible polymers. Innovations in processing hold the promise of producing customized multifunctional devices to meet the growing healthcare needs of an expanding population.
Acknowledgement: The authors thank SPRINGER NATURE, for the permissions to reproduce images in Fig. 1.
Funding Statement: The authors acknowledge FAPESP for funding the Research Project Number 2017-18-782-6 and the Grant 2021/07458-9.
Author Contributions: The authors confirm their contribution to the article as follows: conception and writing: ET. The preparation of the draft and writing on Bacterial Cellulose: WRL. Preparation of the draft and writing on Starch: TSN. Draft preparation and writing on Chitosan: MI and GL. Preparation the draft and writing on PLA, formatting the manuscript: RCL. All the authors reviewed the results and approved the final version of the manuscript.
Availability of Data and Materials: Not applicable.
Conflicts of Interest: The authors declare that they have no conflicts of interest to report regarding the present study.
References
1. Willerth SM, S-ESE. Combining stem cells and biomaterial scaffolds for constructing tissues and cell delivery. StemBook: Harvard Stem Cell Institute; 2008. [Google Scholar]
2. Nair LS, Laurencin CT. Biodegradable polymers as biomaterials. Prog Polym Sci. 2007;32(8–9):762–98. [Google Scholar]
3. Ratner BD, Hoffman AS, Schoen FJ, Lemons JE. Biomaterials science: an introduction to materials in medicine. Biomater Sci. 2004;1:1–19. [Google Scholar]
4. Klemm D, Kramer F, Moritz S, Lindström T, Ankerfors M, Gray D, et al. Nanocelluloses: a new family of nature-based materials. Angew Chem Int Ed Engl. 2011;50(24):5438–66 [Google Scholar] [PubMed]
5. Klemm D, Philipp B, Heinze T, Heinze U, Wagenknecht W. General considerations on structure and reactivity of cellulose. In: Comprehensive cellulose chemistry. Weinheim: Wiley; 1998. [Google Scholar]
6. Sixta H. Handbook of pulp. Germany: Wiley; 2006. [Google Scholar]
7. Oprea M, Voicu SI. Recent advances in composites based on cellulose derivatives for biomedical applications. Carbohydr Polym. 2020;247:116683 [Google Scholar] [PubMed]
8. Joseph BKSV, Sabu C, Kalarikkal N, Thomas S. Cellulose nanocomposites: fabrication and biomedical applications. J Bioresour Bioprod. 2020;5(4):223–37. [Google Scholar]
9. Pourmadadi M, Rahmani E, Shamsabadipour A, Samadi A, Esmaeili J, Arshad R, et al. Novel carboxymethyl cellulose based nanocomposite: a promising biomaterial for biomedical applications. Process Biochem. 2023;130:211–26. [Google Scholar]
10. de Carvalho RA, Veronese G, Carvalho AJF, Barbu E, Amaral AC, Trovatti E. The potential of TEMPO-oxidized nanofibrillar cellulose beads for cell delivery applications. Cellul. 2016;23:3399–405. [Google Scholar]
11. Rios MA, Barbugli PA, Iemma MRC, Grande R, Carvalho AJF, Trovatti E. TEMPO-oxidized cellulose poly-ionic drawn fiber, a cell support system proof of concept. J Mater Sci. 2021;56:16661–70. [Google Scholar]
12. Cândido deLPA, Fregonezi NF, Carvalho AJF, Trovatti E, Resende FA. TEMPO-oxidized cellulose nanofibers in vitro cyto-genotoxicity studies. Bionanosci. 2020;10:766–72. [Google Scholar]
13. Shokri J, Adibki K. Application of cellulose and cellulose derivatives in pharmaceutical industries. In: Cellulose—medical, pharmaceutical and electronic applications. InTech; 2013. Available from: https://www.intechopen.com/books/3173 [Accessed 2023]. [Google Scholar]
14. Pérez S, Samain D. Structure and engineering of celluloses. Adv Carbohydr Chem Biochem. 2010;64:25–116. [Google Scholar]
15. Belgacem MN, Gandini A. Surface modification of cellulose fibres. In: Monomers, polymers and composites from renewable resources. Oxford, UK: Elsevier; 2008. [Google Scholar]
16. Trovatti E. The future of bacterial cellulose and other microbial polysaccharides. J Renew Mater. 2013;1:28–41. [Google Scholar]
17. Gandini A. The irruption of polymers from renewable resources on the scene of macromolecular science and technology. Green Chem. 2011;13(5):1061. [Google Scholar]
18. Dias F, Duarte C. Cellulose and its derivatives use in the pharmaceutical compounding practice. In: Cellulose—medical, pharmaceutical and electronic applications. InTech; 2013. Available from: https://www.intechopen.com/books/3173 [Accessed 2023]. [Google Scholar]
19. Bifari NE, Khan BS, Alamry KA, Asiri MA, Akhtar K. Cellulose acetate based nanocomposites for biomedical applications: a review. Curr Pharm Des. 2016;22(20):3007–19 [Google Scholar] [PubMed]
20. Klemm D, Philipp B, Heinze T, Heinze U, Wagenknecht W. Comprehensive cellulose chemistry. Weinheim: Wiley; 1998. [Google Scholar]
21. Brenner DJ, Krieg NR, Staley JT, Garrity GM, editors. Bergey’s manual® of systematic bacteriology. Boston, MA: Springer US; 2005. [Google Scholar]
22. Kimura S, Itoh T. New cellulose synthesizing complexes (terminal complexes) involved in animal cellulose biosynthesis in the tunicate Metandrocarpa uedai. Protoplasma. 1996;194:151–63. [Google Scholar]
23. Sanxa IM, Brown RM. Cellulose biosynthesis: current views and evolving concepts. Ann Bot. 2005;96(1):9–21. [Google Scholar]
24. Brown AJ. XLIII.—on an acetic ferment which forms cellulose. J Chem Soc, Trans. 1886;49:432–9. [Google Scholar]
25. Lustri WR, Barud HGDO, Barud HDS, Peres MFS, Gutierrez J, Tercjak A, et al. Microbial cellulose—biosynthesis mechanisms and medical applications. In: Cellulose—fundamental aspects and current trends. United Kingtom: InTech; 2015. [Google Scholar]
26. Lazarini SC, Yamada C, da Nóbrega TR, Lustri WR. Production of sphere-like bacterial cellulose in cultivation media with different carbon sources: a promising sustained release system of rifampicin. Cellul. 2022;29:6077–92. [Google Scholar]
27. Samyn P, Meftahi A, Geravand SA, Heravi MEM, Najarzadeh H, Sabery MSK, et al. Opportunities for bacterial nanocellulose in biomedical applications: review on biosynthesis, modification and challenges. Int J Biol Macromol. 2023;231:123316 [Google Scholar] [PubMed]
28. Kaczmarek M, Jędrzejczak-Krzepkowska M, Ludwicka K. Comparative analysis of bacterial cellulose membranes synthesized by chosen komagataeibacter strains and their application potential. Int J Mol Sci. 2022;23(6):3391 [Google Scholar] [PubMed]
29. Barja F. Bacterial nanocellulose production and biomedical applications. J Biom Res. 2021;35:310. [Google Scholar]
30. Pang M, Huang Y, Meng F, Zhuang Y, Liu H, Du M, et al. Application of bacterial cellulose in skin and bone tissue engineering. Eur Polym J. 2020;122:109365. [Google Scholar]
31. Lazarini SC, Yamada C, Barud HS, Trovatti E, Corbi PP, Lustri WR. Influence of chemical and physical conditions in selection of Gluconacetobacter hansenii ATCC 23769 strains with high capacity to produce bacterial cellulose for application as sustained antimicrobial drug-release supports. J Appl Microbiol. 2018;125(3):777–91 [Google Scholar] [PubMed]
32. Ullah H, Badshah M, Mäkilä E, Salonen J, Shahbazi MA, Santos HA, et al. Fabrication, characterization and evaluation of bacterial cellulose-based capsule shells for oral drug delivery. Cellul. 2017;24:1445–54. [Google Scholar]
33. Chen C, Ding W, Zhang H, Zhang L, Huang Y, Fan M, et al. Bacterial cellulose-based biomaterials: from fabrication to application. Carbohydr Polym. 2022;278:118995 [Google Scholar] [PubMed]
34. Janmohammadi M, Nazemi Z, Salehi AOM, Seyfoori A, John JV, Nourbakhsh MS, et al. Cellulose-based composite scaffolds for bone tissue engineering and localized drug delivery. Bioact Mater. 2023;20:137–63 [Google Scholar] [PubMed]
35. Holzer JCJ, Tiffner K, Kainz S, Reisenegger P, de Mattos IB, Funk M, et al. A novel human ex-vivo burn model and the local cooling effect of a bacterial nanocellulose-based wound dressing. Burns. 2020;46(8):1924–32 [Google Scholar] [PubMed]
36. Lazarini SC, de Aquino R, Amaral AC, Corbi FCA, Corbi PP, Barud HS, et al. Characterization of bilayer bacterial cellulose membranes with different fiber densities: a promising system for controlled release of the antibiotic ceftriaxone. Cellul. 2016;23:737–48. [Google Scholar]
37. Shao W, Liu H, Wang S, Wu J, Huang M, Min H, et al. Controlled release and antibacterial activity of tetracycline hydrochloride-loaded bacterial cellulose composite membranes. Carbohydr Polym. 2016;145:114–20 [Google Scholar] [PubMed]
38. Pavaloiu RD, Stoica A, Stroescu M, Dobre T. Controlled release of amoxicillin from bacterial cellulose membranes. Open Chem. 2014;12(9):962–7. [Google Scholar]
39. Dragostin OM, Samal SK, Dash M, Lupascu F, Pânzariu A, Tuchilus C, et al. New antimicrobial chitosan derivatives for wound dressing applications. Carbohydr Polym. 2016;141:28–40 [Google Scholar] [PubMed]
40. Anwar Y, Ul-Islam M, Mohammed AHSH, Ullah I, Khalil A, Kamal T. Silver impregnated bacterial cellulose-chitosan composite hydrogels for antibacterial and catalytic applications. J Mater Res Technol. 2022;18:2037–47. [Google Scholar]
41. Aquaroni NAS, Nakahata DH, Lazarini SC, Resende FA, Cândido ALP, da Silva Barud H, et al. Antibacterial activities and antiproliferative assays over a tumor cells panel of a silver complex with 4-aminobenzoic acid: studies in vitro of sustained release using bacterial cellulose membranes as support. J Inorg Biochem. 2020;212:111247 [Google Scholar] [PubMed]
42. Candido TZ, de Paiva REF, Figueiredo MC, de Oliveira Coser L, Frajácomo SCL, Abbehausen C, et al. Silver nimesulide complex in bacterial cellulose membranes as an innovative therapeutic method for topical treatment of skin squamous cell carcinoma. Pharm. 2022;14(2):462. [Google Scholar]
43. Jung R, Kim Y, Kim HS, Jin HJ. Antimicrobial properties of hydrated cellulose membranes with silver nanoparticles. J Biomater Sci Polym Ed. 2009;20(3):311–24 [Google Scholar] [PubMed]
44. Ma B, Chaudhary JP, Zhu J, Sun B, Chen C, Sun D. Construction of silver nanoparticles anchored in carbonized bacterial cellulose with enhanced antibacterial properties. Colloids Surf A Physicochem Eng Asp. 2021;611:125845. [Google Scholar]
45. Jiji S, Udhayakumar S, Maharajan K, Rose C, Muralidharan C, Kadirvelu K. Bacterial cellulose matrix with in situ impregnation of silver nanoparticles via catecholic redox chemistry for third degree burn wound healing. Carbohydr Polym. 2020;245:116573 [Google Scholar] [PubMed]
46. Barud HS, Regiani T, Marques RFC, Lustri WR, Messaddeq Y, Ribeiro SJL. Antimicrobial bacterial cellulose-silver nanoparticles composite membranes. J Nanomater. 2011;2011:1–8. [Google Scholar]
47. Munhoz LLS, Alves MTO, Alves BC, Nascimento MGFS, Sábio RM, Manieri KF, et al. Bacterial cellulose membrane incorporated with silver nanoparticles for wound healing in animal model. Biochem Biophys Res Commun. 2023;654:47–54 [Google Scholar] [PubMed]
48. Ma L, Jiang W, Xun X, Liu M, Han X, Xie J, et al. Homogeneous silver nanoparticle loaded polydopamine/polyethyleneimine-coated bacterial cellulose nanofibers for wound dressing. Int J Biol Macromol. 2023;246:125658 [Google Scholar] [PubMed]
49. Barjasteh M, Dehnavi SM, Ahmadi Seyedkhani S, Rahnamaee SY, Golizadeh M. Improved biological activities of dual nanofibrous chitosan/bacterial cellulose wound dressing by a novel silver-based metal-organic framework. Surf Interf. 2023;36:102631. [Google Scholar]
50. Horue M, Cacicedo ML, Fernandez MA, Rodenak-Kladniew B, Torres Sánchez RM, Castro GR. Antimicrobial activities of bacterial cellulose—silver montmorillonite nanocomposites for wound healing. Mater Sci Eng C. 2020;116:111152. [Google Scholar]
51. Silva NHCS, Rodrigues AF, Almeida IF, Costa PC, Rosado C, Neto CP, et al. Bacterial cellulose membranes as transdermal delivery systems for diclofenac: in vitro dissolution and permeation studies. Carbohydr Polym. 2014;106:264–9 [Google Scholar] [PubMed]
52. Trovatti E, Freire CSR, Pinto PC, Almeida IF, Costa P, Silvestre AJD, et al. Bacterial cellulose membranes applied in topical and transdermal delivery of lidocaine hydrochloride and ibuprofen: in vitro diffusion studies. Int J Pharm. 2012;435(1):83–7 [Google Scholar] [PubMed]
53. Wu Z, Xie S, Kang Y, Shan X, Li Q, Cai Z. Biocompatibility evaluation of a 3D-bioprinted alginate-GelMA-bacteria nanocellulose (BNC) scaffold laden with oriented-growth RSC96 cells. Mater Sci Eng C. 2021;129:112393. [Google Scholar]
54. Raghav N, Sharma MR, Kennedy JF. Nanocellulose: a mini-review on types and use in drug delivery systems. Carbohydr Polym Technol Appl. 2021;2:100031. [Google Scholar]
55. Ciecholewska-Juśko D, Junka A, Fijałkowski K. The cross-linked bacterial cellulose impregnated with octenidine dihydrochloride-based antiseptic as an antibacterial dressing material for highly-exuding, infected wounds. Microbiol Res. 2022;263:127125 [Google Scholar] [PubMed]
56. Huang C, Ye Q, Dong J, Li L, Wang M, Zhang Y, et al. Biofabrication of natural Au/bacterial cellulose hydrogel for bone tissue regeneration via in-situ fermentation. Smart Mater Med. 2023;4:1–14. [Google Scholar]
57. Kołaczkowska M, Siondalski P, Kowalik MM, Pęksa R, Długa A, Zając W, et al. Assessment of the usefulness of bacterial cellulose produced by Gluconacetobacter xylinus E25 as a new biological implant. Mater Sci Eng C. 2019;97:302–12. [Google Scholar]
58. Pacheco JLC, Yee SM, Zentella MC, Marvan JEE. Celulosa bacteriana en gluconacetobacter xylinum: biosíntesis y aplicaciones. TIP Revista Especializada en Ciencias Químico-Biológicas. 2004;7(1):18–25. [Google Scholar]
59. Picheth GF, Pirich CL, Sierakowski MR, Woehl MA, Sakakibara CN, de Souza CF, et al. Bacterial cellulose in biomedical applications: a review. Int J Biol Macromol. 2017;104:97–106 [Google Scholar] [PubMed]
60. Abdullah SSS, Aris FFA, Azmi SSNN, John AJHS, Anuar KNN, Asnawi MASF. Development and evaluation of ciprofloxacin-bacterial cellulose composites produced through in situ incorporation method. Biotechnol Rep. 2022;34:e00726. [Google Scholar]
61. Mujtaba M, Negi A, King AWT, Zare M, Kuncova-Kallio J. Surface modifications of nanocellulose for drug delivery applications; a critical review. Curr Opin Biomed Eng. 2023;28:100475. [Google Scholar]
62. Sivamaruthi BS, Nallasamy PK, Suganthy N, Kesika P, Chaiyasut C. Pharmaceutical and biomedical applications of starch-based drug delivery system: a review. J Drug Deliv Sci Technol. 2022;77:103890. doi:10.1016/j.jddst.2022.103890. [Google Scholar] [CrossRef]
63. Garcia MAVT, Garcia CF, Faraco AAG. Pharmaceutical and biomedical applications of native and modified starch: a review. Starch—Stärke. 2020;72:7–8. doi:10.1002/star.201900270. [Google Scholar] [CrossRef]
64. da Róz AL, Veiga-Santos P, Ferreira AM, Antunes TCR, de Leite FL, Yamaji FM, et al. Water susceptibility and mechanical properties of thermoplastic starch-pectin blends reactively extruded with edible citric acid. Mater Res. 2016;19:138–42. [Google Scholar]
65. Susheel K, Avérous L. Biodegradable and biobased polymers for environmental and biomedical applications. Wiley; 2016. [Google Scholar]
66. Sarder R, Piner E, Rios DC, Chacon L, Artner MA, Barrios N, et al. Copolymers of starch, a sustainable template for biomedical applications: a review. Carbohydr Polym. 2022;278:118973. doi:10.1016/j.carbpol.2021.118973 [Google Scholar] [PubMed] [CrossRef]
67. Charoensri K, Rodwihok C, Wongratanaphisan D, Ko JA, Chung JS, Park HJ. Investigation of functionalized surface charges of thermoplastic starch/zinc oxide nanocomposite films using polyaniline: tthe potential of improved antibacterial properties. Polymers. 2021;13(3):425. doi:10.3390/polym13030425 [Google Scholar] [PubMed] [CrossRef]
68. Diyana ZN, Jumaidin R, Selamat MZ, Ghazali I, Julmohammad N, Huda N, et al. Physical properties of thermoplastic starch derived from natural resources and its blends: a review. Polymers. 2021;13(9):1396. doi:10.3390/polym13091396 [Google Scholar] [PubMed] [CrossRef]
69. van Soest JJG, Benes K, de Wit D, Vliegenthart JFG. The influence of starch molecular mass on the properties of extruded thermoplastic starch. Polymer. 1996;37(16):3543–52. doi:10.1016/0032-3861(96)00165-6. [Google Scholar] [CrossRef]
70. Zhang Y, Rempel C, Liu Q. Thermoplastic starch processing and characteristics—a review. Crit Rev Food Sci Nutr. 2014;54(10):1353–70. doi:10.1080/10408398.2011.636156 [Google Scholar] [PubMed] [CrossRef]
71. Nossa TS, Belgacem NM, Gandini A, Carvalho AJ. Thermoreversible crosslinked thermoplastic starch. Polym Int. 2015;64(10):1366–72. doi:10.1002/pi.4925. [Google Scholar] [CrossRef]
72. Santos-Rosales V, Alvarez-Rivera G, Hillgärtner M, Cifuentes A, Itskov M, García-González CA, et al. Stability studies of starch aerogel formulations for biomedical applications. Biomacromol. 2020;21(12):5336–44. doi:10.1021/acs.biomac.0c01414 [Google Scholar] [PubMed] [CrossRef]
73. Mary SK, Koshy RR, Arunima R, Thomas S, Pothen LA. A review of recent advances in starch-based materials: bionanocomposites, pH sensitive films, aerogels and carbon dots. Carbohydr Pol Technol App. 2022;3:100190. doi:10.1016/j.carpta.2022.100190. [Google Scholar] [CrossRef]
74. El-Naggar ME, Othman SI, Allam AA, Morsy OM. Synthesis, drying process and medical application of polysaccharide-based aerogels. Int J Biol Macromol. 2020;145:1115–28. doi:10.1016/j.ijbiomac.2019.10.037 [Google Scholar] [PubMed] [CrossRef]
75. Moghadam M, Seyed Dorraji MS, Dodangeh F, Ashjari HR, Mousavi SN, Rasoulifard MH. Design of a new light curable starch-based hydrogel drug delivery system to improve the release rate of quercetin as a poorly water-soluble drug. Eur J Pharm Sci. 2022;174:106191. doi:10.1016/j.ejps.2022.106191 [Google Scholar] [PubMed] [CrossRef]
76. Gholamali I, Yadollahi M. Doxorubicin-loaded carboxymethyl cellulose/Starch/ZnO nanocomposite hydrogel beads as an anticancer drug carrier agent. Int J Biol Macromol. 2020;160:724–35. doi:10.1016/j.ijbiomac.2020.05.232 [Google Scholar] [PubMed] [CrossRef]
77. Nezami S, Sadeghi M, Mohajerani H. A novel pH-sensitive and magnetic starch-based nanocomposite hydrogel as a controlled drug delivery system for wound healing. Polym Degrad Stab. 2020;179:109255. Available from: https://linkinghub.elsevier.com/retrieve/pii/S0141391020301877 doi:10.1016/j.polymdegradstab.2020.109255. [Google Scholar] [CrossRef]
78. Movahedi M, Karbasi S. Electrospun halloysite nanotube loaded polyhydroxybutyrate-starch fibers for cartilage tissue engineering. Int J Biol Macromol. 2022;214:301–11. doi:10.1016/j.ijbiomac.2022.06.072 [Google Scholar] [PubMed] [CrossRef]
79. Movahedi M, Asefnejad A, Rafienia M, Khorasani MT. Potential of novel electrospun core-shell structured polyurethane/starch (hyaluronic acid) nanofibers for skin tissue engineering: in vitro and in vivo evaluation. Int J Biol Macromol. 2020;146:627–37. doi:10.1016/j.ijbiomac.2019.11.233 [Google Scholar] [PubMed] [CrossRef]
80. da Silva GLP, Morais LCDA, Olivato JB, Marini J, Ferrari PC. Antimicrobial dressing of silver sulfadiazine-loaded halloysite/cassava starch-based (bio)nanocomposites. J Biomater Appl. 2021;35(9):1096–108. doi:10.1177/0885328221995920 [Google Scholar] [PubMed] [CrossRef]
81. Mistry P, Chhabra R, Muke S, Narvekar A, Sathaye S, Jain R, et al. Fabrication and characterization of starch-TPU based nanofibers for wound healing applications. Mater Sci Eng C. 2021;119:111316. doi:10.1016/j.msec.2020.111316 [Google Scholar] [PubMed] [CrossRef]
82. Sethi S, Saruchi, Medha, Thakur S, Kaith BS, Sharma N, et al. Biopolymer starch-gelatin embedded with silver nanoparticle-based hydrogel composites for antibacterial application. Biomass Convers Biorefin. 2022;12:5363–84. doi:10.1007/s13399-022-02437-w. [Google Scholar] [CrossRef]
83. Queiroz VM, Kling ICS, Eltom AE, Archanjo BS, Prado M, Simão RA. Corn starch films as a long-term drug delivery system for chlorhexidine gluconate. Mater Sci Eng C. 2020;112:110852. doi:10.1016/j.msec.2020.110852 [Google Scholar] [PubMed] [CrossRef]
84. Culenova M, Birova I, Alexy P, Galfiova P, Nicodemou A, Moncmanova B, et al. In vitro characterization of poly(Lactic Acid)/Poly(Hydroxybutyrate)/thermoplastic starch blends for tissue engineering application. Cell Transplant. 2021;30:096368972110210. doi:10.1177/09636897211021003 [Google Scholar] [PubMed] [CrossRef]
85. Zhang L, Zheng T, Wu L, Han Q, Chen S, Kong Y, et al. Fabrication and characterization of 3D-printed gellan gum/starch composite scaffold for Schwann cells growth. Nanotechnol Rev. 2021;10(1):50–61. doi:10.1515/ntrev-2021-0004. [Google Scholar] [CrossRef]
86. Taherimehr M, Bagheri R, Taherimehr M. In-vitro evaluation of thermoplastic starch/beta-tricalcium phosphate nano-biocomposite in bone tissue engineering. Ceram Int. 2021;47(11):15458–63. doi:10.1016/j.ceramint.2021.02.111. [Google Scholar] [CrossRef]
87. Bou-Francis A, Piercey M, Al-Qatami O, Mazzanti G, Khattab R, Ghanem A. Polycaprolactone blends for fracture fixation in low load-bearing applications. J Appl Polym Sci. 2020;137(32):48940. doi:10.1002/app.48940. [Google Scholar] [CrossRef]
88. Herrera-Ibarra E, Salazar-Hernández M, Talavera-López A, Solis-Marcial OJ, Hernandez-Soto R, Ruelas-Leyva JP, et al. Preparation of surgical thread from a bioplastic based on nopal mucilage. Polymers. 2023;15(9):2112. doi:10.3390/polym15092112 [Google Scholar] [PubMed] [CrossRef]
89. Yadav M, Goswami P, Paritosh K, Kumar M, Pareek N, Vivekanand V. Seafood waste: a source for preparation of commercially employable chitin/chitosan materials. Bioresour Bioprocess. 2019;6:8. doi:10.1186/s40643-019-0243-y. [Google Scholar] [CrossRef]
90. Bukvic GD, Rossi E, Errea MI. Polysaccharides as economic and sustainable raw materials for the preparation of adsorbents for water treatment. Polysaccharides. 2023;4(3):219–55. doi:10.3390/polysaccharides4030016. [Google Scholar] [CrossRef]
91. Errea MI, Rossi E, Goyanes SN, D’Accorso NB. Chitosan: From organic pollutants to high-value polymeric materials. In: Industrial applications of renewable biomass products. Cham: Springer International Publishing; 2017. p. 251–64. doi:10.1007/978-3-319-61288-1_10. Available from: http://link.springer.com/10.1007/978-3-319-61288-1_10 [Accessed 2023]. [Google Scholar] [CrossRef]
92. Colobatiu L, Gavan A, Potarniche AV, Rus V, Diaconeasa Z, Mocan A, et al. Evaluation of bioactive compounds-loaded chitosan films as a novel and potential diabetic wound dressing material. React Funct Polym. 2019;145:104369. doi:10.1016/j.reactfunctpolym.2019.104369. [Google Scholar] [CrossRef]
93. Lu J, Chen Y, Ding M, Fan X, Hu J, Chen Y, et al. A 4arm-PEG macromolecule crosslinked chitosan hydrogels as antibacterial wound dressing. Carbohydr Polym. 2022;277:118871. Available from: https://linkinghub.elsevier.com/retrieve/pii/S0144861721012583 doi:10.1016/j.carbpol.2021.118871 [Google Scholar] [PubMed] [CrossRef]
94. Ranjith R, Balraj S, Ganesh J, John Milton MC. Therapeutic agents loaded chitosan-based nanofibrous mats as potential wound dressings: a review. Mater Today Chem. 2019;12:386–95. Available from: https://linkinghub.elsevier.com/retrieve/pii/S2468519418303033 doi:10.1016/j.mtchem.2019.03.008. [Google Scholar] [CrossRef]
95. Yang S, Zhang X, Zhang D. Electrospun chitosan/poly (vinyl alcohol)/graphene oxide nanofibrous membrane with ciprofloxacin antibiotic drug for potential wounddressing application. Int J Mol Sci. 2019;20:4395. doi:10.3390/ijms20184395 [Google Scholar] [PubMed] [CrossRef]
96. Hashemikia S, Farhangpazhouh F, Parsa M, Hasan M, Hassanzadeh A, Hamidi M. Fabrication of ciprofloxacin-loaded chitosan/polyethylene oxide/silica nanofibers for wound dressing application: in vitro and in vivo evaluations. Int J Pharm. 2021;597:120313. doi:10.1016/j.ijpharm.2021.120313 [Google Scholar] [PubMed] [CrossRef]
97. Qu J, Zhao X, Liang Y, Xu Y, Ma PX, Guo B. Degradable conductive injectable hydrogels as novel antibacterial, anti-oxidant wound dressings for wound healing. Chem Eng J. 2019;362:548–60. doi:10.1016/j.cej.2019.01.028. [Google Scholar] [CrossRef]
98. Bagheri M, Validi M, Gholipour A, Makvandi P, Sharifi E. Chitosan nanofiber biocomposites for potential wound healing applications: antioxidant activity with synergic antibacterial effect. Bioeng Transl Med. 2022;7(1):e10254. doi:10.1002/btm2.10254 [Google Scholar] [PubMed] [CrossRef]
99. Deng L, Wang B, Li W, Han Z, Chen S, Wang H. Bacterial cellulose reinforced chitosan-based hydrogel with highly efficient self-healing and enhanced antibacterial activity for wound healing. Int J Biol Macromol. 2022;217:77–87. doi:10.1016/j.ijbiomac.2022.07.017 [Google Scholar] [PubMed] [CrossRef]
100. Massarelli E, Silva D, Pimenta AFR, Fernandes AI, Mata JLG, Armês H, et al. Polyvinyl alcohol/chitosan wound dressings loaded with antiseptics. Int J Pharm. 2021;593:120110. doi:10.1016/j.ijpharm.2020.120110 [Google Scholar] [PubMed] [CrossRef]
101. Chen Z, Yao J, Zhao J, Wang S. Injectable wound dressing based on carboxymethyl chitosan triple-network hydrogel for effective wound antibacterial and hemostasis. Int J Biol Macromol. 2023;225:1235–45. doi:10.1016/j.ijbiomac.2022.11.184 [Google Scholar] [PubMed] [CrossRef]
102. Mahanta AK, Maiti P. Injectable hydrogel through hydrophobic grafting on chitosan for controlled drug delivery. ACS Appl Bio Mater. 2019;2(12):5415–26. doi:10.1021/acsabm.9b00733 [Google Scholar] [PubMed] [CrossRef]
103. Belabassi Y, Moreau J, Gheran V, Henoumont C, Robert A, Callewaert M, et al. Synthesis and characterization of PEGylated and fluorinated chitosans: application to the synthesis of targeted nanoparticles for drug delivery. Biomacromol. 2017;18(9):2756–66. doi:10.1021/acs.biomac.7b00668 [Google Scholar] [PubMed] [CrossRef]
104. Soerjomataram I, Bray F. Planning for tomorrow: global cancer incidence and the role of prevention 2020-2070. Nat Rev Clin Oncol. 2021;18:663–72. doi:10.1038/s41571-021-00514-z [Google Scholar] [PubMed] [CrossRef]
105. Zhang E, Xing R, Liu S, Qin Y, Li K, Li P. Advances in chitosan-based nanoparticles for oncotherapy. Carbohydr Polym. 2019;222:115004. doi:10.1016/j.carbpol.2019.115004 [Google Scholar] [PubMed] [CrossRef]
106. Ding J, Guo Y. Recent advances in chitosan and its derivatives in cancer treatment. Front Pharmacol. 2022;13:1–13. doi:10.3389/fphar.2022.888740 [Google Scholar] [PubMed] [CrossRef]
107. Song X, Chen Y, Zhao G, Sun H, Che H, Leng X. Effect of molecular weight of chitosan and its oligosaccharides on antitumor activities of chitosan-selenium nanoparticles. Carbohydr Polym. 2020;231:115689. doi:10.1016/j.carbpol.2019.115689 [Google Scholar] [PubMed] [CrossRef]
108. Manjusha V, Rajeev MR, Anirudhan TS. Magnetic nanoparticle embedded chitosan-based polymeric network for the hydrophobic drug delivery of paclitaxel. Int J Biol Macromol. 2023;235:123900. doi:10.1016/j.ijbiomac.2023.123900 [Google Scholar] [PubMed] [CrossRef]
109. Kolawole OM, Ifeanafor AR, Ifade WA, Akinleye MO, Patrojanasophon P, Silva BO, et al. Formulation and evaluation of paclitaxel-loaded boronated chitosan/alginate nanoparticles as a mucoadhesive system for localized cervical cancer drug delivery. J Drug Deliv Sci Technol. 2023;87:104810. doi:10.1016/j.jddst.2023.104810 [Google Scholar] [PubMed] [CrossRef]
110. El-Alfy EA, El-Bisi MK, Taha GM, Ibrahim HM. Preparation of biocompatible chitosan nanoparticles loaded by tetracycline, gentamycin and ciprofloxacin as novel drug delivery system for improvement the antibacterial properties of cellulose based fabrics. Int J Biol Macromol. 2020;161:1247–60. doi:10.1016/j.ijbiomac.2020.06.118 [Google Scholar] [PubMed] [CrossRef]
111. Gao G, Jiang Y-W, Jia H-R, Wu F-G. Near-infrared light-controllable on-demand antibiotics release using thermo-sensitive hydrogel-based drug reservoir for combating bacterial infection. Biomater. 2019;188:83–95. doi:10.1016/j.biomaterials.2018.09.045 [Google Scholar] [PubMed] [CrossRef]
112. Chai Y, Wang Y, Li B, Qi W, Su R, He Z. Microfluidic synthesis of Lignin/Chitosan nanoparticles for the pH-responsive delivery of anticancer drugs. Langmuir. 2021;37(23):7219–26. doi:10.1021/acs.langmuir.1c00778 [Google Scholar] [PubMed] [CrossRef]
113. Long J, Etxeberria AE, Kornelsen C, Nand AV, Ray S, Bunt CR, et al. Development of a long-term drug delivery system with levonorgestrel-loaded chitosan microspheres embedded in poly(vinyl alcohol) hydrogel. ACS Appl Bio Mater. 2019;2(7):2766–79. doi:10.1021/acsabm.9b00190 [Google Scholar] [PubMed] [CrossRef]
114. Sung HW, Chen MC, Lin YH, Liang HF, Tu H. Nanoparticles for protein drug delivery. Patent: US7541046B1. USA; 2008. Available from: https://patents.google.com/patent/US7541046B1/ [Accessed 2023]. [Google Scholar]
115. Liu YL, Wang WY, Dong WJ, Hao HZ, Xia XJ, Huang YS, et al. Phospholipid chitosan drug delivery system and preparation method and application thereof. Patent: CN107308132B. China; 2016. Available from: https://patents.google.com/patent/CN107308132B/en [Accessed 2023]. [Google Scholar]
116. Liu Z, Chen Q, Jin Q, Zhao Q, Xiao Z, Wei T, et al. Use of fluorine-containing compound-modified cationic polymer as drug carrier and preparation method. Patent: US20220273806A1. USA; 2020. Available from: https://patents.google.com/patent/US20220273806A1/ [Accessed 2023]. [Google Scholar]
117. Huang L, Jia J, Lai G, Li Z, Liu K, Wen C, et al. Chitosan drug delivery system and preparation method and application thereof. Patent: AU2021100577A4. Australia; 2021. Available from: https://patents.google.com/patent/AU2021100577A4/). [Google Scholar]
118. Wedmore I, McManus JG, Pusateri AE, Holcomb JB. A special report on the chitosan-based hemostatic dressing: experience in current combat operations. J Trauma Acute Care Surg. 2006;60(3):655–8. doi:10.1097/01.ta.0000199392.91772.44 [Google Scholar] [PubMed] [CrossRef]
119. Brown MA, Daya MR, Worley JA. Experience with Chitosan dressings in a civilian EMS system. J Emerg Med. 2009;37(1):1–7. doi:10.1016/j.jemermed.2007.05.043 [Google Scholar] [PubMed] [CrossRef]
120. Oliveira RF, Sousdaleff M, Lima MVS, Lima HOS. Produção fermentativa de ácido lático a partir do melaço da cana-de-açúcar por Lactobacillus casei. Braz J Food Technol. 2009;VII:34–40. [Google Scholar]
121. Lunt J. Large-scale production, properties and commercial applications of polylactic acid polymers. Polym Degrad Stab. 1998;59(1–3):145–52. [Google Scholar]
122. Lasprilla AJR, Martinez GAR, Lunelli BH, Jardini AL, Filho RM. Poly-lactic acid synthesis for application in biomedical devices—a review. Biotechnol Adv. 2012;30(1):321–8 [Google Scholar] [PubMed]
123. Narayanan N, Roychoudhury PK, Srivastava A. L (+) lactic acid fermentation and its product polymerization. Electron J Biotechnol. 2004;7(2):167–79. [Google Scholar]
124. Napoothiri MK, Nair NR, John RP. An overview of the recent developments in polylactide (PLA) research. Bioresour Technol. 2010;101(22):8493–501. [Google Scholar]
125. Vert M, Feijen J, Albertsson A, Scott G, Chiellini E. Biodegradable polymers/plastics. Cambridge, UK: Royal Society of Chemistry; 1992. [Google Scholar]
126. Auras RH, Lim LT, Selke SEM, Tsuji H. Poly(lactic acidsynthesis, structures, properties, processing, and applications. Wiley; 2010. [Google Scholar]
127. Tsuji H, Ikada Y. Crystallization from the melt of poly(lactide)s with different optical purities and their blends. Macromol Chem Phys. 1996;197(10):3483–99. [Google Scholar]
128. Avinc O, Khoddami A. Overview of poly(lactic acid) (PLA) fibre. Fibre Chem. 2009;41:391–401. [Google Scholar]
129. Lopes SM, Jardini A, Filho MR. Synthesis and characterizations of poly(lactic acid) by ring-opening polymerization for biomedical applications. Chem Eng Trans. 2014;38:331–6. [Google Scholar]
130. Queiroz PD. Diagrama de fases, propriedades termicas e morfologicas de blendas de poli(acido lactico) e poli(metacrilato de metila) (Ph.D. Thesis). State University of Campinas Institute of Chemistry: Brazil; 2000 (In Portuguese). [Google Scholar]
131. Gupta B, Revagade N, Hilborn J. Poly(lactic acid) fiber: an overview. Prog Polym Sci. 2007;32(4):455–82. [Google Scholar]
132. Cheng Y, Deng S, Chen P, Ruan R. Polylactic acid (PLA) synthesis and modifications: a review. Front Chem China. 2009;4:259–64. [Google Scholar]
133. Rathi S, Coughlin E, Hsu S, Golub C, Ling G, Tzivanis M. Maintaining structural stability of poly(lactic acideffects of multifunctional epoxy based reactive oligomers. Polymers. 2014;6(4):1232–50. [Google Scholar]
134. Sharma SJ. Handbook of applied biopolymer technology: synthesis, degradation and applications. In: RSC green chemistry series: royal society of chemistry. Cambridge, UK: The Royal Society of Chemistry; 2011. [Google Scholar]
135. Barbanti SH, Zavaglia CAC, Duek EAR. Bioresorbable polymers in tissue engineering. Polym. 2005;15:13–21. [Google Scholar]
136. Pawar PR, Tekale US, Shisodia US, Totre TJ, Domb JA. Biomedical applications of poly(lactic acid). Recent Pat Regen Med. 2014;4(1):40–51. [Google Scholar]
137. DeStefano V, Khan S, Tabada A. Applications of PLA in modern medicine. Eng Regen. 2020;1:76–87 [Google Scholar] [PubMed]
138. Tyler B, Gullotti D, Mangraviti A, Utsuki T, Brem H. Polylactic acid (PLA) controlled delivery carriers for biomedical applications. Adv Drug Deliv Rev. 2016;107:163–75 [Google Scholar] [PubMed]
139. Singhvi MS, Zinjarde SS, Gokhale DV. Polylactic acid: synthesis and biomedical applications. J Appl Microbiol. 2019;127(6):1612–26 [Google Scholar] [PubMed]
140. Li G, Zhao M, Xu F, Yang B, Li X, Meng X, et al. Synthesis and biological application of polylactic acid. Molecules. 2020;25(21):5023 [Google Scholar] [PubMed]
141. Ebrahimi F, Ramezani Dana H. Poly lactic acid (PLA) polymers: from properties to biomedical applications. Intern J Polym Mater Polym Biomater. 2022;71(15):1117–30. [Google Scholar]
142. Buj-Corral I, Sanz-Fraile H, Ulldemolins A, Tejo-Otero A, Domínguez-Fernández A, Almendros I, et al. Characterization of 3D printed metal-PLA composite scaffolds for biomedical applications. Polymers. 2022;14(13):2754 [Google Scholar] [PubMed]
143. Forrestal B, Case BC, Yerasi C, Musallam A, Chezer-Azerrad C, Waksman R. Bioresorbable scaffolds: ccurrent technology and future perspectives. Rambam Maimonides Med J. 2020;11(2):e0016 [Google Scholar] [PubMed]
144. Johnson&Johson MedTech. MONOCRYL (poliglecaprone 25) suture. Available from: https://www.jnjmedtech.com/en-EMEA/product/monocryl-poliglecaprone-25-suture. [Accessed 2024]. [Google Scholar]
145. Medtronic. V-LocTM wound closure device—barbed sutures. Available from: https://www.medtronic.com/covidien/en-us/products/wound-closure/barbed-sutures.html. [Accessed 2024]. [Google Scholar]
146. Toong DWY, Toh HW, Ng JCK, Wong PEH, Leo HL, Venkatraman S, et al. Bioresorbable polymeric scaffold in cardiovascular applications. Int J Mol Sci. 2020;21(10):3444 [Google Scholar] [PubMed]
147. FANTOM ENCORE. Bioresorbable scaffold. Available from: https://www.revamedical.com/why-bioresorbable-seaffolds/fantom-encore. [Accessed 2024]. [Google Scholar]
148. NatureWorks. 3D printing. Available from: https://www.natureworksllc.com/applications/3d-printing. [Accessed 2024]. [Google Scholar]
149. Stryker. SonicAnchor bioresorbable anchoring system. Available from: https://www.stryker.com/us/en/foot-and-ankle/products/sonicanchor.html. [Accessed 2024]. [Google Scholar]
150. GORE. GORE® BIO-A® tissue reinforcement. Available from: https://www.goremedical.com/products/bioatissue#:~:text=GORE%C2%AE%20BIO%2DA%C2%AE%20Tissue%20Reinforcement%20is%20a%20leading,gradually%20absorbed%20by%20the%20body. [Accessed 2024]. [Google Scholar]
151. XPRT medical. TephaFLEX—absorbable monofilament suture. Available from: https://www.medical-xprt.com/products/tephaflex-absorbable-monofilament-suture-770465. [Accessed 2024]. [Google Scholar]
152. KLS martin group. Implants and implant systems dental. Available from: https://www.klsmartin.com/en/products/implants-dental. [Accessed 2024]. [Google Scholar]
153. KLS martin group. Resorbable-osteosynthesis. Available from: https://www.klsmartin.com/en/products/implants-cmf/resorbable-osteosynthesis. [Accessed 2024]. [Google Scholar]
Cite This Article
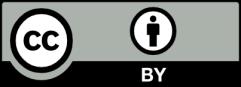
This work is licensed under a Creative Commons Attribution 4.0 International License , which permits unrestricted use, distribution, and reproduction in any medium, provided the original work is properly cited.