Open Access
REVIEW
The Application of Solid Waste in Thermal Insulation Materials: A Review
1 School of Environmental Science and Engineering, Changzhou University, Changzhou, 213164, China
2 School of Urban Construction, Changzhou University, Changzhou, 213164, China
* Corresponding Author: Pinghua Zhu. Email:
(This article belongs to the Special Issue: Special Issue in Celebration of JRM 10 Years)
Journal of Renewable Materials 2024, 12(2), 329-347. https://doi.org/10.32604/jrm.2023.045381
Received 25 August 2023; Accepted 19 October 2023; Issue published 11 March 2024
Abstract
As socioeconomic development continues, the issue of building energy consumption has attracted significant attention, and improving the thermal insulation performance of buildings has become a crucial strategic measure. Simultaneously, the application of solid waste in insulation materials has also become a hot topic. This paper reviews the sources and classifications of solid waste, focusing on research progress in its application as insulation materials in the domains of daily life, agriculture, and industry. The research shows that incorporating household solid waste materials, such as waste glass, paper, and clothing scraps into cementitious thermal insulation can significantly reduce the thermal conductivity of the materials, leading to excellent thermal insulation properties. Insulation materials prepared from agricultural solid waste, such as barley straw, corn stalk, chicken feather, and date palm fibers, possess characteristics of lightweight and strong thermal insulation. Industrial solid waste, including waste tires, iron tailings, and coal bottom ash, can also be utilized in the preparation of insulation materials. These innovative applications not only have positive environmental significance by reducing waste emissions and resource consumption, but also provide efficient and sustainable insulation solutions for the construction industry. However, to further optimize the mix design and enhance the durability of insulation materials, continuous research is required to investigate the mechanisms through which solid waste impacts the performance of insulation materials.Keywords
With the rapid development of the social economy, energy issues have become the focus of public concern, people pay more attention to the thermal insulation performance of buildings. Good building insulation materials not only ensure the thermal comfort of occupants but also greatly enhance energy utilization efficiency [1]. Given that individuals spend nearly 90% of their time indoors, creating a thermally comfortable environment plays a pivotal role in safeguarding the quality of life [2].
However, with the rapid growth of global industry, the increase in energy consumption and pollutant emissions has become a pressing issue. According to statistics, the construction industry accounts for 20%~60% of total energy consumption in various countries and regions [3,4]. Over the past four decades, energy demand in the construction sector has continued to rise at an annual rate of 1.8%. It is projected that by 2050, this demand will increase from 279 billion tons of oil equivalent in 2010 to more than 440 billion tons of oil equivalent, with the majority of this growth occurring in developing countries [5]. Furthermore, greenhouse gas emissions from the construction sector account for one-third of total emissions [6]. It is worth noting that carbon dioxide emissions constitute three-quarters of global greenhouse gas emissions and are widely recognized as a primary driver of climate change [7]. Therefore, the construction sector plays a crucial role in reducing carbon dioxide emissions.
In recent years, materials with low heat conductivity and high insulation efficiency have been widely used in building insulation systems [8,9]. Throughout the research and application of building insulation materials, there has been a transition from organic to inorganic materials. Initially, organic materials such as polystyrene particles [10], polystyrene boards [11], extruded boards [12], and polyurethane [13] were employed as building insulation materials due to their advantages, including low thermal conductivity and good insulation properties. However, these materials had inherent fatal flaws, such as easy-aging, flammability, and poor high-temperature resistance, resulting in serious safety hazards. Consequently, they have gradually been replaced by inorganic materials. Inorganic materials, such as vitrified beads [14], expanded perlite [15], rock wool [16], and expanded vermiculite [17], as insulation aggregates, employed as building insulation materials due to their advantages, including low thermal conductivity and good insulation properties. Have been rapid development and demonstrated excellent thermal insulation properties. However, these insulation mortars exhibit challenges like high water absorption rates, increased thermal conductivity after moisture absorption, easy cracking, and limited durability.
Aerogels are regarded as ideal insulation materials due to their high porosity, low density, and favorable thermal insulating properties, having a wide range of application prospects [18]. However, their high production costs and intricate manufacturing processes constrain their application on a large scale [19]. Therefore, the pressing need is to develop a new, low-cost, high-performance insulation material that can be widely accepted and applied within society. Some scholars argued that more effective utilization of existing resources is a feasible way to alleviate energy shortages [20]. Abdulmunem et al. [21–23] used waste paper and waste chicken feathers as raw materials for the preparation of thermal insulation materials. The results showed that these new thermal insulation materials produced from waste had good sound and heat insulation effects, contributing to reducing the cold and heat load of the building. This initiative not only achieves resourceful utilization of solid waste but also reduces cost.
With the acceleration of population growth and urbanization, the rate of solid waste generation is a worrisome trend, especially in developing countries. Statistical data indicates that generated solid waste includes municipal waste, construction debris, and electronic waste [24]. The accumulation and burning of large quantities of waste are shown in Fig. 1. Toxic and hazardous substances within these solid wastes, if not properly managed, pose significant threats to the environment and human health. Therefore, strengthening the management and treatment of solid waste, promoting waste resource utilization and reduction, has become a critical challenge faced by the global community.
Figure 1: Solid waste accumulation and incineration
This paper comprehensively reviews the application of solid waste in insulation materials. It summaries the thermal conductivity, mechanical properties, density, and water absorption of thermal insulation materials prepared from solid wastes and analyses them economically. Also, limitations of using waste materials as insulation are discussed. This paper proposes viable methods to promote resourceful utilization of solid wastes to contribute to environmental protection.
2 Sources and Classification of Solid Waste
According to different sources of solid waste, it can be primarily categorized into domestic solid waste (DSW), industrial solid waste (ISW), and agricultural solid waste (ASW). A schematic representation of the sources and classifications of solid waste is illustrated in Fig. 2.
Figure 2: Schematic diagram of solid waste sources and classification
DSW refers to the collective term for various discarded materials generated in the daily lives of urban and suburban residents, such as waste paper, waste glass, scrap metal, ceramics, and discarded batteries [25]. Common examples of DSW are depicted in Fig. 3.
Figure 3: Domestic solid waste
Currently, the predominant methods for managing DSW largely involve landfilling and incineration. Among the collected waste, 70% is directly disposed of in landfills, 19% is recycled, and 11% is utilized for energy recovery, with landfilling being a traditional disposal method [26]. However, concerns have arisen due to issues such as land occupation by landfill sites and the potential leakage of pollutants. Incineration, on the other hand, is a process in which DSW is subjected to high-temperature thermal decomposition, converting it into ashes and gases [24]. However, this process generates significant quantities of harmful gases, including carbon dioxide and sulfur dioxide, resulting in adverse environmental and health effects.
ASW refers to various solid waste materials generated in agricultural production and rural living. Globally, billions of tons of agricultural waste are produced annually [27], primarily including crop residues, livestock and poultry manure, and agricultural pesticide packaging waste [28]. Common examples of ASW are shown in Fig. 4.
Figure 4: Agricultural solid waste
The management of ASW is a common concern in both developed and developing countries. Usually, these wastes are either directly dumped in landfills or subjected to incineration. In landfills, solid waste containing organic components undergoes microbial decomposition, generating leachate that can lead to groundwater contamination. Furthermore, the decomposition of organic matter releases methane gas, which possesses a potent greenhouse effect, with a warming potential 25 times that of CO2 [29]. The pollution of the environment and disruption of ecosystems by ASW not only impact human health but also pose potential hazards to animal well-being.
ISW refers to the solid waste materials generated during industrial production processes. In 2019, China’s ISW production reached a staggering 3.543 billion tons [30]. This category encompasses various waste materials, including dust, slag, and other residues produced in processes such as metal smelting, electricity generation, and chemical manufacturing [31]. Common examples of ISW are depicted in Fig. 5.
Figure 5: Industrial solid waste
The accumulation of ISW not only occupies land resources, but also produces dust, polluting the air and exacerbating air quality issues. Furthermore, these wastes contain significant quantities of heavy metals and alkaline substances that can potentially leach into the ground, contaminating groundwater resources and leading to soil salinization [32]. Therefore, effective utilization of ISW faces formidable challenges.
3 Application of Solid Waste in Thermal Insulation Materials
Using solid wastes as insulation materials has become a hot topic in the field of sustainable development. Firstly, compared with traditional thermal insulation materials, the use of solid waste can reduce environmental pollution. Secondly, the thermal insulation performance of these materials is comparable to that of traditional materials, with longer service life and lower maintenance costs. Finally, the use of solid waste also reduces dependence on limited resources, mitigates environmental degradation, and promotes sustainable development. In conclusion, the use of solid waste in insulation materials is an environmentally friendly and sustainable initiative.
3.1 Application of Domestic Solid Waste in Thermal Insulation Materials
With the growth of urban populations and an increase in people’s living standards, more frequent production and consumption activities have resulted in the generation of a greater volume of domestic solid waste. In order to reduce the environmental impact of waste, people are increasingly exploring how to convert waste into resources. This includes the widespread utilization of waste materials such as waste glass, waste woolen clothes, and waste paper as insulation materials.
Waste glass is a highly non-degradable solid waste material that is ubiquitous in our daily lives [33]. According to statistics, waste glass accounts for 5% of the total urban household waste [34]. The non-degradability of waste glass not only poses a threat to human health, but also causes varying degrees of harm to livestock [35]. Due to its unique properties, insulation materials made from waste glass exhibit outstanding durability, resulting in a long service life that effectively protects buildings [36]. Flores-Alés et al. [37] utilized glass waste to replace sand in the preparation of cement mortar, effectively improving the thermal performance and reducing the density of the mortar. However, its mechanical behavior is not uniform and is influenced by the composition, quantity, and manufacturing process of the specimens. Yousefi et al. [38] discovered that replacing natural sand with waste glass and adding nano-titanium dioxide as a nanofiller substantially improved the compressive strength and water resistance of the mortar. These studies demonstrate that the reuse of waste glass not only reduces environmental pollution but also provides a sustainable material choice for applications in construction and industry.
In addition to waste glass, life’s waste woolen clothes, due to their unique thermal insulating properties, are suitable for the production of various types of insulation materials. Research conducted by Cardinale et al. [39] explored the potential application of wool in cement mortar boards, demonstrating that the addition of 2% wool fibers could achieve a thermal conductivity of 0.107 W/(m·K) in composite materials. El Wazna et al. [40] employed needle punching technique to fabricate four types of nonwoven waste using acrylic and wool as substrates. The results indicated that the thermal insulation performance of the wool samples outperformed the acrylic samples, reaching as low as 0.0339 W/(m·K). In addition, wool fibers can also enhance the mechanical properties of building materials. The study by Fiore et al. [41] found that as the fiber content increases, the thermal conductivity of wool-filled mortar decreases, with shorter fibers further reducing thermal conductivity.
In order to achieve efficient value addition of waste paper, Mandili et al. [42] have developed a new building insulation material based on waste paper and limestone aggregates. This material exhibits thermal conductivity ranging from 0.097~0.12 W/(m·K) and compressive strength in the range of 4.2~10.95 MPa. It possesses dual functionality, being capable of providing effective thermal insulation performance while also being suitable for structural applications. Additionally, Shibib [43] has identified significant potential in the utilization of waste paper in brick production, thanks to two factors. First of all, due to the release of a substantial amount of thermal energy during the combustion of waste paper in the firing process, bricks can exhibit uniform performance characteristics overall. Secondly, waste paper possesses a high calorific value, reducing the energy costs required for brick production and thus enhancing its environmental friendliness.
Overall, the utilization of DSW to produce insulation materials has made significant advancements in thermal insulation performance. For instance, waste glass can be used to produce building and insulation materials, enhancing durability and longevity. The incorporation of a certain amount of glass powder can reduce the material’s thermal conductivity while increasing compressive strength and water resistance. Lightweight bricks made from waste paper show excellent thermal insulation properties while remaining suitable for structural applications. The addition of wool noticeably reduces the material’s thermal conductivity.
Table 1 summarizes insulation materials prepared from other common DSW and their performance changes. Based on tabulated data, it is evident that the thermal insulation materials prepared with the addition of household solid waste consistently exhibit excellent heat insulation performance, with thermal conductivity values reaching below 0.2 W/(m·K). When incorporating 5% eggshells and 3% discarded glass bottles, the composite material’s thermal conductivity can be reduced to 0.037 W/(m·K), with a low density of 210 kg/m³ and a remarkably high porosity of 91.4%. However, the compressive strength is relatively low, only 1.12 MPa. On the other hand, incorporating 40% waste paper can increase the material’s compressive strength to 10.95 MPa, while maintaining a relatively high porosity. Nevertheless, an excessive amount of waste paper can lead to an increase in the material’s water absorption rate. In summary, using DSW to produce building insulation materials shows great potential for environmental and cost benefits. Importantly, the mix designs need optimization for different wastes to balance insulation and mechanical properties.
3.2 Application of Agricultural Solid Waste in Insulation Materials
Utilizing ASW as insulation material represents an economically prudent choice. These waste materials are typically readily available at little to no cost, and the expenses associated with processing them into insulation materials are relatively modest. This approach not only reduces costs within sectors such as construction, and farming but also yields economic benefits. Furthermore, these waste materials constitute renewable resources, contributing to a reduction in reliance on natural resources through recycling.
It is estimated that China produces over 700 million tons of crop straw annually, making it one of the world’s largest agricultural nations [52]. The primary sources of straw include wheat straw, rice straw, and corn straw. Currently, these agricultural and forestry residues are typically disposed of through on-site burning, with approximately 280 million tons of crop straw being burned in fields each year. This kind of burning or haphazardly discarding straw not only severely pollutes the environment but also results in wastage of valuable resources [53]. However, straw is widely used as an insulating material due to its low density, porous structure, rich source, and good insulating properties [54].
Jiang et al. [52] employed straw and fallen leaves fibers as organic thermal insulation materials to prepare plant fiber cement-based composite insulation mortar. The results showed that adding wheat straw fibers and straw fibers improved the uniformity of the composite mortar. Mixing leaf fibers with some wheat straw fibers or straw fibers could enhance the strength of the composite insulation mortar. Dou et al. [55] used hydrated lime and rice straw to prepare insulation blocks and assessed the impact of expanded polystyrene waste on the insulation blocks. They found that slaked lime increased the dry apparent density and weight of the insulation blocks, while the expanded polystyrene waste effectively mitigated this negative impact, keeping the thermal conductivity of the insulation blocks below 0.049 W/(m·K).
On the other hand, Laborel-Préneron et al. [56] utilized barley straw, hemp shiv, and corn cob to prepare insulation materials. They found that among these three materials, barley straw exhibited superior insulation performance, reaching 0.14 W/(m·K). Furthermore, it displayed good mechanical properties when coarser particles were added. Similarly, Liu et al. [57] observed that trace amounts of slightly cadmium-enriched kenaf straw had a positive impact on the thermal performance of cement mortar. When the content of slightly cadmium-enriched kenaf straw was 8% of the mass of the cementitious material, the mortar’s thermal conductivity decreased by 37%, and its dry density decreased by 27%. The 28 d compressive strength of the cement mortar was 5.55 MPa, still meeting engineering requirements.
Karapinar [58] used corn stalk as raw material to produce a polyurethane insulation material. When the corn stalk content was 10%, the thermal conductivity of the insulation material decreased by 22%, reaching 0.042 W/(m·K). This is of significant importance for the utilization of agricultural waste as a new type of insulation material. Shao et al. [59] explored the use of processed corn cob aggregate to replace river sand in mortar production. They found that the processed corn cob aggregate had almost no inhibitory effect on the hydration products of cement and could even accelerate cement hydration. However, a high content of corn cob aggregate reduced the workability and strength of the mortar while enhancing its ductility and insulation performance. The addition of corn cob aggregate reduced the thermal conductivity of the mortar and increased its water vapor permeability, making it suitable for lightweight structural concrete production.
Date palm fibers is widely used in insulation materials due to its lightweight properties and excellent insulating performance. Boumhaout et al. [60] found that when date palm fibers was incorporated into composite materials, the thermal conductivity of the composite decreased by 70%, thermal diffusivity decreased by 52%, density decreased by 39%. However, its influence on mechanical strength was relatively minor. Benmansour et al. [61] demonstrated that when the date palm fibers content was below 15%, the composite material met the thermal and mechanical requirements of building materials and could be used in building components as a novel bio-based composite material for energy-efficient construction. Benaniba et al. [62] through further research found that with increasing date palm fibers content, the composite material’s thermal conductivity, flexural strength, and compressive strength significantly decreased. When the coconut palm fiber content was 6%, the compressive strength of the material decreased by 16%. When the coconut palm fiber content reached 30%, the thermal conductivity of the composite material could be as low as 0.083 W/(m·K), making it possess excellent insulation properties.
With the continuous growth of the global population and economic development, there has been a steady increase in chicken meat consumption, leading to the generation of a substantial amount of chicken feather waste. About 65 million tons of chicken feathers are produced worldwide every year [63]. In European, it is estimated that chicken feather production reaches around 3.1 million tons annually [64]. The conventional methods for handling chicken feather waste involve landfilling and incineration. However, due to the highly toxic and non-degradable nature of chicken feathers, these treatment methods are easy to cause pollution of the air, soil, groundwater, and surface water [22]. Therefore, numerous research efforts are dedicated to exploring novel and high-value utilization pathways for chicken feathers.
Bessa et al. [65] evaluated the potential application of chicken feather fibers in reinforced polymer materials. It was found that the thermal conductivity of the composites decreased from 0.175 to 0.128 W/(m·K) with the increase in the mass content of chicken feather fibers, which may be related to the unique structural morphology of chicken feather fibers. These fibers contain a large number of hollow channels within them, which are helpful in improving the thermal performance of composites. Fedorik et al. [66] investigated the thermal insulation properties of several bio-based insulation materials. The results showed that the thermal conductivity of peat, recycled paper, wood chips, and chicken feathers was 0.033~0.044 W/(m·K) at 10°C. And among these materials, the chicken feather-based thermal insulator had the best thermal performance.
In a word, date palm fibers and straw, due to their lightweight nature and excellent insulation properties, hold significant promise in the field of insulation materials. The addition of date palm fibers can reduce the thermal conductivity and thermal diffusivity of composite materials, enhance thermal damping, and simultaneously reduce material density. In the construction sector, the combined use of date palm fibers with other organic fibers can meet both thermal and mechanical requirements, potentially serving as novel materials for energy-efficient construction. However, an excessive amount of date palm fibers can reduce thermal conductivity, flexural strength, and compressive strength, so it is necessary to balance between fiber content and material properties. Similarly, plant fibers like straw exhibit excellent insulation properties and can be used to produce products such as composite insulation mortar and other products. Waste chicken feather fibers are also gradually coming into people’s view because of its good thermal insulation performance. However, the impact of different plant fibers on insulation material performance may vary, requiring optimization based on specific applications.
Table 2 summarizes the preparation of insulation materials using other common ASW and their resulting performance changes. From the data in the table, it can be observed that composite materials prepared with the addition of 20% bagasse waste fibers exhibit outstanding thermal insulation performance, with a thermal conductivity as low as 0.034 W/(m·K), along with excellent sound absorption properties and noise reduction effects. Furthermore, insulation materials prepared from groundnut shells demonstrate good overall performance. When the groundnut shell content is 20%, the composite material has a thermal conductivity of 0.156 W/(m·K), a tensile strength of up to 15.6 MPa, and a flexural strength of 37.6 MPa. In conclusion, insulation materials prepared from plant fibers not only provide insulation effectiveness but also effectively utilize agricultural waste, offering environmental and sustainable development advantages.
3.3 Application of Industrial Solid Wastes in Insulation Materials
ISW refers to discarded solid materials generated during industrial production processes, in 2011, global industrial waste production amounted to approximately 9.2 billion tons, with China contributing 3.2 billion tons to this total [78]. According to data, in 2019, China’s industrial solid waste generation reached approximately 3.543 billion tons, primarily consisting of tailings, red mud, aluminum dust, carbide slag, flue gas desulfurization gypsum, fly ash, coal gangue, and waste tires [79]. These wastes are typically byproducts, residues, wastewater, or emissions produced during industrial production processes. They may contain toxic and harmful substances, posing potential threats to the environment and human health. Therefore, it is very important to strengthen the control, supervision, and management of ISW.
With the improvement of living standards, there is an increasing demand for private vehicles, leading to a corresponding increase in the quantity of waste tires. The global production of tires has reached approximately 1.5 billion units, while the number of tires that have reached the end of their usable life is estimated to be around 1 billion units [80]. It is projected that by 2030, more than 1.2 billion tires will reach the end of their lifespan annually [81]. However, a concerning issue is that still more than 50% of waste tires are either landfilled or disposed of without proper treatment, which has caused great environmental challenges [82]. The rubber materials present in waste tires can be efficiently employed for soundproofing and thermal insulation, exhibiting excellent insulation properties.
Kazmierczak et al. [83] utilized scrap rubber from waste tires to prepare cement composite materials. When adding 6% rubber powder, the thermal conductivity of the mortar decreased by 57%, thus enhancing its performance as an insulating material. Furthermore, Qin et al. [84] conducted surface modification of silicone rubber particles to enhance their hydrophilicity. Experimental results demonstrated that after surface modification, the strength of silicone rubber mortar increased, its thermal conductivity improved, porosity increased, and its thermal and noise insulation effects were more effective. In the study by Na et al. [85], it was found that the addition of cellulose ether, re-dispersible latex powder, and wood fibers improved the mechanical properties of silicone rubber mortar. When using micrometer-sized rubber granules, the mortar exhibited favorable drying shrinkage performance.
In addition to using scrap rubber, incorporating waste tires rubber into mortar using various binder materials can also yield high-performance mortars. Bostanci [86] employed the combined action of silica fume and scrap rubber in alkali-activated slag mortar. Experimental results show that when scrap rubber content was 3.0% and silica fume content was 0.3%, a synergistic effect was observed. This not only enhanced the thermal insulation performance of the specimens but also increased the compressive strength of the samples by 48.35%. Furthermore, Wongsa et al. [87] conducted research on lightweight polymer mortar using 100% recycled crumb rubber as fine aggregates. Their study revealed that the density of the lightweight fly ash-based polymer mortar ranged from 1067~1275 kg/m³, with a thermal conductivity of 0.237~0.298 W/(m·K). However, the compressive strength of the mortar was significantly reduced, accompanied by increased porosity and water absorption. This indicates that the scrap rubber from waste tires possesses excellent soundproofing and thermal insulation properties, making it an effective choice for insulation materials.
Sludge bottom ash is a common industrial waste used in insulation materials. Torkittikul et al. [88] found that use of coal bottom ash had almost no effect on the compressive strength of concrete. Wang et al. [89] added wood fibers from paper sludge to cement-based thermal insulation mortar, which greatly improved the ductility and softening coefficient of the paper sludge mortar. It also exhibited a low thermal conductivity ranging from 0.0897~0.0885 W/(m·K), making it a suitable thermal insulation material. Finally, Lee et al. [90] discovered that mortar with added coal bottom ash had a lightweight proportion of around 30% compared to regular mortar, but its thermal conductivity increased by approximately 30% compared to standard mortar.
As a common plastic material, polystyrene is everywhere and provides us with great convenience. However, it poses potential health risks due to the emission of toxic smoke and gases when it decomposes at high temperatures, leading to respiratory and eye irritation and damage [91]. Nevertheless, owing to its excellent thermal insulating properties, it is frequently used in the production of thermal insulation materials to effectively impede heat transfer and facilitate resource reuse [92].
In recent years, many scholars have researched the reuse of waste polystyrene. Záleská et al. [93] used expanded polypropylene-based aggregate as a complete substitute for natural aggregates. They found that the addition of expanded polypropylene-based aggregate significantly improved the material’s thermal insulation performance, reduced the density of the composite material, increased porosity, resulting in an 82% decrease in the composite material’s thermal conductivity. On the other hand, Ferrández et al. [94] used expanded polystyrene waste containing graphite and mineral wool to prepare gypsum mortar. The expanded polystyrene waste reduced the density of the mortar, improved water absorption properties, and lowered the thermal conductivity of the gypsum mortar. Koksal et al. [95] studied the effect of expanded vermiculite and waste expanded polystyrene on the properties of cement-based mortar. They found that vermiculite and expanded polystyrene could be used to prepare mortars with densities ranging from 393~946 kg/m3, resulting in excellent thermal insulation performance with a thermal conductivity as low as 0.09 W/(m·K) and compressive strength ranging from 0.57~5.89 MPa, meeting the requirements for practical engineering applications.
In summary, ISW, such as byproducts or residues from industrial production, may contain toxic substances and should be controlled and reasonably utilized. Sludge bottom ash can also be employed in insulation materials, replacing some fine aggregates to reduce material density and thermal conductivity. Adding paper sludge to cement mortar can improve toughness and reduce thermal conductivity. Using waste polystyrene can reduce material density while enhancing insulation properties and flexural strength. Furthermore, scrap rubber from waste tires demonstrates outstanding thermal insulation performance, and the inclusion of a certain amount of rubber powder can substantially improve the thermal insulation performance of the material.
Table 3 summarizes other common ISW materials used in the production of insulation materials and their performance changes. From the table, it can be observed that composite materials prepared using industrial solid waste consistently exhibit excellent thermal insulation performance, with thermal conductivity values consistently below 0.137 W/(m·K). It is worth noting that when 45% iron tailings are added, the thermal insulation performance of the composite material significantly improves, reducing the thermal conductivity to as low as 0.032 W/(m·K), and maintaining a relatively low density. In addition, when the steel slag content is 6%, the composite material demonstrates excellent overall performance, with a low thermal conductivity of 0.08 W/(m·K) and remarkable compressive strength of up to 29.98 MPa. In a word, manufacturing insulation materials from industrial waste can reduce environmental pollution, provide sustainable material options, and further research should be done to improve the durability of material.
The use of solid waste materials as thermal insulation provides excellent thermal insulation performance and also reduces cost. Abdulmunem et al. [21,23] added waste paper and waste chicken feathers to polyvinyl chloride panels. It was found that the addition of waste paper and chicken feathers reduced the cooling and heating load by 19% and 20.3%, respectively, resulting in savings of 16.3% and 22.5% in electricity costs. Benallel et al. [107] investigated the use of waste paper and plant fibers for the preparation of thermal insulating materials, which not only have low thermal conductivity, but also reduce the total cost to 43 $/m3, which is a significant advantage over the traditional insulating materials expanded polystyrene [108] (67.22 $/m3) and glass felts [109] (154.25 $/m3), and the reused material is also more environmentally friendly. In addition, Yang et al. [30] produced two types of low-density foam concrete using limestone tailings and desulfurization gypsum, and the results showed that the profit of the concrete containing desulfurization gypsum was 170~245 CNY/m3, while the profit of the concrete containing limestone tailings was 225~295 CNY/m3. The use of solid waste as insulation materials holds great potential, not only for enhancing building performance and thermal comfort, but also for providing significant economic and environmental sustainability benefits.
In summary, the preparation of building materials based on solid waste and in-depth analysis of their mechanical and thermal insulation properties revealed a wide range of applications. However, there are also many shortcomings, and the limitations are summarized below:
1. Although waste glass can be used in mortars to enhance compressive strength and water resistance, its smooth surface properties are not ideal when replacing coarse aggregates, limiting bonding to the cement matrix. In order to make more effective use of waste glass, it is necessary to study the mixing design to reduce the alkali-silica reaction that may occur between silica and alkali in waste glass [34].
2. Waste chicken feathers can be used in the preparation of insulating materials, owing to the multiple internal hollow channels they contain, which reduce the thermal conductivity of composite materials. However, due to the naturally biodegradable nature of chicken feather waste, appropriate measures need to be taken to reduce the time required for its collection, cleaning, and disinfection in order to prevent unwanted biodegradation from occurring [22].
3. While the use of solid waste can reduce production costs to some extent, the collection, transport, and disposal of waste requires significant economic costs. In addition, the environmental impact of waste disposal needs to be considered. Therefore, when considering solid waste reuse or recycling, it is important to comprehensively assess its economic and environmental costs in order to achieve sustainable development goals.
4. Develop a greater variety of composite insulation materials, utilizing the synergistic effects of two or more types of solid waste to produce insulation materials with improved overall performance. Explore various methods for mortar preparation, incorporating 3D printing technology to develop solid waste insulation materials that are easy to shape and exhibit stable performance, thereby expanding their application methods.
This review provided a comprehensive survey of the sources of solid waste and categorized them into domestic solid waste, agricultural solid waste, and industrial solid waste. It also explores the utilization of various solid wastes as raw materials for the preparation of building insulation materials. Thermal conductivity, mechanical properties, density, and water absorption of building insulation materials were analyzed and an economic analysis was carried out. The results showed that the thermal insulation materials prepared from solid wastes can significantly reduce the cost and have good thermal insulation and mechanical properties, compared with the traditional thermal insulation materials. These solid wastes are characterized by their low density, porous structure, and excellent thermal insulation properties, which meet the requirements for building insulation materials. Therefore, more effective use of solid waste has become a feasible method to alleviate the shortage of resources.
Although solid wastes have a wide range of applications in building insulation materials, there are still certain problems such as high costs and carbon emissions during collection, transportation and disposal, which limit their further development in building insulation materials. In summary, the utilization of solid waste to prepare thermal insulation materials has great potential. This can not only effectively achieve the resource utilization of waste to achieve the goal of sustainable development, but also provide an efficient and environmentally friendly insulation solution for the construction industry.
Acknowledgement: The authors would like to thank the Changzhou City Key Laboratory of Building Energy-Saving Technology, and all the authors of the references for their research contributions.
Funding Statement: This research was funded by the National Natural Science Foundation of China (52078068), Postgraduate Research & Practice Innovation Program of Jiangsu Province (SJCX22_1391), the National Science Foundation of Jiangsu Province (BK20220626) and Changzhou Leading Innovative Talent Introduction and Cultivation Project (CQ20210085).
Author Contributions: The authors confirm contribution to the paper as follows: study conception and design: Ming Liu and Pinghua Zhu; data collection: Haichao Li and Xintong Chen; analysis and interpretation of results: Ming Liu and Xiancui Yan; draft manuscript preparation: Ming Liu and Pinghua Zhu. All authors reviewed the results and approved the final version of the manuscript.
Availability of Data and Materials: The authors confirm that the data supporting the findings of this study are available within the article.
Conflicts of Interest: The authors declare that they have no conflicts of interest to report regarding the present study.
References
1. Salakij, S., Yu, N., Paolucci, S., Antsaklis, P. (2016). Model-based predictive control for building energy management. I: Energy modeling and optimal control. Energy and Buildings, 133, 345–358. [Google Scholar]
2. Paraschiv, L. S., Acomi, N., Serban, A., Paraschiv, S. (2020). A web application for analysis of heat transfer through building walls and calculation of optimal insulation thickness. Energy Reports, 6, 343–353. [Google Scholar]
3. Zhang, Y., He, C. Q., Tang, B. J., Wei, Y. M. (2015). China’s energy consumption in the building sector: A life cycle approach. Energy and Buildings, 94, 240–251. [Google Scholar]
4. Ma, Y. X., Yu, C. (2020). Impact of meteorological factors on high-rise office building energy consumption in Hong Kong: From a spatiotemporal perspective. Energy and Buildings, 228, 110468. [Google Scholar]
5. Nejat, P., Jomehzadeh, F., Taheri, M. M., Gohari, M., Abd. Majid, M. Z. (2015). A global review of energy consumption, CO2 emissions and policy in the residential sector (with an overview of the top ten CO2 emitting countries). Renewable and Sustainable Energy Reviews, 43, 843–862. [Google Scholar]
6. Robert, A., Kummert, M. (2012). Designing net-zero energy buildings for the future climate, not for the past. Building and Environment, 55, 150–158. [Google Scholar]
7. Ruth, N. E. H., Tian, X. J. (2014). Energy related CO2 emissions and the progress on CCS projects: A review. Renewable and Sustainable Energy Reviews, 31, 368–385. [Google Scholar]
8. Liu, Z., Ding, Y., Wang, F., Deng, Z. (2016). Thermal insulation material based on SiO2 aerogel. Construction and Building Materials, 122, 548–555. [Google Scholar]
9. Guan, P., Cheng, J., Zhu, S., Zhang, Y. (2023). Development and application of thermal insulation material for underground roadway with high ground temperature. Energy Sources, Part A: Recovery, Utilization, and Environmental Effects, 45(1), 1499–1510. [Google Scholar]
10. Ding, L., Zhao, J., Pan, Y., Guan, J., Jiang, J. et al. (2019). Insights into pyrolysis of nano-polystyrene particles: Thermochemical behaviors and kinetics analysis. Journal of Thermal Science, 28(4), 763–771. [Google Scholar]
11. Zhang, J., Chen, B., Yu, F. (2019). Preparation of EPS-based thermal insulation mortar with improved thermal and mechanical properties. Journal of Materials in Civil Engineering, 31(9), 04019183. [Google Scholar]
12. Niu, F., Jiang, H., Su, W., Jiang, W., He, J. (2021). Performance degradation of polymer material under freeze-thaw cycles: A case study of extruded polystyrene board. Polymer Testing, 96, 107067. [Google Scholar]
13. Wang, C., Huang, S., Chen, Q., Ji, X., Duan, K. (2023). Materials, preparation, performances and mechanism of polyurethane modified asphalt and its mixture: A systematic review. Journal of Road Engineering, 3(1), 16–34. [Google Scholar]
14. Lu, J., Zhang, H., Yang, H., Wang, J. (2022). Performance of resin-cement composite clad modified vitrified beads. Construction and Building Materials, 345, 128317. [Google Scholar]
15. Kotwica, Ł., Pichór, W., Kapeluszna, E., Różycka, A. (2017). Utilization of waste expanded perlite as new effective supplementary cementitious material. Journal of Cleaner Production, 140, 1344–1352. [Google Scholar]
16. Cheng, A., Lin, W. T., Huang, R. (2011). Application of rock wool waste in cement-based composites. Materials Design, 32(2), 636–642. [Google Scholar]
17. Mo, K. H., Lee, H. J., Liu, M. Y. J., Ling, T. C. (2018). Incorporation of expanded vermiculite lightweight aggregate in cement mortar. Construction and Building Materials, 179, 302–306. [Google Scholar]
18. Jia, G., Li, Z. (2021). Influence of the aerogel/expanded perlite composite as thermal insulation aggregate on the cement-based materials: Preparation, property, and microstructure. Construction and Building Materials, 273, 121728. [Google Scholar]
19. Chen, Y. X., Klima, K. M., Brouwers, H. J. H., Yu, Q. (2022). Effect of silica aerogel on thermal insulation and acoustic absorption of geopolymer foam composites: The role of aerogel particle size. Composites Part B: Engineering, 242, 110048. [Google Scholar]
20. Davraz, M., Koru, M., Akdağ, A. E., Kılınçarslan, Ş., Delikanlı, Y. E. et al. (2020). Investigating the use of raw perlite to produce monolithic thermal insulation material. Construction and Building Materials, 263, 120674. [Google Scholar]
21. Abdulmunem, A. R., Hussein, N. F., Samin, P. M., Sopian, K., Hussien, H. A. et al. (2023). Integration of recycled waste paper with phase change material in building enclosure. Journal of Energy Storage, 64, 107140. [Google Scholar]
22. Alsamaraie, A. R. A., Samin, P. M., Mazali, I. I., Jadallah, A. A., Sultan, K. F. (2023). Insulation materials based on recycled feather waste: A review. Tikrit Journal of Engineering Sciences, 30(1), 104–111. [Google Scholar]
23. Abdulmunem, A. R., Samin, P. M., Sopian, K., Hoseinzadeh, S., Al-Jaber, H. A. et al. (2022). Waste chicken feathers integrated with phase change materials as new inner insulation envelope for buildings. Journal of Energy Storage, 56, 106130. [Google Scholar]
24. Nanda, S., Berruti, F. (2021). A technical review of bioenergy and resource recovery from municipal solid waste. Journal of Hazardous Materials, 403, 123970. [Google Scholar] [PubMed]
25. Gupta, N., Yadav, K. K., Kumar, V. (2015). A review on current status of municipal solid waste management in India. Journal of Environmental Sciences, 37, 206–217. [Google Scholar]
26. Sekhohola-Dlamini, L., Tekere, M. (2020). Microbiology of municipal solid waste landfills: A review of microbial dynamics and ecological influences in waste bioprocessing. Biodegradation, 31(1–2), 1–21. [Google Scholar] [PubMed]
27. Asim, N., Emdadi, Z., Mohammad, M., Yarmo, M. A., Sopian, K. (2015). Agricultural solid wastes for green desiccant applications: An overview of research achievements, opportunities and perspectives. Journal of Cleaner Production, 91, 26–35. [Google Scholar]
28. Khedulkar, A. P., Pandit, B., Dang, V. D., Doong, R. (2023). Agricultural waste to real worth biochar as a sustainable material for supercapacitor. Science of the Total Environment, 869, 161441. [Google Scholar] [PubMed]
29. Chilakamarry, C. R., Mimi Sakinah, A. M., Zularisam, A. W., Sirohi, R., Khilji, I. A. et al. (2022). Advances in solid-state fermentation for bioconversion of agricultural wastes to value-added products: Opportunities and challenges. Bioresource Technology, 343, 126065. [Google Scholar] [PubMed]
30. Yang, S., Yao, X., Li, J., Wang, X., Zhang, C. et al. (2021). Preparation and properties of ready-to-use low-density foamed concrete derived from industrial solid wastes. Construction and Building Materials, 287, 122946. [Google Scholar]
31. Gu, J., Liu, X., Zhang, Z. (2023). Road base materials prepared by multi-industrial solid wastes in China: A review. Construction and Building Materials, 373, 130860. [Google Scholar]
32. Rada, E. C., Schiavon, M., Torretta, V. (2021). A regulatory strategy for the emission control of hexavalent chromium from waste-to-energy plants. Journal of Cleaner Production, 278, 123415. [Google Scholar]
33. Khan, M. N. N., Saha, A. K., Sarker, P. K. (2020). Reuse of waste glass as a supplementary binder and aggregate for sustainable cement-based construction materials: A review. Journal of Building Engineering, 28, 101052. [Google Scholar]
34. Harrison, E., Berenjian, A., Seifan, M. (2020). Recycling of waste glass as aggregate in cement-based materials. Environmental Science and Ecotechnology, 4, 100064. [Google Scholar] [PubMed]
35. Jiang, Y., Ling, T. C., Mo, K. H., Shi, C. (2019). A critical review of waste glass powder–Multiple roles of utilization in cement-based materials and construction products. Journal of Environmental Management, 242, 440–449. [Google Scholar] [PubMed]
36. Ez-zaki, H., El Gharbi, B., Diouri, A. (2018). Development of eco-friendly mortars incorporating glass and shell powders. Construction and Building Materials, 159, 198–204. [Google Scholar]
37. Flores-Alés, V., Alducin-Ochoa, J. M., Martín-del-Río, J. J., Torres-González, M., Jiménez-Bayarri, V. (2020). Physical-mechanical behaviour and transformations at high temperature in a cement mortar with waste glass as aggregate. Journal of Building Engineering, 29, 101158. [Google Scholar]
38. Yousefi, A., Tang, W., Khavarian, M., Fang, C., Wang, S. (2020). Thermal and mechanical properties of cement mortar composite containing recycled expanded glass aggregate and nano titanium dioxide. Applied Sciences, 10(7), 2246. [Google Scholar]
39. Cardinale, T., Arleo, G., Bernardo, F., Feo, A., De Fazio, P. (2017). Thermal and mechanical characterization of panels made by cement mortar and sheep’s wool fibres. Energy Procedia, 140, 159–169. [Google Scholar]
40. El Wazna, M., El Fatihi, M., El Bouari, A., Cherkaoui, O. (2017). Thermo physical characterization of sustainable insulation materials made from textile waste. Journal of Building Engineering, 12, 196–201. [Google Scholar]
41. Fiore, V., di Bella, G., Valenza, A. (2020). Effect of sheep wool fibers on thermal insulation and mechanical properties of cement-based composites. Journal of Natural Fibers, 17(10), 1532–1543. [Google Scholar]
42. Mandili, B., Taqi, M., El Bouari, A., Errouaiti, M. (2019). Experimental study of a new ecological building material for a thermal insulation based on waste paper and lime. Construction and Building Materials, 228, 117097. [Google Scholar]
43. Shibib, K. S. (2015). Effects of waste paper usage on thermal and mechanical properties of fired brick. Heat and Mass Transfer, 51(5), 685–690. [Google Scholar]
44. Mandili, B., Taqi, M., Chakir, H., Douzane, O., Errouaiti, M. (2020). Development of new construction material for thermal insulation of building based on aggregate of waste paper and cement. Heat and Mass Transfer, 56(6), 1753–1765. [Google Scholar]
45. Aigbomian, E. P., Fan, M. (2013). Development of Wood-Crete building materials from sawdust and waste paper. Construction and Building Materials, 40, 361–366. [Google Scholar]
46. Aksogan, O., Resatoglu, R., Binici, H. (2018). An environment friendly new insulation material involving waste newsprint papers reinforced by cane stalks. Journal of Building Engineering, 15, 33–40. [Google Scholar]
47. Bourguiba, A., Touati, K., Sebaibi, N., Boutouil, M., Khadraoui, F. (2020). Recycled duvets for building thermal insulation. Journal of Building Engineering, 31, 101378. [Google Scholar]
48. Ding, X., Wang, S., Dai, R., Chen, H., Shan, Z. (2022). Hydrogel beads derived from chrome leather scraps for the preparation of lightweight gypsum. Environmental Technology Innovation, 25, 102224. [Google Scholar]
49. Mariosi, F. R., Arcaro, S., Venturini, J., Camaratta, R., Machado Machado, F. et al. (2021). Eggshells as agro-industrial waste substitute for CaCO3 in glass foams: A study on obtaining lower thermal conductivity. International Journal of Applied Ceramic Technology, 18(3), 838–849. [Google Scholar]
50. Lacoste, C., El Hage, R., Bergeret, A., Corn, S., Lacroix, P. (2018). Sodium alginate adhesives as binders in wood fibers/textile waste fibers biocomposites for building insulation. Carbohydrate Polymers, 184, 1–8. [Google Scholar] [PubMed]
51. Valverde, I. C., Castilla, L. H., Nuñez, D. F., Rodriguez-Senín, E., de la Mano Ferreira, R. (2013). Development of new insulation panels based on textile recycled fibers. Waste and Biomass Valorization, 4(1), 139–146. [Google Scholar]
52. Jiang, D., Lv, S., Cui, S., Sun, S., Song, X. et al. (2020). Effect of thermal insulation components on physical and mechanical properties of plant fibre composite thermal insulation mortar. Journal of Materials Research and Technology, 9(6), 12996–13013. [Google Scholar]
53. Ji, L. Q., Zhang, C., Fang, J. Q. (2017). Economic analysis of converting of waste agricultural biomass into liquid fuel: A case study on a biofuel plant in China. Renewable and Sustainable Energy Reviews, 70, 224–229. [Google Scholar]
54. Wang, S., Li, H., Zou, S., Zhang, G. (2020). Experimental research on a feasible rice husk/geopolymer foam building insulation material. Energy and Buildings, 226, 110358. [Google Scholar]
55. Dou, Y., Bao, A., Sun, D., Niu, Z. (2021). Rapid preparation of a composite insulating block comprising waste expanded polystyrene (EPS) foam and rice straw. BioResources, 17(1), 699–713. [Google Scholar]
56. Laborel-Préneron, A., Magniont, C., Aubert, J. E. (2018). Characterization of barley straw, hemp shiv and corn cob as resources for bioaggregate based building materials. Waste and Biomass Valorization, 9(7), 1095–1112. [Google Scholar]
57. Liu, X., Wang, H., Fei, Z., Dai, L., Zhang, G. et al. (2022). Effect of slightly cadmium-enriched kenaf straw on the mechanical and thermal properties of cement mortar. European Journal of Environmental and Civil Engineering, 26(9), 4093–4111. [Google Scholar]
58. Karapinar, I. S. (2020). Improvement of polyurethane thermal insulation materials by using corn stalk. IOP Conference Series: Materials Science and Engineering, 737(1), 012077. [Google Scholar]
59. Shao, K., Du, Y., Zhou, F. (2021). Feasibility of using treated corn cob aggregates in cement mortars. Construction and Building Materials, 271, 121575. [Google Scholar]
60. Boumhaout, M., Boukhattem, L., Hamdi, H., Benhamou, B., Ait Nouh, F. (2017). Thermomechanical characterization of a bio-composite building material: Mortar reinforced with date palm fibers mesh. Construction and Building Materials, 135, 241–250. [Google Scholar]
61. Benmansour, N., Agoudjil, B., Gherabli, A., Kareche, A., Boudenne, A. (2014). Thermal and mechanical performance of natural mortar reinforced with date palm fibers for use as insulating materials in building. Energy and Buildings, 81, 98–104. [Google Scholar]
62. Benaniba, S., Driss, Z., Djendel, M., Raouache, E., Boubaaya, R. (2020). Thermo-mechanical characterization of a bio-composite mortar reinforced with date palm fiber. Journal of Engineered Fibers and Fabrics, 15, 155892502094823. [Google Scholar]
63. Zhao, W., Yang, R., Zhang, Y., Wu, L. (2012). Sustainable and practical utilization of feather keratin by an innovative physicochemical pretreatment: High density steam flash-explosion. Green Chemistry, 14(12), 3352–3360. [Google Scholar]
64. Dieckmann, E., Onsiong, R., Nagy, B., Sheldrick, L., Cheeseman, C. (2021). Valorization of waste feathers in the production of new thermal insulation materials. Waste and Biomass Valorization, 12(2), 1119–1131. [Google Scholar]
65. Bessa, J., Souza, J., Lopes, J. B., Sampaio, J., Mota, C. et al. (2017). Characterization of thermal and acoustic insulation of chicken feather reinforced composites. Procedia Engineering, 200, 472–479. [Google Scholar]
66. Fedorik, F., Zach, J., Lehto, M., Kymäläinen, H. R., Kuisma, R. et al. (2021). Hygrothermal properties of advanced bio-based insulation materials. Energy and Buildings, 253, 111528. [Google Scholar]
67. Gutierrez, O., Balart, R., Lascano, D., Quiles-Carrillo, L., Fages, E. et al. (2020). Development and characterization of environmentally friendly insulation materials for the building industry from olive pomace waste. Fibers and Polymers, 21(5), 1142–1151. [Google Scholar]
68. Cao, L., Shi, X., Liu, X., Wu, J. (2013). Laboratory study on the properties of plastering mortar modified by feather fibers. Science and Engineering of Composite Materials, 20(3), 293–299. [Google Scholar]
69. Horma, O., Charai, M., El Hassani, S., El Hammouti, A., Mezrhab, A. (2022). Thermo-physical and mechanical characterization of cement-based mortar incorporating spent tea. Journal of Building Engineering, 52, 104392. [Google Scholar]
70. Sheng, D. D. C. V., Ramegowda, N. S., Guna, V., Reddy, N. (2022). Groundnut shell and coir reinforced hybrid bio composites as alternative to gypsum ceiling tiles. Journal of Building Engineering, 57, 104892. [Google Scholar]
71. Guna, V., Ilangovan, M., Rather, M. H., Giridharan, B. V., Prajwal, B. et al. (2020). Groundnut shell/rice husk agro-waste reinforced polypropylene hybrid biocomposites. Journal of Building Engineering, 27, 100991. [Google Scholar]
72. Mehrzad, S., Taban, E., Soltani, P., Samaei, S. E., Khavanin, A. (2022). Sugarcane bagasse waste fibers as novel thermal insulation and sound-absorbing materials for application in sustainable buildings. Building and Environment, 211, 108753. [Google Scholar]
73. Panyakaew, S., Fotios, S. (2011). New thermal insulation boards made from coconut husk and bagasse. Energy and Buildings, 43(7), 1732–1739. [Google Scholar]
74. Liu, Y., Cao, Z., Wang, Y., Wang, D., Liu, J. (2021). Experimental study of hygro-thermal characteristics of novel cement-cork mortars. Construction and Building Materials, 271, 121901. [Google Scholar]
75. Philip, S., Rakendu, R. (2020). Thermal insulation materials based on water hyacinth for application in sustainable buildings. Materials Today: Proceedings, 33, 3803–3809. [Google Scholar]
76. Alshndah, Z., Becquart, F., Belayachi, N. (2023). Recycling of wheat straw aggregates of end-of-life vegetal concrete: Experimental investigation to develop a new building insulation material. Journal of Building Engineering, 76, 107199. [Google Scholar]
77. Binici, H., Aksogan, O., Demirhan, C. (2016). Mechanical, thermal and acoustical characterizations of an insulation composite made of bio-based materials. Sustainable Cities and Society, 20, 17–26. [Google Scholar]
78. Song, Q., Li, J., Zeng, X. (2015). Minimizing the increasing solid waste through zero waste strategy. Journal of Cleaner Production, 104, 199–210. [Google Scholar]
79. Duan, D., Wu, H., Wei, F., Song, H., Ma, Z. et al. (2023). Preparation, characterization, and rheological analysis of eco-friendly geopolymer grouting cementitious materials based on industrial solid wastes. Journal of Building Engineering, 78, 107451. [Google Scholar]
80. Williams, P. T. (2013). Pyrolysis of waste tyres: A review. Waste Management, 33(8), 1714–1728. [Google Scholar] [PubMed]
81. Azevedo, F., Pacheco-Torgal, F., Jesus, C., Barroso de Aguiar, J. L., Camões, A. F. (2012). Properties and durability of HPC with tyre rubber wastes. Construction and Building Materials, 34, 186–191. [Google Scholar]
82. Thomas, B. S., Gupta, R. C. (2016). A comprehensive review on the applications of waste tire rubber in cement concrete. Renewable and Sustainable Energy Reviews, 54, 1323–1333. [Google Scholar]
83. Kazmierczak, C. S., Schneider, S. D., Aguilera, O., Albert, C. C., Mancio, C. (2020). Rendering mortars with crumb rubber: Mechanical strength, thermal and fire properties and durability behaviour. Construction and Building Materials, 253, 119002. [Google Scholar]
84. Qin, G., Shen, Z., Yu, Y., Fan, L., Cao, H. et al. (2019). Effect of silicone rubber of a waste composite insulator on cement mortar properties. Materials, 12(17), 2796. [Google Scholar] [PubMed]
85. Na, O., Xi, Y. (2017). Mechanical and durability properties of insulation mortar with rubber powder from waste tires. Journal of Material Cycles and Waste Management, 19(2), 763–773. [Google Scholar]
86. Bostanci, L. (2020). Synergistic effect of a small amount of silica aerogel powder and scrap rubber addition on properties of alkali-activated slag mortars. Construction and Building Materials, 250, 118885. [Google Scholar]
87. Wongsa, A., Sata, V., Nematollahi, B., Sanjayan, J., Chindaprasirt, P. (2018). Mechanical and thermal properties of lightweight geopolymer mortar incorporating crumb rubber. Journal of Cleaner Production, 195, 1069–1080. [Google Scholar]
88. Torkittikul, P., Nochaiya, T., Wongkeo, W., Chaipanich, A. (2017). Utilization of coal bottom ash to improve thermal insulation of construction material. Journal of Material Cycles and Waste Management, 19(1), 305–317. [Google Scholar]
89. Wang, S., Chen, M., Lu, L., Zhao, P., Gong, C. (2016). Investigation of the adaptability of paper sludge with wood fiber in cement-based insulation mortar. BioResources, 11(4), 10419–10432. [Google Scholar]
90. Lee, J. G., Yeo, W. H. (2018). Characteristics of lightweight and thermal insulation of bituminous coal bottom ash. Journal of the Korea Organic Resources Recycling Association, 26(1), 39–45. [Google Scholar]
91. Gautam, B., Huang, M. R., Lin, C. C., Chang, C. C., Chen, J. T. (2023). A viable approach for polymer upcycling of polystyrene (styrofoam) wastes to produce high value predetermined organic compounds. Polymer Degradation and Stability, 217, 110528. [Google Scholar]
92. Khoukhi, M. (2018). The combined effect of heat and moisture transfer dependent thermal conductivity of polystyrene insulation material: Impact on building energy performance. Energy and Buildings, 169, 228–235. [Google Scholar]
93. Záleská, M., Pavlíková, M., Jankovský, O., Lojka, M., Pivák, A. et al. (2018). Experimental analysis of MOC composite with a waste-expanded polypropylene-based aggregate. Materials, 11(6), 931. [Google Scholar]
94. Ferrández, D., Álvarez, M., Saiz, P., Zaragoza, A. (2022). Experimental study with plaster mortars made with recycled aggregate and thermal insulation residues for application in building. Sustainability, 14(4), 2386. [Google Scholar]
95. Koksal, F., Mutluay, E., Gencel, O. (2020). Characteristics of isolation mortars produced with expanded vermiculite and waste expanded polystyrene. Construction and Building Materials, 236, 117789. [Google Scholar]
96. Li, R., Zhou, Y., Li, C., Li, S., Huang, Z. (2019). Recycling of industrial waste iron tailings in porous bricks with low thermal conductivity. Construction and Building Materials, 213, 43–50. [Google Scholar]
97. Trajković, D., Jordeva, S., Tomovska, E., Zafirova, K. (2017). Polyester apparel cutting waste as insulation material. The Journal of the Textile Institute, 108(7), 1238–1245. [Google Scholar]
98. Li, X., Pan, M., Tao, M., Liu, W., Gao, Z. et al. (2022). Preparation of high closed porosity foamed ceramics from coal gangue waste for thermal insulation applications. Ceramics International, 48(24), 37055–37063. [Google Scholar]
99. Tang, Z., Liang, J., Jiang, W., Liu, J., Jiang, F. et al. (2020). Preparation of high strength foam ceramics from sand shale and steel slag. Ceramics International, 46(7), 9256–9262. [Google Scholar]
100. Zhu, L., Li, S., Li, Y., Xu, N. (2018). Novel applications of waste ceramics on the fabrication of foamed materials for exterior building walls insulation. Construction and Building Materials, 180, 291–297. [Google Scholar]
101. Gao, H., Liu, H., Liao, L., Mei, L., Shuai, P. et al. (2019). A novel inorganic thermal insulation material utilizing perlite tailings. Energy and Buildings, 190, 25–33. [Google Scholar]
102. Martínez-García, C., González-Fonteboa, B., Carro-López, D., Pérez-Ordóñez, J. L. (2020). Mussel shells: A canning industry by-product converted into a bio-based insulation material. Journal of Cleaner Production, 269, 122343. [Google Scholar]
103. Reynoso, L. E., Carrizo Romero, Á. B., Viegas, G. M., San Juan, G. A. (2021). Characterization of an alternative thermal insulation material using recycled expanded polystyrene. Construction and Building Materials, 301, 124058. [Google Scholar]
104. Cheng, J., Ran, D., Shu, C. M., Zhu, S., Zhang, Y. (2023). Resource utilisation of waste TPU: Reform and modification for improving a bentonite-based thermal insulation material’s performance. Inorganic Chemistry Communications, 154, 110866. [Google Scholar]
105. Shi, G., Liu, T., Li, G., Wang, Z. (2021). A novel thermal insulation composite fabricated with industrial solid wastes and expanded polystyrene beads by compression method. Journal of Cleaner Production, 279, 123420. [Google Scholar]
106. Liu, Y., Kumar, D., Lim, K. H., Lai, Y. L., Hu, Z. et al. (2023). Efficient utilization of municipal solid waste incinerator bottom ash for autoclaved aerated concrete formulation. Journal of Building Engineering, 71, 106463. [Google Scholar]
107. Benallel, A., Tilioua, A., Ettakni, M., Ouakarrouch, M., Garoum, M. et al. (2021). Design and thermophysical characterization of new thermal insulation panels based on cardboard waste and vegetable fibers. Sustainable Energy Technologies and Assessments, 48, 101639. [Google Scholar]
108. Gounni, A., Mabrouk, M. T., El Wazna, M., Kheiri, A., El Alami, M. et al. (2019). Thermal and economic evaluation of new insulation materials for building envelope based on textile waste. Applied Thermal Engineering, 149, 475–483. [Google Scholar]
109. Yang, J., Wu, H., Xu, X., Huang, G., Xu, T. et al. (2019). Numerical and experimental study on the thermal performance of aerogel insulating panels for building energy efficiency. Renewable Energy, 138, 445–457. [Google Scholar]
Cite This Article
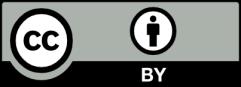
This work is licensed under a Creative Commons Attribution 4.0 International License , which permits unrestricted use, distribution, and reproduction in any medium, provided the original work is properly cited.