Open Access
ARTICLE
Morphological Evolution of Self-Assembled Sodium Dodecyl Sulfate/Dodecyltrimethylammonium Bromide@Epoxy-β-Cyclodextrin Supramolecular Aggregates Induced by Temperature
1 Key Laboratory of Textile Science & Technology, Ministry of Education, College of Textiles, Donghua University, Shanghai, 201620, China
2 Collaborative Innovation Center of Fragrance Flavor and Cosmetics, School of Perfume and Aroma Technology (Shanghai Research Institute of Fragrance & Flavour Industry), Shanghai Institute of Technology, Shanghai, China
* Corresponding Authors: Qinfei Ke. Email: ; Xingran Kou. Email:
(This article belongs to the Special Issue: Application of Renewable Materials in Perfumes, Fragrances, and Cosmetics)
Journal of Renewable Materials 2024, 12(4), 629-641. https://doi.org/10.32604/jrm.2023.029182
Received 03 February 2023; Accepted 24 March 2023; Issue published 12 June 2024
Abstract
Bio-based cyclodextrins (CDs) are a common research object in supramolecular chemistry. The special cavity structure of CDs can form supramolecular self-assemblies such as vesicles and microcrystals through weak interaction with guest molecules. The different forms of supramolecular self-assemblies can be transformed into each other under certain conditions. The regulation of supramolecular self-assembly is not only helpful to understand the self-assembly principle, but also beneficial to its application. In the present study, the self-assembly behavior of epoxy-β-cyclodextrin (EP-β-CD) and mixed anionic and cationic surfactant system (sodium dodecyl sulfate/dodecyltrimethylammonium bromide, SDS/DTAB) in aqueous solution was studied. Morphological and particle size characterization found that the SDS/DTAB@EP-β-CD complex, as the basic building unit, self-assembled into worm-like micelles at lower temperatures and vesicles at higher temperatures. Nuclear magnetic resonance (NMR) and Fourier transform infrared spectroscopy (FT-IR) analysis revealed that the driving force for the formation of vesicles and worm-like micelles was the hydrogen bonds between EP-β-CD molecules, while water molecules played an important role in promoting vesicle formation between SDS/DTAB@EP-β-CD units. Herein, the mechanism of the morphologic transformation of SDS/DTAB@EP-β-CD supramolecular aggregates induced by temperature was elucidated by exploring the self-assembly process, which may provide an excellent basis for the development of delivery carriers.Graphic Abstract
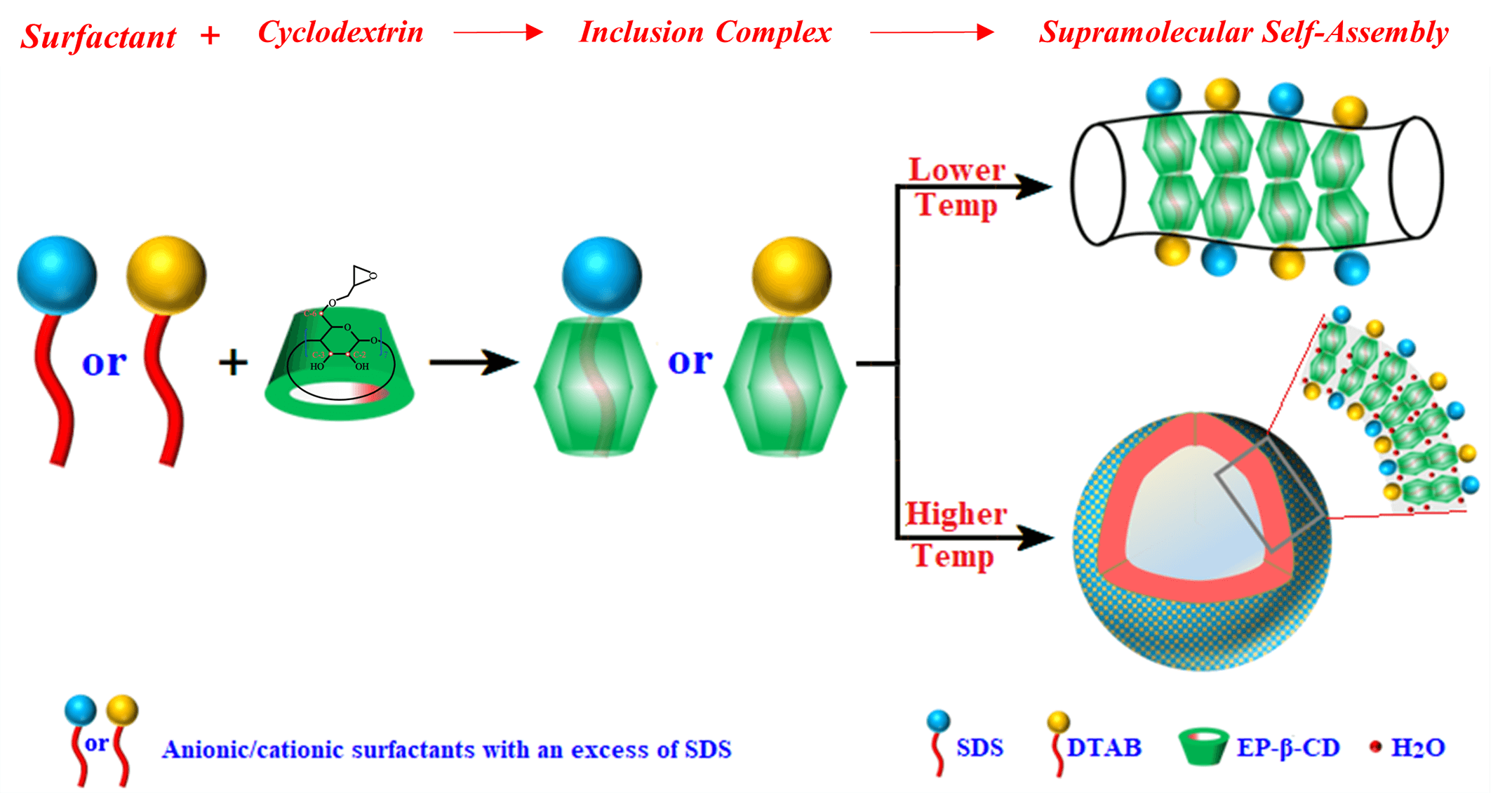
Keywords
Nomenclature
EP-β-CD | Epoxy-β-cyclodextrin |
SDS/DTAB | Sodium dodecyl sulfate/dodecyltrimethylammonium bromide |
CDs | Cyclodextrins |
TDPS | Tetradecyldimethylpropane sulfonate |
NMR | Nuclear magnetic resonance |
FT-IR | Fourier transform infrared spectroscopy |
TEM | Transmission electron microscopy |
DLS | Dynamic light scattering |
TG | Thermogravimetric analysis |
DSC | Differential scanning calorimetry |
UV-vis | Ultraviolet visible |
Dh | Hydrodynamic diameter |
Cyclodextrins (CDs) are a kind of bio-based raw material, which are mainly produced by starch, glycogen, malt oligosaccharide, and other green regenerative raw materials under the action of cyclodextringlycosyltransferase. Common commercial CDs are a kind of circular oligosaccharides with conical cavity structure formed by 6–8 glucose units connected by α-1,4-glycoside bonds, named α-CD, β-CD, and γ-CD [1]. The inner conical cavity of CDs is hydrophobic, while the outer cavity wall is hydrophilic, and this special structure enables CDs to form inclusion complexes with surfactants through host-guest inclusion coordination, hydrophobic interaction, van der Waals force, etc. In recent years, CDs have been widely used in the construction and regulation of aggregates and have become one of the most important objects in the field of supramolecular research [2–4]. Among the three common CDs, β-CD possesses a larger cavity diameter than α-CD and a lower production cost than γ-CD, and this makes β-CD a research hotspot in the supramolecular aggregates field [5]. However, β-CD itself has many limitations in solubility, safety, embedding, binding ability, etc. Therefore, it is necessary to modify β-CD chemically to improve its physical and chemical properties. Chemical modification can increase the water solubility of β-CD, change the binding cavity or provide other specific binding sites, introduce special groups to build particular supramolecules, assist in the construction of special stereo geometric relationships, etc., thereby improving its practical application range. At present, the introduction of functional groups from the primary surface of CDs (the narrow end of the tapered cavity) is a relatively mature means for β-CD modification, and the main β-CD derivatives include ionic β-CD (thio-β-CD [6], carboxymethyl-β-CD [7]), hydrophobic β-CD (diethyl-β-CD [8], hydroxyethyl-β-CD [9]), hydrophilic β-CD [10], etc.
Amphiphilic molecules, especially surfactant molecules, have both hydrophilic and hydrophobic groups and can self-assemble into ordered structures in the aqueous environment [11,12]. If the hydrophobic part of the surfactant matches the size of the CD cavity, it will enter the cavity and form the inclusion complexes [13], which can further be used as basic building units to construct various higher-order structures (such as sheets, vesicles, nanotubes, nanofibers, etc.) [14]. The binding strength of the self-assembled system depended on the structure of the surfactant and the ratio to CDs, while the crystalline assembly depended on the driving forces between surfactants and CDs, which mainly included hydrogen bonds and other interactions (such as electrostatic interactions between charged groups on different types of surfactants) [15,16]. Jiang et al. found that the hydrophobic alkyl chain of sodium dodecyl sulfate (SDS) could easily inserted into the cavity of β-CD to form SDS@2β-CD dimer, and such dimers were able to self-assembled as basic building units into channel-type crystalline bilayers. The formed crystalline bilayers could expand and extend to form an infinite two-dimensional layered structure at high concentration, and such an infinite two-dimensional layered structure could expand along the axis to form a one-dimensional multilayer microtubes or dispersed vesicle system by dilution [17,18]. Similarly, tetradecyldimethylpropane sulfonate (TDPS) was able to form a 2TDPS@3β-CD structural unit, which could further self-assembled into microtubes and other structures along a certain direction [19]. Compared with SDS@2β-CD nanotubes, TDPS@3β-CD microtubules were more flexible, mainly because the head group of TDPS was a zwitterion with less electrostatic repulsion while SDS was an anion with greater electrostatic repulsion. In addition to ionic surfactants, nonionic surfactants such as Tween 20 could also self-assemble with CDs to form various ordered structures [20,21]. Surfactants with special topologies (such as gemini/bola type) were also able to self-assemble with CDs [22,23].
Vesicles are supramolecules formed by the self-assembly of amphiphilic molecules. Since the vesicle system can improve the solubility of hydrophilic, lipophilic, and organic macromolecules at the same time, it can be used to prepare and encapsulate multiple active ingredients at the same time [24]. Li et al. prepared self-assembled SDS/β-CD vesicles with a drug loading capacity of 1.47% and an encapsulation rate of 87.62% [25].
The morphology evolution of supramolecular can occur between various self-assembled aggregates such as micelles, sheets, and vesicles. So far, many studies have focused on the morphological transition between micelles and vesicles, because the inter-transformation process between micelles and vesicles is fundamentally important for basic applied research. Many simple control methods (such as enzymes [26], pH [27], light [28], concentration [29], and temperature [30], etc.) can be used to modulate CDs-guest supramolecular self-assembly. Among the above control means, temperature regulation can greatly affect the non-covalent interactions between aggregates, thereby ultimately controlling the self-assembled morphology of molecules [31].
In the present study, the self-assembly behavior of epoxy-β-cyclodextrin (EP-β-CD) and mixed anionic and cationic surfactant system (sodium dodecyl sulfate/dodecyltrimethylammonium bromide, SDS/DTAB) in aqueous solution was studied. The effects of temperature on the transformation of SDS/DTAB@EP-β-CD supramolecular aggregates were analyzed by macroscopic observation, turbidity and viscosity analysis, and particle size distribution analysis. The morphological changes of SDS/DTAB@EP-β-CD supramolecules were characterized by means of nuclear magnetic resonance (NMR), Fourier transform infrared spectroscopy (FT-IR), thermodynamic analysis, transmission electron microscopy (TEM), etc., so as to elucidate the possible mechanism. Herein, the mechanism of the morphologic transformation of SDS/DTAB@EP-β-CD supramolecular aggregates induced by temperature was elucidated by exploring the self-assembly process, which may provide an excellent basis for the development of delivery carriers.
SDS of analytical grade was purchased from Shanghai Adamas Reagent Co., Ltd. (Shanghai, China). DTAB of reagent grade was purchased from Beyotime Biotechnology Co., Ltd. (Shanghai, China). Phosphotungstic acid of high purity grade was purchased from VWR (Shanghai) Co., Ltd. (China). EP-β-cyclodextrin (EP-β-CD) (≥98%) was purchased from Shandong Binzhou Zhiyuan Biotechnology Co., Ltd. (China).
2.2 Preparation of Supramolecular Self-Assembled Aggregates
A mixed surfactant solution of SDS and DTAB (molar ratio of 3:1, Ctotal = 10 mmol/L) was accurately weighed and placed in a glass bottle. Subsequently, 16 mmol/L EP-β-CD aqueous solution was added to the above mixed surfactant system by drops at a host-guest ratio of 2:1. The mixture was thoroughly mixed to ensure a uniform dispersion of EP-β-CD. Finally, the obtained mixture was placed in incubators at 15°C, 25°C, and 35°C for 24 h to achieve equilibrium.
2.3 Characterization of Supramolecular Self-Assembled Aggregates
2.3.1 Dynamic Light Scattering (DLS) Analysis
A DLS particle size and zeta potential analyzer (NanoBrook Omni, Brookhaven Instruments, USA) equipped with a 35-mW optical pump semiconductor laser was used to characterize the supramolecular self-assembly system formed at different temperatures (15°C, 25°C, and 35°C). The scattering angle was fixed to 90°. The samples were filtered with 0.45 μm microporous membrane before testing.
2.3.2 Absorbance and Viscosity Analysis
An ultraviolet visible (UV-vis) spectrophotometer (UV-1800, Shimadzu Analytical Technology Co., Ltd., Japan) with resolution of 1 nm was used to measure the turbidity of the mixed solution at different temperatures (5°C–40°C). The absorbance was monitored at the wavelength of 500 nm, and there is no absorption for the blank mixed surfactant system under such wavelength.
A NDJ-5S digital viscometer (Shanghai Jingtian Electronics Instrument Co., Ltd., China) was used to measure the viscosity of the solution formed at different temperatures (5°C–40°C) with distilled water as the reference.
2.3.3 Transmission Electron Microscopy (TEM) Analysis
The morphology of supramolecular self-assembly system formed at different temperatures (15°C, 25°C, and 35°C) was observed by a TEM (Tecnai G2 20 TWIN, FEI Company, USA). Specifically, 2 drops of sample solution were first added to a 230-mesh copper grid coated with Formvar film, and 1 drop of phosphotungstate acid solution (0.2%) was added after removing excess liquid with filter paper. The samples were then left to dry at room temperature (25°C) before analysis.
2.3.4 Thermogravimetric Analysis (TG) and Differential Scanning Calorimetry (DSC) Analysis
TGA and DSC measurements were performed on the thermogravimetric analyzer (Q5000IR, TA Instruments, Shanghai, China) and differential scanning calorimeter (Q2000, TA Instruments, Shanghai, China), respectively. Before characterization, the prepared samples were frozen at −18°C for 24 h, and then lyophilized using a FD-1A-50 freeze dryer (Shanghai Bilon Instrument Manufacturing Co., Ltd., China) for at least 24 h. For DSC analysis, the lyophilized samples were placed in an alumina crucible and heated from 25°C to 120°C at a rate of 2°C/min under a nitrogen environment.
2.3.5 Fourier Transform Infrared Spectroscopy (FT-IR) Analysis
A Nicolet iN10 spectrometer (Nicolet iN10, Thermo Fisher Scientific, USA) was used to analyze the dried samples. All spectra were recorded in the range of 4000–400 cm−1 with the resolution of 4 cm−1.
2.3.6 Nuclear Magnetic Resonance (1H NMR) Analysis
The 1H NMR measurement was carried out with D2O as the deuterated reagent using an NMR apparatus (400 M, JEOL, Ltd., Japan) operating at 400 MHz. The displacements and changes of EP-β-CD, mixed surfactants, and supramolecular self-assemblies formed at different temperature (15°C, 25°C, and 35°C) were tested.
3.1 Aggregates Transition Induced by Different Temperature
By macroscopic observation, it was found that the SDS/DTAB aqueous solution containing 16 mmol/L EP-β-CD (Ctotal = 10 mM) was transparent at 25°C. However, the solution turned into transparent blue light after being kept at 15°C for 24 h (Fig. 1A), indicating that the system produced aggregates. When heated to 25°C and 35°C, the system transformed from a clear blue solution to a light blue cloudy solution, indicating that the aggregates transformed into larger particles.
Figure 1: Aqueous solutions of SDS/DTAB (Ctotal = 10 mM) containing 16 mM EP-β-CD were incubated for 24 h at three different temperatures. (A) Photographic images after incubating at 15°C (blue transparent solution), 25°C (slightly turbid solution), and 35°C (slightly blue turbid solution). (B) Changes in relative viscosity and absorbance of samples with different temperatures
When the temperature was increased from 5°C to 45°C, the turbidity change of the mixed solution could be easily observed by UV-vis spectroscopy. As shown in Fig. 1B (blue line), the absorbance of the mixture varies with temperature: the turbidity gradually increased with the increase of temperature, which was consistent with the macroscopic observations. The study of viscosity analysis provided more information about the transition of aggregates. At lower temperature, the SDS/DTAB@EP-β-CD solution was transparent (Fig. 1B, red line) with lower viscosity, which indicated that the major aggregates in the system were micelles. As the temperature increased, the viscosity increased and the solution appeared light blue, indicating the presence of large particles or vesicles in the system. Viscosity increase and permeability decrease are typical features of aggregate transformation [32,33]. Therefore, based on the above observations and measurements, it can be speculated that the solution may have transitioned from micelles to vesicles.
3.2 Temperature-Induced Supermolecules Morphology Transition
3.2.1 Aggregates Size Distribution and TEM Analysis
As shown in Fig. 2, the hydrodynamic diameter (Dh) of the aggregates produced at 15°C was 113.39 ± 2.97 nm (Fig. 2A). When the temperature increased to 25°C, the particle size of the self-assembled aggregates increased to 683.42 ± 3.11 nm (Fig. 2B). While at 35°C, the system transformed into larger aggregates with the particle size of 709.70 ± 4.87 nm (Fig. 2C).
Figure 2: DLS analysis of SDS/DTAB@EP-β-CD self-assemblies formed at 15°C (A), 25°C (B), and 35°C (C) and their autocorrelation curves (D)
Since the mixed SDS/DTAB surfactants (15 and 5 mmol/L) itself can form micelles in aqueous solution [34], while the size of micelles is typically within 100 nm (5–100 nm) [35]. Hence, other aggregates than micelles may exist in the SDS/DTAB@EP-β-CD system. Jiang et al (2011) reported the existence of hollow vesicles with diameters of about 100 and 700 nm SDS@2β-CD system [17]. Therefore, it is reasonable to speculate that the system in this study formed a high-sized polydisperse vesicle structure. To verify such speculation, TEM was used to characterize the aggregates present in different solutions.
As shown in Fig. 3A, typical worm-like micelles were formed at 15°C in the SDS/DTAB@EP-β-CD solution system. The increase in temperature resulted in a significant change in the structure of the aggregates. As shown in Figs. 3B and 3C, these aggregates exhibited different brightness at the edge and center, indicating that the aggregates were not solid particles but hollow vesicles. In addition, the forming process of aggregates can further verify the formation of vesicles. The red arrows in Fig. 3C indicate the rupture of some aggregates. In addition, it can also be observed from TEM images that some aggregates contain dark objects. According to Nayak et al., these dark objects may be small aggregates that were incorporated in vesicles during their formation [36].
Figure 3: Typical TEM images of self-assembled SDS/DTAB@EP-β-CD formed at 15°C (A), 25°C (B), and 35°C (C)
By comparing the autocorrelation curves of the slow decay curves of large particles, information about aggregate particle size can be characterized. It is well known that higher temperatures result in slower autocorrelation decay curves [37]. As shown in Fig. 2D, the higher the temperature, the larger the particle size of the system. To quantify the dimensional changes, the CONTIN method was used to convert the autocorrelation curves to the distribution of hydrodynamic radii [32]. DLS provides statistical results of particle size distribution, while TEM reflects the local morphology and size results of aggregates, and the results obtained by the two methods were consistent with each other. Therefore, with the change in temperature, the SDS/DTAB@EP-β-CD aggregates generated in the system changed from micelles to vesicles.
To reveal the steric changes in the EP-β-CD cavity due to the insertion of the alkyl chain of the guest molecule (surfactant), the 1H NMR characterization was carried out to confirm the formation of an inclusion between the mixed surfactant and EP-β-CD. As shown in Fig. 4A, the 1H NMR spectrum of EP-β-CD in D2O showed a triplet at δH3 = 3.85 ppm, which is denoted as the H3 proton. These protons were located in the wide end of the tapered cavity of the cyclodextrin cavity and had poor shielding properties. In contrast, the H5 protons were located inside the cavity and overlapped with the H6 protons located outside the cavity at the narrow end of the tapered cavity, yielding resonances of 3.75 and 3.77 ppm, respectively [36]. The H3 and H5 protons of β-CD molecules located in the cavity revealed the changes in the environment around the cavity caused by the entry of guest molecules, thus providing strong evidence for inclusion complex binding [38–40]. In the presence of the mixed SDS/DTAB surfactants, high-field chemical shift signals of the H3 and H5, which are located on the wide and narrow sides of the cavity, were observed. Such a chemical shift may be attributed to the formation of inclusion complexes that changed the conformation and electromagnetic environment of EP-β-CD and mixed surfactant SDS/DTAB molecules. When the system did not contain guest molecules, the EP-β-CD cavity was full of high-energy water, but when the mixed SDS/DTAB surfactants were added to the system, the hydrophobic cavity was replaced by guest molecules with suitable size. Therefore, the inclusion of non-polar species in the hydrophobic cavity shielded the internal protons of the EP-β-CD glucose unit [23]. In addition, the Δδ value of H6 was larger than that of H1, indicating that the alkane chain of the guest molecule was most likely inserted into the cavity of EP-β-CD from the main surface. Besides, these protons mainly interacted with EP-β-CD through the hydrophobic chain of mixed SDS/DTAB surfactants and then participated in the host-guest interaction.
Figure 4: 1H NMR spectra of EP-β-CD, SDS/DTAB, and self-assembled SDS/DTAB@EP-β-CD formed at 15°C, 25°C, and 35°C (A); (B) for the enlarged 1H NMR spectra of the H2, H4, H3, H5, and H6 protons of EP-β-CD; (C) for the enlarged 1H NMR spectra of the methyl protons in SDS/DTAB and the SDS/DTAB@EP-β-CD formed at 15°C, 25°C, and 35°C
Figs. 4B and 4C show an enlarged view of the local 1H NMR spectra of the H2, H4, H3, H5, and H6 protons of EP-β-CD, and the changes in chemical shifts (Δδ) are summarized in Table 1. When EP-β-CD was added to the mixed SDS/DTAB surfactants solution, the proton signals of the long alkyl chains of mixed SDS/DTAB surfactants shifted downfield from their initial values of 1.21 and 0.79 ppm, which indicated that the proton environment changes due to the inner cavity of EP-β-CD accommodated the alkyl side chain of the SDS/DTAB surfactants [41].
Studies have shown that hydrogen bonding played a crucial role in the self-assembly of CD-surfactant systems. Therefore, the characterization of SDS/DTAB@EP-β-CD prepared by EP-β-CD at different temperatures (15°C, 25°C, and 35°C) by FT-IR can further confirm the existence of hydrogen bonds [42]. It is well known that the association of OH groups resulted in a shift of the stretch peak [43]. In present study, as shown in Fig. 5, the hydroxyl group signal of the SDS/DTAB@EP-β-CD system became wider and stronger at 25°C and 35°C, which indicated that the hydrogen bond between EP-β-CD was strengthened with increasing of temperature [40], and the order of hydrogen bonding enhancement was 15°C << 25°C < 35°C.
Figure 5: FT-IR spectra of the EP-β-CD and self-assembled SDS/DTAB@EP-β-CD formed at 15°C, 25°C, and 35°C
According to the above results, the temperature dependence of aggregate morphology can be attributed to the effect of hydrogen bonds within the system. To further confirm such hypothesis, DSC and TGA experiments were performed. As shown in Fig. 6, the elimination of all water molecules was observed in the endothermic peaks shown by the DSC curves of SDS/DTAB@EP-β-CD vesicles prepared at 25°C and 35°C. The endothermic peak of the SDS/DTAB@EP-β-CD sample prepared at 25°C was about 58.04°C (Fig. 6A). When the preparation temperature increased to 35°C, the endothermic peak increased to 64.01°C (Fig. 6B), which may be due to the presence of tighter vesicles and abundant hydrogen bonds in the SDS/DTAB@EP-β-CD system at 35°C, and such compact structure and hydrogen bonds required additional energy to be destroyed, therefore the endothermic peak increased. The above DSC results were consistent with the change in hydrogen bond strength determined by FT-IR.
Figure 6: TGA and DSC results for the self-assembled SDS/DTAB@EP-β-CD vesicles formed at 25°C (A) and 35°C (B)
In contrast, the TGA results of lyophilized vesicles showed that the weight loss of the SDS/DTAB@EP-β-CD aggregates prepared at 25°C was 2.642%, while 2.437% for the samples at 35°C, which indicated that there was less structural water in SDS/DTAB@EP-β-CD vesicles prepared at 35°C. This structural water can be attributed to the bonding of water molecules to the hydroxyl groups of EP-β-CD on the surface of vesicles [44]. Moreover, in addition to participating in hydrogen bonds, the distribution of water molecules also affected the curvature of the formed vesicles. In other words, the higher the self-assembly temperature, the fewer water molecules involved, resulting in larger curvature and larger vesicles, and such result was consistent with DLS characterization.
3.3 The Possible Mechanism of the Morphology Transition of SDS/DTAB@EP-β-CD Supramolecular Aggregates
Based on the above experimental results, a possible mechanism of the aggregate transformation process was proposed, as shown in Fig. 7. For the system containing SDS (15 mmol/L) and DTAB (5 mmol/L), the addition of EP-β-CD resulted in the incorporation of surfactant hydrophobic chains into the hydrophobic cavity of EP-β-CD. EP-β-CD selectively bound to the main components of the mixed surfactants system and wrapped them into the cavity. After the inclusion complex of EP-β-CD and mixed surfactants was formed, the concentration of free surfactants in the system decreased, and the volume of self-assembled aggregates increased, resulting in an increase in the critical packing parameter (PC) [45]. The hybrid system exhibited additional phase behavior due to the presence of different amounts of surfactant and the variation of PC. More specifically, the addition of EP-β-CD to a mixed solution of SDS (15 mmol/L)-DTAB (5 mmol/L) at 15°C resulted in the aggregate transition phenomenon, whereby circular micelles were transformed into worm-like micelles. As the temperature increases, the hydrogen bonds between EP-β-CD molecules may become weaker than those between EP-β-CD and H2O molecules or between the H2O molecules themselves, allowing water molecules to participate in the adjacent in hydrogen bond between two SDS/DTAB@EP-β-CD. Indeed, mediated water played an important role in the interaction between SDS/DTAB@EP-β-CD; such a molecular model allowed for greater curvature, which ultimately led to the transformation of self-assembled aggregates from worm-like micelles to vesicles at higher temperatures.
Figure 7: Schematic illustration of the morphologic evolution of SDS/DTAB@EP-β-CD supramolecular aggregates induced by temperature
The present study mainly studied the self-assembly behavior of EP-β-CD and mixed anionic and cationic surfactant systems (SDS/DTAB) in an aqueous solution. Macroscopic observation, turbidity, and viscosity analyses showed that the mixture solution showed different properties with the change of temperature. TEM and DLS analyses showed that SDS/DTAB@EP-β-CD, as the basic building block of the supramolecular system, could self-assemble into worm-like micelles at lower temperatures and vesicles at higher temperatures. FT-IR, NMR, TG, and DSC analyses indicated that the driving force for the formation of vesicles and worm-like micelles was hydrogen bonds between EP-β-CD molecules, while water molecules played an important role in promoting vesicle formation between SDS/DTAB@EP-β-CD units. This paper mainly discussed the mechanism of the morphologic transformation of SDS/DTAB@EP-β-CD supramolecular aggregates induced by temperature, which may provide an excellent basis for the development of bio-based delivery carriers.
Acknowledgement: None.
Funding Statement: This work was supported by grants from China Postdoctoral Science Foundation (2020M681125), National Natural Science Foundation of China (32272254, 31901618), and Collaborative Innovation Center of Fragrance Flavour and Cosmetics.
Author Contributions: Study conception and design: X. Kou, Q. Ke; data collection: W. Xu; analysis and interpretation of results: Z. Xiao; draft manuscript preparation: Q. Meng. All authors reviewed the results and approved the final version of the manuscript.
Availability of Data and Materials: Data is available on request from the authors.
Conflicts of Interest: The authors declare that they have no conflicts of interest to report regarding the present study.
References
1. Kou X, Zhang Y, Su D, Wang H, Huang X, Niu Y, et al. Study on host-guest interaction of aroma compounds/γ-cyclodextrin inclusion complexes. LWT. 2023;178:114589. doi:10.1016/j.lwt.2023.114589. [Google Scholar] [CrossRef]
2. Alami E, Abrahmsén-Alami S, Eastoe J, Grillo I, Heenan RK. Interactions between a nonionic gemini surfactant and cyclodextrins investigated by small-angle neutron scattering. J Colloid Interface Sci. 2002;255(2):403–9. doi:10.1006/jcis.2002.8616 [Google Scholar] [PubMed] [CrossRef]
3. Antonietti M, Förster S. Vesicles and liposomes: a self-assembly principle beyond lipids. Adv Mater Deerfield. 2003;15(16):1323–33. doi:10.1002/adma.v15:16. [Google Scholar] [CrossRef]
4. Bennevault V, Huin C, Guégan P, Evgeniya KG, Qiu X, Winnik FM. Temperature sensitive supramolecular self assembly of per-6-PEO-β-cyclodextrin and α,ω-di-(adamantylethyl)poly(N-isopropylacrylamide) in water. Soft Matter. 2015;11(32):6432–43. doi:10.1039/C5SM01293G [Google Scholar] [PubMed] [CrossRef]
5. Kou X, Zhang X, Ke Q, Meng Q. Pickering emulsions stabilized by β-CD microcrystals: construction and interfacial assembly mechanism. Front Nutr. 2023;10:1161232. doi:10.3389/fnut.2023.1161232 [Google Scholar] [PubMed] [CrossRef]
6. Bianchini G, Bazan M, Fabris F, Scarso A. β-cyclodextrin supramolecular recognition of bis-cationic dithienylethenes. Organics. 2022;3(2):77–86. doi:10.3390/org3020005. [Google Scholar] [CrossRef]
7. Chen C, Chen Y, Dai X, Li J, Jia S, Wang S, et al. Multicharge β-cyclodextrin supramolecular assembly for ATP capture and drug release. Chem Commun. 2021;57(22):2812–5. doi:10.1039/D1CC00292A [Google Scholar] [PubMed] [CrossRef]
8. Chen Q, Bai H, Guo JC, Miao CQ, Li SD. Perfectly planar concentric π-aromatic B18H3−, B18H4, B18H5+, and B18H62+ with [10] annulene character. Phys Chem Chem Phys. 2011;13(46):20620–6. doi:10.1039/c1cp21927h [Google Scholar] [PubMed] [CrossRef]
9. Cheng J, Tian B, Huang Q, Ge H, Wang ZZ. Resveratrol functionalized carboxymethyl-βcyclodextrin: Synthesis, characterization, and photostability. J Chem. 2018;2018:6789076. [Google Scholar]
10. Filippini G, Goujon F, Bonal C, Malfreyt P. Host-guest complexation in the ferrocenyl alkanethiolsthio β-cyclodextrin mixed self-assembled monolayers. J Phys Chem C. 2014;118(6):3102–9. doi:10.1021/jp4114128. [Google Scholar] [CrossRef]
11. Iakimov NP, Zotkin MA, Dets EA, Abramchuk SS, Arutyunian AM, Grozdova ID, et al. Evaluation of critical packing parameter in the series of polytyrosine-PEG amphiphilic copolymers. Colloid Polym Sci. 2021;299(10):1543–55. doi:10.1007/s00396-021-04853-2. [Google Scholar] [CrossRef]
12. Ivanov AA, Falaise C, Abramov PA, Shestopalov MA, Kirakci K, Lang K, et al. Host-guest binding hierarchy within redox-and luminescence-responsive supramolecular self-assembly based on chalcogenide clusters and γ-cyclodextrin. Chemistry. 2018;24(51):13467–78. doi:10.1002/chem.v24.51. [Google Scholar] [CrossRef]
13. Jiang L, Deng M, Wang Y, Liang D, Yan Y, Huang J. Special effect of β-cyclodextrin on the aggregation behavior of mixed cationic/anionic surfactant systems. J Phys Chem B. 2009;113(21):7498–504. doi:10.1021/jp811455f [Google Scholar] [PubMed] [CrossRef]
14. Jiang L, Peng Y, Yan Y, Deng M, Wang Y, Huang J. Annular Ring microtubes formed by SDS@2β-CD complexes in aqueous solution. Soft Matter. 2010;6(8):1731–6. doi:10.1039/b920608f. [Google Scholar] [CrossRef]
15. Jiang L, Peng Y, Yan Y, Huang J. Aqueous self-assembly of SDS@2β-CD complexes: lamellae and vesicles. Soft Matter. 2011;7(5):1726–31. doi:10.1039/C0SM00917B. [Google Scholar] [CrossRef]
16. Jiang L, Yan Y, Huang J. Versatility of cyclodextrins in self-assembly systems of amphiphiles. Adv Colloid Interface Sci. 2011a;169(1):13–25. doi:10.1016/j.cis.2011.07.002 [Google Scholar] [PubMed] [CrossRef]
17. Jiang L, Yan Y, Huang J. Zwitterionic surfactant/cyclodextrin hydrogel: microtubes and multiple responses. Soft Matter. 2011b;7(21):10417–23. doi:10.1039/c1sm06100c. [Google Scholar] [CrossRef]
18. Jiang L, Yan Y, Huang J, Yu C, Jin C, Deng M, et al. Selectivity and stoichiometry boosting of β-cyclodextrin in cationic/anionic surfactant systems: when host−guest equilibrium meets biased aggregation equilibrium. J Phys Chem B. 2010;114(6):2165–74. doi:10.1021/jp911092y [Google Scholar] [PubMed] [CrossRef]
19. Ketner AM, Kumar R, Davies TS, Elder PW, Raghavan SR. A simple class of photorheological fluids: surfactant solutions with viscosity tunable by light. J Am Chem Soc. 2007;129(6):1553–9. doi:10.1021/ja065053g [Google Scholar] [PubMed] [CrossRef]
20. Li J, Sun Z, Zhang F. Accounting for the surface temperature persistence by using signal energy. Theor Appl Climatol. 2021;144(1):363–77. [Google Scholar]
21. Li S, Zhang L, Wang B, Ma M, Xing P, Chu X, et al. An easy approach for constructing vesicles by using aromatic molecules with β-cyclodextrin. Soft Matter. 2015;11(9):1767–77. doi:10.1039/C4SM02339K [Google Scholar] [PubMed] [CrossRef]
22. Araújo LDSS, Watson L, Traore DA, Lazzara G, Chiappisi L. Hierarchical assembly of pH-responsive surfactant-cyclodextrin complexes. Soft Matter. 2022;18(35):6529–37. doi:10.1039/D2SM00807F [Google Scholar] [PubMed] [CrossRef]
23. Liu K, Ma C, Wu T, Qi W, Yan Y, Huang J. Recent advances in assemblies of cyclodextrins and amphiphiles: construction and regulation. Curr Opin Colloid Interf Sci. 2020;45:44–56. doi:10.1016/j.cocis.2019.11.007. [Google Scholar] [CrossRef]
24. Liu Z, Liu Y. Multicharged cyclodextrin supramolecular assemblies. Chem Soc Rev. 2022;51(11):4786–827. doi:10.1039/D1CS00821H [Google Scholar] [PubMed] [CrossRef]
25. Lu B, Li L, Wei L, Guo X, Hou J, Liu Z. Synthesis and thermo-responsive self-assembly behavior of amphiphilic copolymer β-CD-(PCL-P(MEO2MA-co-PEGMA))21 for the controlled intracellular delivery of doxorubicin. RSC Adv. 2016;6(56):50993–1004. doi:10.1039/C6RA08108H. [Google Scholar] [CrossRef]
26. Machado MVH, Rodrigues-Junior G, Malachias A. Emergence of supramolecular order from combined linear amphiphilic and diphosphonate molecules. Langmuir. 2021;37(12):3685–93. doi:10.1021/acs.langmuir.1c00075 [Google Scholar] [PubMed] [CrossRef]
27. Sun P, Qin B, Xu JF, Zhang X. Supramonomers for controllable supramolecular polymerization and renewable supramolecular polymeric materials. Prog Polym Sci. 2022;124:101486. doi:10.1016/j.progpolymsci.2021.101486. [Google Scholar] [CrossRef]
28. Mohandoss S, Palanisamy S, You S, Vinosha M, Rajasekar P, Velu KS, et al. Nanofibers from hydroxypropyl β-cyclodextrin/pantothenic acid supramolecular complexes: physicochemical characterization and potential biomedical applications. J Ind Text. 2022;51(4_suppl):6276S–6297S. doi:10.1177/15280837221082032. [Google Scholar] [CrossRef]
29. Pimple P, Singh P, Prabhu A, Gupta S. Development and optimization of HP-β-cd inclusion complex-based fast orally disintegrating tablet of pitavastatin calcium. J Pharm Innov. 2022;17(3):993–1010. doi:10.1007/s12247-022-09661-x. [Google Scholar] [CrossRef]
30. Schneider HJ, Hacket F, Rüdiger V, Ikeda H. NMR studies of cyclodextrins and cyclodextrin complexes. Chem Rev. 1998;98(5):1755–86. doi:10.1021/cr970019t [Google Scholar] [PubMed] [CrossRef]
31. Valente AJM, Söderman O. The formation of host-guest complexes between surfactants and cyclodextrins. Adv Colloid Interface Sci. 2014;205:156–76. doi:10.1016/j.cis.2013.08.001 [Google Scholar] [PubMed] [CrossRef]
32. Wang H, Zheng X. Theoretical study of macrocyclic host molecules: from supramolecular recognition to self-assembly. Phys Chem Chem Phys. 2022;24(32):19011–19028. doi:10.1039/D2CP02152H [Google Scholar] [PubMed] [CrossRef]
33. Wankar J, Kotla NG, Gera S, Rasala S, Pandit A, Rochev YA, et al. Recent advances in host-guest self-assembled cyclodextrin carriers: implications for responsive drug delivery and biomedical engineering. Adv Funct Mater. 2020;30(44):1909049. doi:10.1002/adfm.v30.44. [Google Scholar] [CrossRef]
34. Weiss-Errico MJ, Ghiviriga I, O’Shea KE. 19F NMR characterization of the encapsulation of emerging perfluoroethercarboxylic acids by cyclodextrins. J Phys Chem B. 2017;121(35):8359–66. doi:10.1021/acs.jpcb.7b05901 [Google Scholar] [PubMed] [CrossRef]
35. Xu D, Ma Y, Jing Z, Han L, Singh B, Feng J, et al. π-π interaction of aromatic groups in amphiphilic molecules directing for single-crystalline mesostructured zeolite nanosheets. Nat Commun. 2014;5:4262. doi:10.1038/ncomms5262 [Google Scholar] [PubMed] [CrossRef]
36. Xu HN, Ma SF, Chen W. Unique role of β-cyclodextrin in modifying aggregation of Triton X-114 in aqueous solutions. Soft Matter. 2012;8(14):3856–63. doi:10.1039/c2sm07371d. [Google Scholar] [CrossRef]
37. Yang S, Yan Y, Huang J, Petukhov AV, Kroon-Batenburg LMJ, Drechsler M, et al. Giant capsids from lattice self-assembly of cyclodextrin complexes. Nat Commun. 2017;8:15856. doi:10.1038/ncomms15856 [Google Scholar] [PubMed] [CrossRef]
38. Acharjee D, Das A, Panda MK, Barai M, Ghosh S. Facet engineering for decelerated carrier cooling in polyhedral perovskite nanocrystals. Nano Lett. 2023;23(5):1946–53. doi:10.1021/acs.nanolett.2c05107 [Google Scholar] [PubMed] [CrossRef]
39. Yuan X, Liu G, Zhang P, Xu W, Tang K. Lipase-catalyzed production of (S)-carprofen enhanced by hydroxyethyl-β-cyclodextrins: experiment and optimization. Organ Process Res Dev. 2019;23(5):891–9. doi:10.1021/acs.oprd.9b00009. [Google Scholar] [CrossRef]
40. Zhang J, Liu C, Hu X, Lv Q, Zhang H, Pi B, et al. Supramolecular self-assembly of the γ-cyclodextrin and perfluorononanoic acid system in aqueous solution. Soft Matter. 2021;17(5):1428–36. doi:10.1039/D0SM01744B [Google Scholar] [PubMed] [CrossRef]
41. Zhang J, Shen X. Temperature-induced reversible transition between vesicle and supramolecular hydrogel in the aqueous ionic liquid-β-cyclodextrin system. J Phys Chem B. 2013;117(5):1451–7. doi:10.1021/jp308877w [Google Scholar] [PubMed] [CrossRef]
42. Ke Q, Wang H, Huang X, Zhang Y, Meng Q, Kou XR. Direct addition of vanillin improved the physicochemical properties and antibacterial activities of gelatin/sodium carboxymethyl cellulose composite film. Ind Crops Prod. 2023;206:117653. doi:10.1016/j.indcrop.2023.117653. [Google Scholar] [CrossRef]
43. Xie W, Bao Q, Liu Y, Wen H, Wang Q. Hydrogen bond association to prepare flame retardant polyvinyl alcohol film with high performance. ACS Appl Mater Interf. 2021;13(4):5508–17. doi:10.1021/acsami.0c19093 [Google Scholar] [PubMed] [CrossRef]
44. Zhou C, Huang J, Yan Y. Chain length dependent alkane/β-cyclodextrin nonamphiphilic supramolecular building blocks. Soft Matter. 2016;12(5):1579–85. doi:10.1039/C5SM02698A [Google Scholar] [PubMed] [CrossRef]
45. Zhu B, Jia L, Guo X, Yin J, Zhao Z, Chen N, et al. Controllable assembly of a novel cationic gemini surfactant containing a naphthalene and amide spacer with β-cyclodextrin. Soft Matter. 2019;15(15):3198–207. doi:10.1039/C9SM00172G [Google Scholar] [PubMed] [CrossRef]
Cite This Article
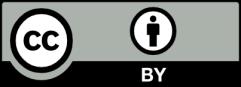
This work is licensed under a Creative Commons Attribution 4.0 International License , which permits unrestricted use, distribution, and reproduction in any medium, provided the original work is properly cited.