Open Access
ARTICLE
Multifunctional Films Based on Wheat Gluten and Microencapsulated Thyme Essential Oil
1 Instituto de Investigaciones en Ciencia y Tecnología de Materiales, Facultad de Ingeniería, Universidad Nacional de Mar del Plata-Consejo Nacional de Investigaciones Científicas y Técnicas, Mar del Plata, 7600, Argentina
2 Grupo de Investigación en Ingeniería en Alimentos, Facultad de Ingeniería, Universidad Nacional de Mar del Plata-Consejo Nacional de Investigaciones Científicas y Técnicas, Mar del Plata, 7600, Argentina
* Corresponding Author: María Roberta Ansorena. Email:
(This article belongs to the Special Issue: Recent Advances on Renewable Materials)
Journal of Renewable Materials 2024, 12(12), 2049-2068. https://doi.org/10.32604/jrm.2024.055151
Received 19 June 2024; Accepted 01 November 2024; Issue published 20 December 2024
Abstract
In this work, active and edible films for food packaging were obtained by intensive mixing and compression molding of the wheat gluten (WG) glycerol-plasticized paste. Thyme essential oil (TEO, 10 and 15 wt.%) was incorporated as the active component microencapsulated in β-cyclodextrins (β-CD) and included directly into the biopolymer matrix for comparison. It was found that films incorporating microencapsulated TEO are more soluble in water (total soluble matter (dry method) of about 33% and 36.6% vs. 22.4% and 18.6%, for films containing 10% and 15% TEO, respectively) but less rigid than those obtained with free oil (elastic tensile moduli of 6.9 and 3.1 vs. 9.9 and 6.8 MPa, for films containing 10% and 15% TEO, respectively), although the water vapor permeability of the former is lower (4.95 10−9 and 6.29 10−9 vs. 8.85 10−9 and 11.13 10−9 g/Pa*s*m, for films containing 10% and 15% TEO, respectively). Active films containing both free and encapsulated TEO inhibited gram (+) as well as gram (−) bacteria and exhibited enlarged antioxidant properties, with the latter presenting slightly better performance. In addition, encapsulation slowed the release rate of TEO from the films, allowing the bioactive to remain active in the film for much longer. Therefore, the present work revealed that films prepared from TEO/β-CD microcapsules included in compression-molded wheat gluten matrices showed great potential to be used as an active food packaging.Graphic Abstract
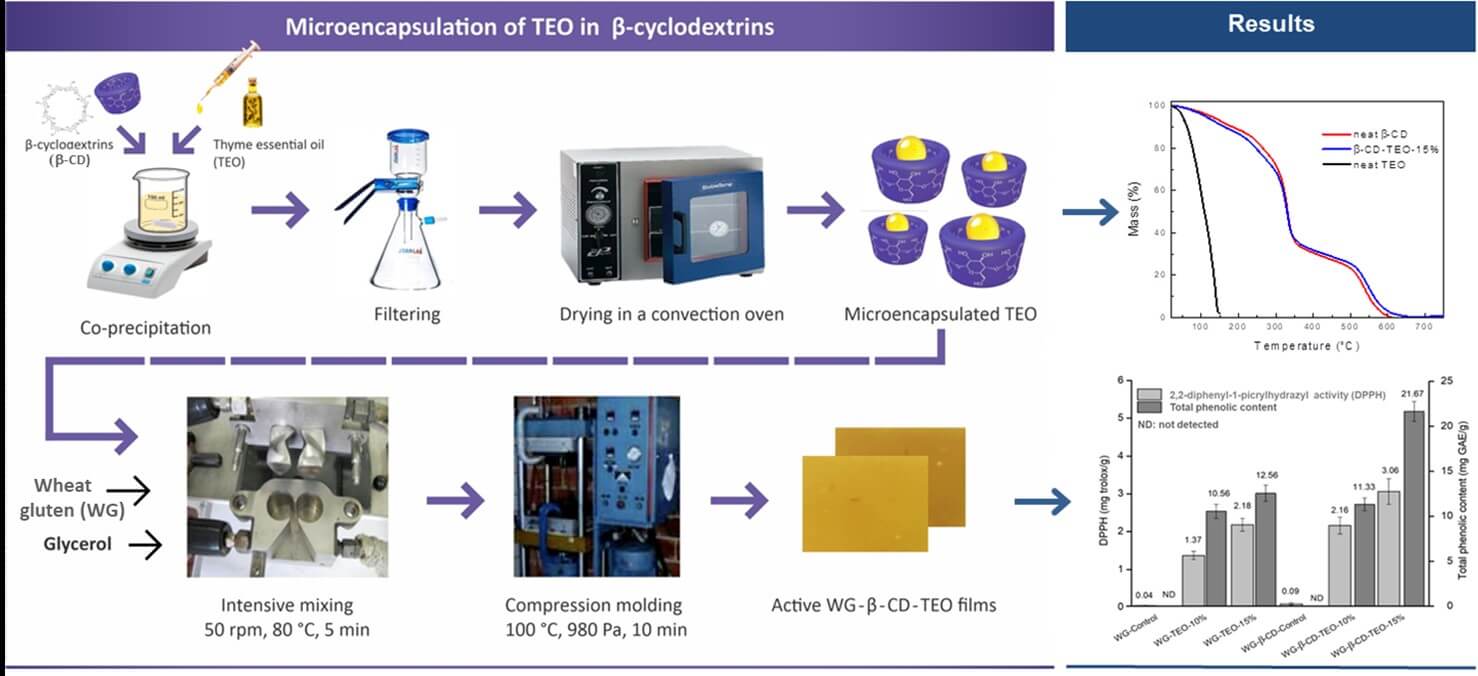
Keywords
Nomenclature
WG | Wheat gluten |
TEO | Thyme essential oil |
β-CD | β-cyclodextrin |
Eos | Essential oils |
GRAS | Generally Recognized as Safe |
CDs | Cyclodextrins |
IC | Inclusion complexes |
EE | Encapsulation efficiency |
RH | Relative humidity |
WVP | Water vapor permeability |
P | Vapor pressures (Pa) |
A | Exposed area (m2) |
Y | Thickness (m) |
ΔW | Mass (g) |
Δt | Time (s) |
TSM | Total soluble matter |
DRY TSM | Total soluble matter (dry method) |
WET TSM | Total soluble matter (wet method) |
m0 | Initial dry matter |
mf | Undissolved dry matter |
M∞ | Equilibrium moisture content |
Mf | Weight at equilibrium |
Mi | Initial dry weight |
σm | Maximum strength |
εb | Elongation at break |
E | Elastic modulus |
DMA | Dynamic Mechanical Analysis |
G′ | Storage modulus |
G″ | Loss modulus |
ATCC | American Type Culture Collection |
BHI | Brain-heart-infusion |
FCR | Folin-Ciocalteu reagent |
DPPH | 2,2-diphenyl-1-picrylhydrazyl |
TPC | Total phenolic content |
GAE | Gallic acid equivalents |
mt | Amount of the active agent released from the film at time t |
m∞ | Amount of the active agent released at equilibrium |
D | Diffusion coefficient |
l | Film thickness |
k | Release rate constant |
ANOVA | Analysis of variance |
TGA | Thermogravimetric analysis |
DTG | Differential thermogravimetric |
SEM | Scanning electron microscopy |
FE-SEM | Field emission scanning electron microscope |
PLA | Polylactic acid |
NC | Nominal concentration |
APC | After processing concentration |
Edible films are among the emerging technologies currently being used to improve the quality and shelf life of food [1–4]. They are generally obtained from renewable ecological resources and have been demonstrated to be economical and effective [5,6]. Furthermore, they can incorporate food additives such as antimicrobials and antioxidants, providing highly localized functional effects, and, in some cases, they can even improve organoleptic properties [1,5]. Edible matrices are made up of carbohydrates or proteins, such as starch, pectins, gums, cellulose and derivatives, chitosan, etc. [7]. Moreover, consumer concerns about food safety and quality are driving the advancement of active packaging as novel tools that work beyond inert/passive product containment. Active packaging is an extension of the protection function of a package and can therefore ameliorate food storage due to its ability to act as a barrier to water (i.e., preventing food dehydration and/or keeping it from getting moistened), light (i.e., reducing lipid oxidation), oxygen, and its ability to incorporate specific functional properties [3,8,9]. Thus, the latest research on active packaging consists of providing the packaging with antibacterial/antioxidant function by adding bioactive agents to it [9]. In this regard, several methods for controlled or slow release of active compounds have been reported in the literature, for instance, incorporation of the active ingredient directly into the polymeric matrix, multilayer coatings applied outside or inside the packaging, microencapsulation of active compounds incorporated into the polymeric matrix, etc. [10].
Wheat gluten [11,12] is a by-product of wheat starch production. Gluten comprises several complex proteins, in contents as high as 72%–85%, which can be classified into two classes in agreement with their solubility in aqueous alcohols: insoluble glutenins and soluble gliadins. In addition, due to its great availability and low price, wheat gluten is widely used in biological and food applications, as well as in pharmaceutical industries [13,14]. Furthermore, mixtures of wheat gluten and plasticizers can be processed as thermoplastics to give many types of shaped products and can therefore be considered as an alternative to synthetic plastics for different applications [12,15]. Most efforts in finding applications for wheat gluten have focused on the development of environmentally friendly packaging films for food storage [12,16], mainly due to its exceptional carbon dioxide and oxygen barrier properties, along with its low water vapor barrier characteristics [12]. Thus, wheat gluten films could be applied as edible films on foods that naturally contain gluten, such as pastry and bakery products, to slow down the water and oxygen transfer phenomena responsible for the decrease in food quality. The absorption of water from the surrounding environment, as well as its migration from the food itself, can increase the rate of microbial growth, and chemical reactions leading to a reduced shelf life of the product. Oxygen transfer, on the other hand, could accelerate the oxidation of pigments and lipids present in foods and trigger undesirable color changes as well as unpleasant flavors [16].
Essential oils (EOs) obtained from plants are interesting native antimicrobial agents since they are rich in phenolic compounds and small terpenoids that exhibit antimicrobial activities [1–3,8,17]. Nevertheless, their use in food packaging is restricted due to their intense flavor, high volatility, and high content of unstable components [9]. In addition, their use as preserving agents is often restricted due to their intense flavor, which can exceed acceptable organoleptic levels to provide effective antimicrobial properties, although most EOs are registered as Generally Recognized as Safe (GRAS) [2,4,8,9]. Amongst the EOs, the antimicrobial and antioxidant properties of thyme essential oil (TEO) have been recognized [2,8,18] and ascribed to the synergistic effect of its main components, thymol and carvacrol, resulting in TEO being more effective than pure thymol [19]. Encapsulation methodologies, specially designed to shield aromas and flavors from external interactions and provide a controlled release of the bioactive component to the packaged food, are quite convenient to incorporate EOs into food packaging [2,17]. In this line, Yammine et al. [20] summarized recent advances in micro/nanoencapsulation, considering them as promising tools to improve the functionalities of essential oil formulations, while addressing their different limitations Cyclodextrins (CDs) are natural, nontoxic trapping macromolecules made up of torus-shaped cyclic oligosaccharides comprising glucopyranose units linked by α-1,4 bonds [10,21,22] and consisting of an hydrophobic internal cavity with a hydrophilic outer wall [22,23]. CDs have demonstrated an excellent ability to entrap guest molecules such as food additives, drugs, and several bioactive compounds, by forming guest-host inclusion complexes (IC) through hydrogen bonds, hydrophobic or van der Waals interactions [10,22]. Microencapsulation in β-cyclodextrins has been revealed as one of the most efficient methodologies to protect active ingredients against evaporation, oxidation and heat degradation, increase solubility, and mask unwanted odors or flavors [24]. Moreover, the encapsulation of EOs (or their main bioactive components), specially in β-CDs, gave rise to inclusion complexes (IC) that confirmed their ability to maintain the quality and extend the shelf life of packaged meat [25], mushrooms [26], and fruits [27].
An alternative to the usual approach in the design of active edible films is to add the bioactive compound, previously retained in β-CD, to a polymeric matrix, in order to improve the control of molecule delivery [25]. However, most research on active biodegradable polymers incorporated with encapsulated bioactives focuses on casting as a manufacturing method [28–30], so there is little research on obtaining these active edible films using thermoplastic processes such as extrusion or compression molding [25]. In fact, most of the scientific studies reporting on the possibility of using industrial thermal processing techniques (extrusion, injection molding, etc.) on biopolymer/bio-based polymer matrices focus on the unusual carbohydrates whose molecular interactions between chains can be disrupted via plasticization, leading to enhanced polymer flexibility and processability, such as starch [31,32] or eventually agar [33]. There are far fewer works on thermoplastic processing of protein-based matrices and when available, they are mainly combined with other polymers [34]. Additionally, there is still limited knowledge regarding the release rate of the active component loaded in microcapsules when added to polymeric matrices processed by techniques involving heat and pressure such as compression molding.
To our knowledge, this is the first study on wheat gluten active films incorporated with TEO microencapsulated into β-CD, produced by thermoplastic processing. This study demonstrated that the application range of wheat gluten and thyme essential oil in the field of active packaging can be expanded.
Food grade wheat gluten (WG), moisture content = 9.08 ± 0.10% (determined by drying WG in a convection oven at 120°C until constant weight was achieved), technical grade glycerol and thyme essential oil (TEO), extracted from the plant Thymus vulgaris, were purchased in local shops (Mar del Plata/Buenos Aires, Argentina) [18]. β-cyclodextrin (β-CD) (Cavamax W7 food grade) was provided by Wacker Biochem, Eddyville, IA, USA.
2.2.1 Microencapsulation of TEO in β-cyclodextrins
TEO:β-CD microcapsules were obtained by the co-precipitation methodology, as described in [35], which was applied until reaching a TEO:β-CD ratio of 8:92 (%, w/w). This ratio was selected based on preliminary studies in which different TEO to β-CD weight ratios were tested, because it was the one that presented the highest encapsulation efficiency (EE). The obtained suspension was filtered and the TEO:β-CD inclusion complexes were then dried in a convection oven (50°C, 24 h) and finally stored in a desiccator containing silica-gel at 25°C.
Wheat gluten plasticized films were prepared as described in previous papers [18,36], using 20% weight glycerol (based on total mass). The active films were prepared in the same way, but including into the paste free [18] or microencapsulated TEO, leading to films containing 10% and 15% by weight of thyme oil (labeled as WG-TEO-% and WG-β-CD-TEO-%, respectively), where % accounts for the weight percentage of TEO incorporated into the films. Neat WG films (WG-Control) and control films containing β-CD without thyme essential oil were also prepared and labeled as WG-β-CD-control.
2.2.3 Characterization Techniques
Encapsulation Efficiency (EE) and Particle Size of TEO:β-CD Microcapsules: the encapsulation efficiency of TEO:β-CD microcapsules was evaluated according to the methodology reported in [37,38], with slight modifications. 5 mg TEO:β-CD were dispersed in 5 mL of acetonitrile/water mixture (95 g/100 mL) and stirred for 48 h to ensure that all the entrapped active compound was released into the solution. Then, the remaining β-CD was removed from the solution by centrifugation at 3200 g for 15 min. The amount of the active compound (thymol, main TEO constituent of the thyme essential oil) entrapped in the TEO:β-CD microcapsules was determined spectrophotometrically at 274 nm in a UV–Vis spectrophotometer (UV-4100, Shimadzu Corporation, Tokyo, Japan) and by high performance liquid chromatography (HPLC, Shimadzu, Japan), as described in [18]. EE was calculated according to Eq. (1):
EE=Amount of entrapped active compoundNominal active compound amount×100 (1)
where “Amount of entrapped active compound” is the quantity of active compound included in the inclusion complex particles, and “Nominal active compound amount” indicates the quantity of active compound initially used to obtain the particles.
A nano particle analyzer (Nanotrac Wave II/Zeta, Microtrac, York, PA, USA) was used to determine the size of TEO:β-CD microcapsules. The measurements were performed using a 4:1 particle:distilled water ratio that was placed into plastic cuvettes with a path length of 1 cm. Data were reported as the average of the three readings.
Thermogravimetric Analysis (TGA): tests were conducted on a Shimadzu (TGA-50) thermogravimetric analyzer. Measurements were performed on samples of 4 to 10 mg, at a heating rate of 10°C min−1, from 35°C to 750°C, and in oxidizing atmosphere (air). Both, percentage of initial mass vs. temperature/time (TGA) and differential thermogravimetric (DTG) (i.e., the first derivative of the weight loss with respect to temperature/time) curves were recorded for each sample.
Microstructure: morphology of films was analyzed utilizing a field emission scanning electron microscope (FE-SEM, SUPRA 40, ZEISS) under a 15 kV accelerating voltage. As usual, films were cryo-fractured in liquid nitrogen and their cross sections coated with gold prior observation.
Water Vapor Permeability (WVP): film samples were pre-conditioned for at least 3 days in an environmental chamber kept at room temperature (25 ± 2°C) and 65% relative humidity (RH). Then, they were sealed in acrylic permeation cups (5 cm diameter), which contained distilled water inside (100% RH) and located into the chamber. The cups were periodically weighed until reaching constant weight. The WVP of the films, [g.m/(Pa.s.m2)], was calculated as:
WVP=ΔW×y×[A×Δt×(p2−p1)]−1 (2)
where A and y are the exposed area (m2, calculated from the cup diameter) and the thickness (m, measured with a caliper) of the film sample, respectively, while p2 and p1 are the vapor pressures (Pa) inside and outside the cup that are calculated based on the cup and chamber relative humidities. In addition, ΔW is the mass (g) of water gained/lost by the assembly (cup + film + distilled water) and Δt is the elapsed time for mass change.
Total Soluble Matter (TSM): these tests involve determining the percentage of a dry sample that is solubilized in distilled water after 24 h of immersion. Two different approaches for this measurement, named DRY and WET, were used and compared. In both cases, dried film pieces were placed in 50 mL vessels containing 30 mL distilled water and traces of sodium azide (to inhibit microbial growth), and maintained in a chamber for 24 h at 25°C, with intermittent shaking. The main difference between these methods is that in the DRY one, the specimens were previously dried in a convective oven at 105°C for 24 h and then weighed (precision of ± 0.0001 g), to evaluate the initial dry matter (m0), while in the WET one m0 was determined from different samples to prevent heating test specimens before being stored in water. In both cases, the dry matter that did not dissolve during the test (mf) was determined from the specimens removed from the vessels that were previously rinsed gently with distilled water and dried in the convective oven (105°C for 24 h). TSM was finally calculated according to Eq. (3). Additional details regarding this methodology can be found in [36].
TSM(%)=m0−mfm0×100 (3)
Equilibrium Moisture Sorption: pieces of vacuum dried films (at 40°C until reaching constant weight) were placed inside an environmental chamber (25 ± 2°C, 95% RH). At regular time intervals, specimens were taken out of the chamber and weighed with a precision of ± 0.0001 g. Their equilibrium moisture content (M∞), expressed as a percentage on a dry basis, was calculated according to Eq. (4):
M∞(%)=Mf−MiMi×100 (4)
where Mf and Mi are, respectively, the weight of the specimen at equilibrium and its initial dry weight (g).
Opacity: it was evaluated by placing rectangular strips of films directly into the cell of an ultraviolet-visible (UV-Vis) spectrophotometer (Shimadzu 1601 PC, Tokyo, Japan). Opacity was calculated as the area under the absorption spectra from 400 to 800 nm (absorbance units) divided by sample thickness (mm). Three values were taken for each sample and the results are presented as mean value ± standard deviation.
Tensile Properties: films specimens cut from the molded plates according to ASTM D1708-93 (ASTM, 1993) were conditioned for 96 h at 23°C and 50 ± 5% RH before performing mechanical tests and then tested at room temperature (25 ± 2°C) and 5 mm/min crosshead speed in an Instron 8501 universal testing machine. Elastic modulus (E), maximum strength (σm), and elongation at break (εb) were calculated as described in ASTM D638-94b (ASTM, 1994).
Dynamic Mechanical Analysis (DMA): dynamic mechanical measurements were performed as described in [36]. Both the storage (G′) and loss modulus (G″) were determined from the test.
Antimicrobial Activity: it was assessed by the agar diffusion methodology, evaluating the sensitivity of Gram (+) bacteria (Listeria innocua (CIP 8011) and Staphylococcus aureus (ATCC 25923)) and Gram (−) bacteria (Escherichia coli (ATCC 25922) and Pseudomona aeruginosa (ATCC 27853)) to active and control films (neat WG films and WG films containing β-CD without thyme essential oil), as described in Ansorena et al. [18]. Films discs were placed on brain-heart-infusion (BHI) agar plates previously inoculated with native microflora and bacterial suspensions using a sterile cotton swab, and then incubated at 37°C for 24 h. The total diameter of the growth inhibition zones (mm) was used as a measure of the antimicrobial activity. Measurements were taken in triplicate for each sample.
Antioxidant Capacity and Total Phenolic Content: pieces of films (0.5 g) were immersed in 10 mL of pure methanol that were submitted to constant shaking for 24 h at room temperature and under dark conditions. Subsequently, the flasks were sonicated (Ultrasound chamber PS-30A, RoHs, Jiangsu, China) for 20 min. These extracts were further used to determine antioxidant capacity and total polyphenol content, with the later determined spectrophotometrically using the Folin-Ciocalteu reagent (FCR), and the antioxidant properties evaluated as the scavenging ability of 2,2-diphenyl-1-picrylhydrazyl (DPPH) free radicals at 517 nm [35]. Antioxidant capacity was informed in mg trolox/g and total phenolic content (TPC) results were expressed as mg gallic acid equivalents (GAE)/g.
In Vitro Controlled Release Studies: the release kinetics of free and microencapsulated TEO from wheat gluten films was determined using the migration diffusion of thymol (main TEO constituent) from the biopolymer into a 50% (v/v) ethanol solution, which can be regarded as a simulant for oil-in-water emulsions and alcoholic beverages [39]. The concentration of thymol released into food simulants was periodically determined by high pressure liquid chromatography (HPLC, Shimadzu, Japan). About 1.5 g active films were placed in closed containers with 250 mL of the simulant and agitated at 20°C and 100 rpm on an orbital shaker (KS 130 Basic, IKA, Campinas, Brazil). Aliquots of each solution were taken out over 144 h at different time intervals to determine the kinetics of thymol release.
According to Cran et al. [40] and Chen et al. [25], the release process of an active component from a film can be completely described by the diffusion models. The release of the bioactive agent from the film to the food simulant is typically analyzed according to the time elapsed, using either the short-term or the long-term migration equations [25,40]. The beginning of the migratory process, up to mt/m∞ < 0.6, can be adequately described by the short-term equation:
mtm∞=4×(D×tπ×l2)1/2 (5)
being m∞ and mt the quantity of the active agent released from the specimen at equilibrium and at any time t, respectively, while D represents the diffusion coefficient and l is the film thickness. For the long-term migration (mt/m∞ > 0.6), Eq. (6) can be used [40]:
ln(1−mtm∞)=ln(8π2)−k×t (6)
being k the release rate constant.
Data were statistically analyzed as reported in previous papers [18,36] (ANOVA methodology, least significance difference of p < 0.05).
3.1 Encapsulation Efficiency and Particle Size of TEO:β-CD Microcapsules
Encapsulation efficiency (EE) indicates the amount of active component entrapped in the encapsulation systems (i.e., load capacity) and is therefore directly related to the controlled release characteristics of the core material [28]. The EE value of TEO:β-CD microcapsules (Eq. (1)), was 72.22 ± 2.82%, which indicated the effective entrapment of thyme essential oil by the β-CD carrier. This value was comparable with those obtained in other studies using the same method of inclusion, such as 73.5% by loading lemongrass [28], 71.66% by embedding TEO [41] and 77.74% by loading clove into β-CD [38]. Besides, Tao and co-workers [19] measured an encapsulation efficiency of 74.57% for TEO in β-CD microcapsules obtained by freeze-drying.
The average diameter of TEO:β-CD microcapsules was found to be 7.3 ± 0.2 µm. These values are in the same range as those obtained by Tao and co-workers [19] and Chen and co-workers [28] by loading TEO and lemongrass into β-CD.
3.2 Appearance and Physicochemical and Mechanical Characterization of Films
Scheme 1 summarizes the methodology applied to obtain active and control WG based films. From the image, it can be noticed that films are yellowish/light brown, optically opaque, and do not present notable visual differences that can be attributed to the incorporation of free or microencapsulated TEO.
Scheme 1: Scheme of the process for obtaining active films
Fig. 1 presents the thermogravimetric curves obtained for pure β-cyclodextrins, neat and microencapsulated TEO. The great volatility of the thyme essential oil can be recognized by the sudden loss of mass that occurs as the test temperature increases, resulting in the entire evaporation/degradation of the TEO at 150°C. The neat β-CDs show several stages of mass loss or decomposition, which are evidenced by a change in the slope of the TGA vs. temperature curve or, more clearly, as downward peaks in the DTG curve. The early one occurs in the temperature range of 50°C to 190°C and shows a relatively low mass loss of 10.4%, mainly attributed to the evaporation of water from the inclusion complexes, as indicated in related papers [42]. The major loss of mass was traced in the degradation stages following the loss of adsorbed water: 12.6% mass loss in the second stage (DTG peak between 190°C and 280°C), 46.5% mass loss in the third (280°C to 400°C, from DTG peak), and fourth stage (400°C to 600°C), in which the rest of the mass is lost, leaving almost no carbonaceous residue. The mass loss in these stages was attributed to the breakdown of the eight-membered cyclodextrin structure, as reported by Ahmed et al. [42]. On the other hand, the microencapsulated oil presents a mass loss pattern very similar to that of the β-CDs, clearly indicating that the encapsulated TEO degrades at a higher temperature and slower rate than neat TEO, which confirms the molecular encapsulation of the essential oil inside the β-CD cavity (i.e., complexation between essential oil and CDs), after the encapsulation process. Furthermore, the mass loss in the first (up to 190°C) and second (up to 280°C) stages of the β-CDs-TEO-15% sample are 12.3% and 14.2%, respectively, that is, higher than the mass lost by the neat β-CDs in the same steps. However, for the following heating steps this behavior reversed, showing in the third stage a 41.6% mass loss, lower than the 46.5% exhibited by the neat β-CDs, and leaving a low but noticeable amount of carbonaceous residue (1.5% at 620°C) in the last stage, indicating that the encapsulated β-CD retained higher masses compared to the neat β-CD powder [42]. This corroborates the usefulness of microencapsulation in preserving the volatile active principles of TEO against agents such as heat.
Figure 1: TGA and DTG thermograms of pure β-cyclodextrins, neat and microencapsulated TEO
The morphology of the films incorporating free and encapsulated TEO was evaluated by scanning electron microscopy (SEM). As shown in Fig. 2, the fractured surfaces of films containing no or free TEO are smooth, neat and compact, and do not exhibit evidence of phase separation, which could indicate that free TEO is highly compatible and miscible with wheat gluten plasticized matrices. However, sample preparation for SEM observation, which involves a vacuum stage for covering the sample with a conductive metal, should also be considered before drawing conclusions. In fact, the free TEO incorporated into the wheat gluten films would probably evaporate during this stage and this would be the reason why the fracture surface of WG-Control (Fig. 2a) and those containing free essential oil (Fig. 2c,d) are so similar. On the other hand, the encapsulation into the β-CD should protect the TEO from evaporation during sample preparation for SEM observation. Although this statement cannot be verified from the SEM images, it is clear that the morphology of the fractured surfaces of these samples is different than the previous ones, which can be attributed to the presence of β-CD. In fact, films carrying β-CD (control) exhibit an irregular and rough fracture surface, in which the presence of relatively large aggregates embedded in the polymeric matrix stands out. These inclusions are attributed to a certain degree of β-CD aggregation that took place during the film formation process.
Figure 2: SEM micrographs (5000×) of the cross-section of wheat gluten films incorporating free or microencapsulated TEO: WG-Control (a), WG-β-CD-control (b), WG-TEO-10% (c), WG-TEO-15% (d), WG-β-CD-TEO-10% (e), WG-β-CD-TEO-15% (f)
On the other hand, films containing β-CD-TEO (Fig. 2e,f) also exhibit more heterogeneous and uneven fracture surfaces than those corresponding to pure wheat gluten or films incorporated with free TEO (Fig. 2a–c). Even when no large agglomerates are noticed in the WG-β-CD-TEO-10% film, its surface exhibits a heterogeneous and rough morphology, evidenced by the presence of ridges and valleys that could have originated during the brittle fracture of the film, at points where β-CD inclusions diverted crack propagation toward an easier fracture path. Furthermore, the SEM image of the WG-β-CD-TEO-15% film also evidences that for higher loadings, a certain degree of β-CD aggregation occurred and therefore Fig. 2f combines the features of WG-β-CD-TEO-10% and WG-β-CD-control films, albeit with slightly smaller aggregates than the latter. This decrease in aggregate size can be attributed to the methodology used to prepare the TEO-β-CD complex, which involves dissolution, inclusion and co-precipitation steps. Similar trends were found in related papers [43–45].
Table 1 presents the equilibrium moisture content, the total soluble mass values obtained from two different methodologies (i.e., WET and DRY) and the water vapor permeability of the different wheat gluten films. The equilibrium moisture content decreases from about 46% for the glycerol plasticized wheat gluten (WG-Control) film to around 40% for the active films (i.e., samples containing thyme oil in any form), which can be ascribed to the slightly more hydrophobic nature of the TEO compared to plasticized WG, although this property does not show a clear trend with respect to thyme essential oil concentration. On the other hand, it is also clear that the incorporation of β-CD into the WG matrix also affects the equilibrium moisture content, since the WG-β-CD-control sample exhibits the lowest value of the entire series, while it does not improve the performance of the TEO (i.e., β-CDs do not act by enhancing the reduction of adsorbed moisture when the oil is microencapsulated). Regarding total soluble matter, when taking into account the standard deviation of the WET TSM results, it becomes evident that the content of free thyme oil almost does not affect the total soluble mass of the corresponding films when TSM is determined from samples not previously dried. Otherwise, adding β-CDs to the protein matrix increases the solubility of the resulting films largely when the TEO is not microencapsulated into them. The microencapsulation of thyme oil leads to a reduction in this parameter, but the active films still remain more soluble than the WG-Control sample. Evidently, the amount of active ingredient, the interaction developed among film components, and the consequential film microstructure, affect the solubility of the resulting films, as indicated in related works [43]. The values of the total soluble mass determined from dried films (DRY TSM) are smaller than those corresponding to the wet samples in all cases, supporting previous observations indicating that increased cross-linking of gluten proteins takes place during drying at 105°C [18,36]. However, this effect is more noticeable for the films without oil (control films) or containing free oil (reductions ranging from 0.54 to 0.66) than in those containing β-CDs (reductions ranging from 0.75 to 0.87), indicating that the cyclic oligosaccharides do not participate in the cross-linking reactions. In both cases, the influence of the essential oil, if any, is not clear. Similar results can be found in related papers [43].
Regarding water vapor permeability, the incorporation of free oil results in a slight (WG-TEO-10%) or a significant increase (WG-TEO-15%) in WVP values compared to that of the WG-Control sample, probably because its inclusion into the wheat gluten network leads to the reduction of the interactions between protein chains. On the other hand, the films incorporating microencapsulated TEO display significantly (WG-β-CD-TEO-10%) or slightly (WG-β-CD-TEO-15%) reduced WVP values with respect to that of the β-CD-control sample, that could be ascribed to the increase in network heterogeneity, leading to a more tortuous course for the passage of water vapor. However, it is clear that the increase in the content of microencapsulated TEO above 10% does not contribute to a further decrease in this property. This effect can be explained considering the changes introduced in the structure of the films by the β-CD-TEO inclusion complex when its amount is excessive, which, according to related works [43], leads to polymer networks with decreased intermolecular interactions between protein chains, which results in increased segmental motions as a result of the increased free volume of the system. In summary, moderate loading of microencapsulated TEO leads to a decrease in the WVP because the effect of increasing vapor path due to increased tortuosity predominates over the effect caused by increasing free volume of the system, but the opposite occurs for higher or excessive contents.
The optical properties of films are important characteristics that could determine the acceptability and applicability of foods in packaging systems. Light-permeable materials have absolute transparency as their characteristic optical property. The transparency of a package could affect the appearance and marketability of the food and therefore could determine the suitability of the film for various demands [46]. Therefore, the opacity values of the different wheat gluten-based films are shown in Table 2. Both control films exhibited lower opacity values than those containing free or microencapsulated TEO, which is attributed to the existence of an oil-dispersed phase in the films containing free essential oil that favors light scattering and therefore reduces transparency. On the other hand, samples including microencapsulated oil show a higher increase in opacity values compared to the analogous control samples, almost certainly due to both the greater opacity of the β-CD phase when it contains TEO, and the higher heterogeneity of the polymeric network, as was also noticed in other works [26].
The mechanical properties of the active wheat gluten films obtained from tensile tests were also studied. From the results presented in Table 2, it can be seen that the films containing free or microencapsulated TEO are less stiff than the corresponding control ones, since a decrease in tensile modulus (E) and strength (σm) as well as an increase in the elongation at break (εb) are observed as the TEO content increases. Evidently, the oily nature of the active component added to its less polar nature reduces the interactions developed between protein chains, leading to softer and more flexible films. The variations in the mechanical properties suggest that the polymeric network has a lower cohesion, almost certainly because the lipid phase partially hinders the formation of protein-protein intermolecular bonds, while favoring the formation of hydrophobic interactions during the drying stage of the film [47]. Regarding samples containing β-CD, it is evident that they have a less compact and less cohesive structure than films made only from plasticized wheat gluten, since these oligosaccharides also limit protein interactions, which become more evident when TEO is microencapsulated into them. Similar performance was noted in previous [18] and related works, i.e., wheat gluten films incorporating potassium sorbate obtained by compression molding [47]. The mechanical behavior of the films is influenced by the type and concentration of EO, the nature of the polymeric matrix and the specific interactions developed among components [48], which dictate the actual adhesion forces between the polymeric and oily phases. Usually, if immiscible and/or non-polymeric components such as essential oils or β-CDs (as in the present case), are included in excessive amounts into the polymeric matrix, the obtained films will comprise a large amount of dispersed phase, generating a structure that is too heterogeneous and contains a large number of discontinuities.
The dynamical mechanical behavior presented in Fig. 3 indicates that all materials exhibit a predominantly elastic character, since G′ higher than G″ over the entire temperature range studied. Moreover, these results also support the finding that TEO and β-CD behaves as co-plasticizers for the wheat gluten films, since their addition caused a reduction in both viscoelastic moduli that is more pronounced in the glassy region. This effect can again be attributed to the decrease of the protein-protein interactions due to the addition of both the TEO essential oil and the cyclic oligosaccharides [49], as discussed in previous sections. The glass transition temperature corresponding to the glycerol-rich phase (peaks in the loss modulus curves) decreased from 11°C for the control sample to about −6°C (films containing β-CD) and −12°C (films containing free TEO). Moreover, the curves of storage modulus clearly show that active wheat gluten films are less rigid than the control sample, although the observed trend does not exactly match the tensile behavior.
Figure 3: Dynamic mechanical assessments (torsion mode) as a function of the temperature of WG active films. (a) Storage modulus (G′) and (b) Loss modulus (G″)
3.3 Antimicrobial, Antioxidant and Release Properties
The concentration of thymol incorporated into film formulations prior to processing and the remained concentration after thermoplastic processing were calculated (data not shown). Results revealed a thymol retention after processing of approximately 71% when TEO was incorporated in its free form [18]. Encapsulation further reduces thymol losses, resulting in WG active films with ≥90% retention of thymol content after processing (93.1% and 90.2% for WG-β-CD-TEO-10% and WG-β-CD-TEO-15%, respectively). Thymol losses are mainly due to evaporation or degradation of the bioactive during high temperature and high pressure film processing. Similar results were obtained by Ramos et al. [50] by including thymol to polylactic acid (PLA) films prepared by compression molding and by Hwang et al. [51] by including resveratrol and α-tocopherol into poly(lactic acid)/starch blend films.
The antimicrobial activity of control and active WG films incorporated with free and microencapsulated TEO against selected pathogens is presented in Table 3. Control films were not effective against any of the tested bacteria, while both microencapsulation and TEO concentration affect significantly the antimicrobial performance of the active films (p < 0.05). As higher the TEO concentration (in any form), the larger is the film antimicrobial activity. The antimicrobial activity of thyme essential oil has been well demonstrated and is directly related to the synergistic effect of its main components thymol and carvacrol [19,52], as stated above. The action mechanism of TEO could be associated to the lipophilic characteristics of these major compounds, which attach themselves to the phospholipid bilayer of the cell membrane, increasing its permeability, therefore causing damage to the cellular enzymatic system by altering the cytoplasmic structure [53].
Wheat gluten films with encapsulated TEO presented higher inhibition zones compared to those containing free TEO oil against all pathogenic bacterial strains tested, and were also significantly less effective against Gram (−) bacteria than against Gram (+) bacteria, as also found in related works [54]. These results can be attributed to the higher thymol retention after film processing due to encapsulation, which sustains their antimicrobial activity. The use of encapsulated thymol for preserving the antimicrobial activity of films against E.coli and S.aureus was also reported by Yuan and co-workers by developing starch based composite films incorporated with thyme essential oil microcapsules and/or microemulsion [55].
Fig. 4 shows the antioxidant capacity and total phenolic content of WG films containing free and encapsulated TEO. The DPPH (2,2-diphenyl-1-picrylhydrazyl) radical scavenging rate of control films was close to zero, suggesting these films had no antioxidant capacity. Also in this case, both the encapsulation and concentration of TEO significantly affected the total polyphenol content and the antioxidant capacity of the active films. The antioxidant performance of WG films significantly increased (p < 0.05) as the concentration of TEO (incorporated in both free and microencapsulated forms) increases. On the other hand, the DPPH radical scavenging activity of films containing encapsulated TEO was significantly higher than that of samples prepared with free TEO, denoting that encapsulation preserves TEO from light, heat and pressure during film processing, improving the stability of the essential oil and therefore boosting the action of the antioxidant polyphenols contained in it. The use of microencapsulation for preserving the antioxidant activity of films was reported by other authors for starch films developed by casting and incorporated with black tea extract encapsulated in a pectin-sodium caseinate mixture [56], polyvinyl alcohol (PVA)-starch films obtained by casting and incorporated with β-cyclodextrin inclusion complex embedding lemongrass oil [28] and PVA films obtained by casting and incorporated with tea polyphenols-loaded starch microcapsules [57].
Figure 4: DPPH radical scavenging activity and TPC content of active WG films incorporated with free and microencapsulated TEO. ND = not detected
The excellent antioxidant activity of EOs, mainly attributed to its phenolic compounds content, has been reviewed by Adel et al. [23] and Bai et al. [30]. As reported in a previous publication [18], the total phenolic content of the pure thyme essential oil used in this work is high, 74.54 mg GAE/g and therefore it is not surprising that the TPC in the WG films increases significantly (p < 0.05) as the thyme essential oil concentration increases, in agreement with the results obtained for the free radical scavenging activity.
Fig. 5 shows the cumulative release of thymol as a function of time obtained from compression-molded active WG films containing free and microencapsulated TEO and immersed in 50% ethanol. The release rate showed no significant differences between films with different TEO concentrations for both types of films, i.e., containing free or encapsulated thyme essential oil. Moreover, in the release curves of the active component, two stages can be clearly distinguished: burst or fast release (0–25 h) and sustained slow release (25–360 h). The performance of the encapsulated TEO during the burst period could be ascribed to the presence of unencapsulated thymol in the surface of the β-CD microcapsules as well as to the part of the active component that is located very close to the inner surface of the capsules and therefore could be released in the early stages of the assay [39]. Furthermore, release kinetics showed that thymol release from WG films in the food simulant system is more sustained when TEO is incorporated as an inclusion complex compared to adding TEO directly into the films. The cumulative release rates at 24 h from the active WG films were approximately 73% and 65% for films containing free and microencapsulated TEO, respectively. Alinaqi and co-workers [58] reported results in agreement with those found in this work: a release of approximately 60% of clove essential oil encapsulated in zein nanoparticles and incorporated in a starch bionanocomposite film after 24 h using 50% ethanol as food simulant. Film formulations containing free TEO showed a more accelerated release, reaching equilibrium release rates of 95% (for both TEO concentrations) within 72 h, indicating that almost all the bioactive was released from the films after 3 days. On the other hand, encapsulated TEO was released from the active films in a more controlled way, compared to free TEO, supporting that microencapsulation of the active agent can delay the liberation rate of TEO. In this way, the TEO can be maintained into the film for longer periods of time, thus enabling the packaging to stay active for much longer. Similar results were also reported for whey protein edible films containing carvacrol and eugenol β-cyclodextrin inclusion complexes [59] and for gelatin films incorporated with β-cyclodextrin/curcumin complexes [60].
Figure 5: Cumulative release rate of thymol from active WG films
Release of compounds with low molecular weight from a polymeric matrix can be described in terms of the following stages: (1) diffusion of the solvent from the external solution into the polymer network; (2) swelling/relaxation of the polymeric network; (3) diffusion of the compound from the swollen macromolecular matrix to the external solvent solution [61,62]. In this case, once the WG matrix is swollen, the encapsulated thymol must first be released from the β-CD inclusion complexes, then migrate to the polymeric matrix and finally, pass through the film into the ethanol simulant until both phases reach thermodynamic equilibrium.
The experimental release results were fitted to short-term (Eq. (5)) and long-term (Eq. (6)) diffusion models. Both models fitted the experimental data quite well (R2 > 0.9), which therefore allowed the calculation of the diffusion coefficients (D) and release rate constants (k) presented in Table 4. Wheat gluten films containing encapsulated TEO presented lower D and k values (42% and 35%, respectively) compared to those exhibited by films prepared with free TEO, regardless the amount of TEO incorporated into the films, confirming that β-CD had a significant slow-release effect on the thyme essential oil.
Glycerol plasticized wheat gluten was successfully used to develop active, edible and suitable films for food packaging. The films were manufactured by intensive mixing of their components followed by compression molding of the resulting paste, a thermoplastic processing method that is fairly easy to scale up.
The incorporation of TEO in any of its forms (free or microencapsulated) led to films that absorb less moisture than the plasticized WG control film, present similar (free TEO) or higher (β-CD-TEO) water solubility, although slightly higher (free TEO) or even lower (microencapsulated TEO) water vapor permeability. Films containing TEO are more opaque and present lower tensile resistance and rigidity than control films, although this does not necessarily overturn their potential use as food packaging. The overall performance of these films was related to the reduction of the interactions developed between WG chains, caused by the incorporation of both, free or microencapsulated TEO into the polymeric matrix, which resulted in an increase of the free volume of these complex films, while increasing their heterogeneity. On the other hand, the incorporation of TEO in any form leads to the improvement of the antioxidant and antimicrobial properties of WG films.
This work also confirms that the encapsulation of TEO in β-CD is an effective means to protect the active component from thermal degradation, oxidation and/or evaporation during the obtaining of the thermo-compressed active films as well as to provide the films with attractive antioxidants and antimicrobial properties in addition to a sustained release rate of TEO in food simulant.
Acknowledgement: The authors thank the Consejo Nacional de Investigaciones Científicas y Técnicas (CONICET), the Agencia Nacional de Promoción de la Investigación, el Desarrollo Tecnológico y la Innovación (Agencia I+D+i) and the Universidad Nacional de Mar del Plata (UNMdP) for their financial support.
Funding Statement: This study received financial support from the Consejo Nacional de Investigaciones Científicas y Técnicas (CONICET) (Grant # PIP 0855), the Agencia Nacional de Promoción de la Investigación, el Desarrollo Tecnológico y la Innovación (Agencia I+D+i) (Grant # PICT 03507) and the Universidad Nacional de Mar del Plata (UNMdP) (Grant # 22/ G377).
Author Contributions: Conceptualization and design: Norma E. Marcovich and María Roberta Ansorena; data collection: Norma E. Marcovich, Matías Federico Hernández and María Roberta Ansorena; analysis and interpretation of results: Norma E. Marcovich and María Roberta Ansorena; investigation: Norma E. Marcovich and María Roberta Ansorena; writing—original draft preparation: Norma E. Marcovich, Matías Federico Hernández and María Roberta Ansorena; writing—review and editing, Norma E. Marcovich and María Roberta Ansorena; funding acquisition: Norma E. Marcovich and María Roberta Ansorena. All authors reviewed the results and approved the final version of the manuscript.
Availability of Data and Materials: Data available on request from the authors. The data that support the findings of this study are available from the corresponding author (María Roberta Ansorena) upon reasonable request.
Ethics Approval: Not applicable.
Conflicts of Interest: The authors declare no conflicts of interest to report regarding the present study.
References
1. Amin U, Khan MU, Majeed Y, Rebezov M, Khayrullin M, Bobkova E, et al. Potentials of polysaccharides, lipids and proteins in biodegradable food packaging applications. Int J Biol Macromol. 2021;183:2184–98. doi:10.1016/j.ijbiomac.2021.05.182. [Google Scholar] [PubMed] [CrossRef]
2. Petkoska AT, Daniloski D, Cunha NM, Naumovski N, Broach AT. Edible packaging: sustainable solutions and novel trends in food packaging. Food Res Int. 2021;140:109981. doi:10.1016/j.foodres.2020.109981. [Google Scholar] [PubMed] [CrossRef]
3. Chawla R, Sivakumar S, Kaur H. Antimicrobial edible films in food packaging: current scenario and recent nanotechnological advancements—a review. Carbohydr Polym Technol Appl. 2021;2:100024. doi:10.1016/j.carpta.2020.100024. [Google Scholar] [CrossRef]
4. Abdollahzadeh E, Nematollahi A, Hosseini H. Composition of antimicrobial edible films and methods for assessing their antimicrobial activity: a review. Trends Food Sci Technol. 2021;110:291–303. doi:10.1016/j.tifs.2021.01.084. [Google Scholar] [CrossRef]
5. Liu Y, Sameen DE, Ahmed S, Dai J, Qin W. Antimicrobial peptides and their application in food packaging. Trends Food Sci Technol. 2021;112:471–83. doi:10.1016/j.tifs.2021.04.019. [Google Scholar] [CrossRef]
6. Roy S, Ramakrishnan R, Afzia N, Ghosh T, Zhang W. Recent progress in the antimicrobial and antioxidant peptide activated film/coatings for food packaging applications: a review. Food Biosci. 2024;105288. doi:10.1016/j.fbio.2024.105288. [Google Scholar] [CrossRef]
7. Gupta D, Lall A, Kumar S, Patil TD, Gaikwad KK. Plant based edible films and coatings for food packaging applications: recent advances, applications, and trends. Sustain Food Tech. 2024;2:1428–55. doi:10.1039/D4FB00110A. [Google Scholar] [CrossRef]
8. Sharma S, Barkauskaite S, Jaiswal AK, Jaiswal S. Essential oils as additives in active food packaging. Food Chem. 2020;128403. doi:10.1016/j.foodchem.2020.128403. [Google Scholar] [PubMed] [CrossRef]
9. Firouz MS, Mohi-Alden K, Omid M. A critical review on intelligent and active packaging in the food industry: research and development. Food Res Int. 2021;110113. doi:10.1016/j.foodres.2021.110113. [Google Scholar] [PubMed] [CrossRef]
10. Zhang L, Yu D, Regenstein JM, Xia W, Dong J. A comprehensive review on natural bioactive films with controlled release characteristics and their applications in foods and pharmaceuticals. Trends Food Sci Tech. 2021;112:690–707. doi:10.1016/j.tifs.2021.03.053. [Google Scholar] [CrossRef]
11. Liu Z, Zheng Z, Zhu G, Luo S, Zhang D, Liu F, et al. Modification of the structural and functional properties of wheat gluten protein using a planetary ball mill. Food Chem. 2021;130251. doi:10.1016/j.foodchem.2021.130251. [Google Scholar] [PubMed] [CrossRef]
12. Qu M, Jiang P, Zhu Y, Zhu X, Liu L, Huang Y. Effects of glutenin/gliadin ratio and calcium ion on the structure and gelatinity of wheat gluten protein under heat induction. Food Biosci. 2024;58:103704. doi:10.1016/j.fbio.2024.103704. [Google Scholar] [CrossRef]
13. Dong M, Tian L, Li J, Jia J, Dong Y, Tu Y, et al. Improving physicochemical properties of edible wheat gluten protein films with proteins, polysaccharides and organic acid. LWT-Food Sci Technol. 2022;154:112868. doi:10.1016/j.lwt.2021.112868. [Google Scholar] [CrossRef]
14. Hu F, Song Y-Z, Thakur K, Zhang J-G, Khan MR, Ma Y-L, et al. Blueberry anthocyanin based active intelligent wheat gluten protein films: preparation, characterization, and applications for shrimp freshness monitoring. Food Chem. 2024;453:139676. doi:10.1016/j.foodchem.2024.139676. [Google Scholar] [PubMed] [CrossRef]
15. Jiménez-Rosado M, Zarate-Ramírez L, Romero A, Bengoechea C, Partal P, Guerrero A. Bioplastics based on wheat gluten processed by extrusion. J Clean Prod. 2019;239:117994. doi:10.1016/j.jclepro.2019.117994. [Google Scholar] [CrossRef]
16. Chen Y, Li Y, Qin S, Han S, Qi H. Antimicrobial, UV blocking, water-resistant and degradable coatings and packaging films based on wheat gluten and lignocellulose for food preservation. Compos Part B–Eng. 2022;238:109868. doi:10.1016/j.compositesb.2022.109868. [Google Scholar] [CrossRef]
17. Varghese SA, Siengchin S, Parameswaranpillai J. Essential oils as antimicrobial agents in biopolymer-based food packaging–a comprehensive review. Food Biosci. 2020;100785. doi:10.1016/j.fbio.2020.100785. [Google Scholar] [CrossRef]
18. Ansorena MR, Zubeldía F, Marcovich NE. Active wheat gluten films obtained by thermoplastic processing. LWT-Food Sci Technol. 2016;69:47–54. doi:10.1016/j.lwt.2016.01.020. [Google Scholar] [CrossRef]
19. Tao F, Hill LE, Peng Y, Gomes CL. Synthesis and characterization of β-cyclodextrin inclusion complexes of thymol and thyme oil for antimicrobial delivery applications. LWT-Food Sci Technol. 2014;59(1):247–55. doi:10.1016/j.lwt.2014.05.037. [Google Scholar] [CrossRef]
20. Yammine J, Chihib N-E, Gharsallaoui A, Ismail A, Karam L. Advances in essential oils encapsulation: development, characterization and release mechanisms. Polym Bull. 2024;3837–82. doi:10.1007/s00289-023-04916-0. [Google Scholar] [CrossRef]
21. Simionato I, Domingues FC, Nerín C, Silva F. Encapsulation of cinnamon oil in cyclodextrin nanosponges and their potential use for antimicrobial food packaging. Food ChemToxicol. 2019;132:110647. doi:10.1016/j.fct.2019.110647. [Google Scholar] [PubMed] [CrossRef]
22. Matencio A, Navarro-Orcajada S, García-Carmona F, López-Nicolás JM. Applications of cyclodextrins in food science. A review. Trends Food Sci Technol. 2020;104:132–43. doi:10.1016/j.tifs.2020.08.009. [Google Scholar] [CrossRef]
23. Adel AM, Ibrahim AA, El-Shafei AM, Al-Shemy MT. Inclusion complex of clove oil with chitosan/β-cyclodextrin citrate/oxidized nanocellulose biocomposite for active food packaging. Food Packag Shelf Life. 2019;20:100307. doi:10.1016/j.fpsl.2019.100307. [Google Scholar] [CrossRef]
24. Cid-Samamed A, Rakmai J, Mejuto JC, Simal-Gandara J, Astray G. Cyclodextrins inclusion complex: preparation methods, analytical techniques and food industry applications. Food Chem. 2022;384:132467. doi:10.1016/j.foodchem.2022.132467. [Google Scholar] [PubMed] [CrossRef]
25. Chen H, Li L, Ma Y, Mcdonald TP, Wang Y. Development of active packaging film containing bioactive components encapsulated in β-cyclodextrin and its application. Food Hydrocoll. 2019;90:360–6. doi:10.1016/j.foodhyd.2018.12.043. [Google Scholar] [CrossRef]
26. Cheng M, Wang J, Zhang R, Kong R, Lu W, Wang X. Characterization and application of the microencapsulated carvacrol/sodium alginate films as food packaging materials. Int J Biol Macromol. 2019;141:259–67. doi:10.1016/j.ijbiomac.2019.08.215. [Google Scholar] [PubMed] [CrossRef]
27. Buendía L, Sánchez MJ, Antolinos V, Ros M, Navarro L, Soto S, et al. Active cardboard box with a coating including essential oils entrapped within cyclodextrins and/or halloysite nanotubes. A case study for fresh tomato storage. Food Control. 2020;107:106763. doi:10.1016/j.foodcont.2019.106763. [Google Scholar] [CrossRef]
28. Chen Z, Zong L, Chen C, Xie J. Development and characterization of PVA-Starch active films incorporated with β-cyclodextrin inclusion complex embedding lemongrass (Cymbopogon citratus) oil. Food Packag Shelf Life. 2020;26:100565. doi:10.1016/j.fpsl.2020.100565. [Google Scholar] [CrossRef]
29. Zhang D, Cao G, Bu N, Huang L, Lin H, Mu R, et al. Multi-functional konjac glucomannan/chitosan bilayer films reinforced with oregano essential oil loaded β-cyclodextrin and anthocyanins for cheese preservation. Int J Biol Macromol. 2023;244:125365. doi:10.1016/j.ijbiomac.2023.125365. [Google Scholar] [PubMed] [CrossRef]
30. Bai M-Y, Zhou Q, Zhang J, Li T, Cheng J, Liu Q, et al. Antioxidant and antibacterial properties of essential oils-loaded β-cyclodextrin-epichlorohydrin oligomer and chitosan composite films. Colloids Surf B Biointerfaces. 2022;215:112504. doi:10.1016/j.colsurfb.2022.112504. [Google Scholar] [PubMed] [CrossRef]
31. Alves Z, Brites P, Ferreira NM, Figueiredo G, Otero-Irurueta G, Gonçalves I, et al. Thermoplastic starch-based films loaded with biochar-ZnO particles for active food packaging. J Food Eng. 2024;361:111741. doi:10.1016/j.jfoodeng.2023.111741. [Google Scholar] [CrossRef]
32. Almeida T, Karamysheva A, Valente BF, Silva JM, Braz M, Almeida A, et al. Biobased ternary films of thermoplastic starch, bacterial nanocellulose and gallic acid for active food packaging. Food Hydrocoll. 2023;144:108934. doi:10.1016/j.foodhyd.2023.108934. [Google Scholar] [CrossRef]
33. Iribarren E, Wongphan P, Bumbudsanpharoke N, Chonhenchob V, Jarupan L, Harnkarnsujarit N. Thermoplastic agar blended PBAT films with enhanced oxygen scavenging activity. Food Biosci. 2023;54:102940. doi:10.1016/j.fbio.2023.102940. [Google Scholar] [CrossRef]
34. Gutiérrez TJ, Mendieta JR, Ortega-Toro R. In-depth study from gluten/PCL-based food packaging films obtained under reactive extrusion conditions using chrome octanoate as a potential food grade catalyst. Food Hydrocoll. 2021;111:106255. doi:10.1016/j.foodhyd.2020.106255. [Google Scholar] [CrossRef]
35. Viacava GE, Cenci MP, Ansorena MR. Effect of chitosan edible coatings incorporated with free or microencapsulated thyme essential oil on quality characteristics of fresh-cut carrot slices. Food Bioproc Tech. 2022;15(4):768–84. doi:10.1007/s11947-022-02783-7. [Google Scholar] [CrossRef]
36. Zubeldía F, Ansorena MR, Marcovich NE. Wheat gluten films obtained by compression molding. Polym Test. 2015;43:68–77. doi:10.1016/j.polymertesting.2015.02.001. [Google Scholar] [CrossRef]
37. Abarca RL, Rodríguez FJ, Guarda A, Galotto MJ, Bruna JE. Characterization of beta-cyclodextrin inclusion complexes containing an essential oil component. Food Chem. 2016;196:968–75. doi:10.1016/j.foodchem.2015.10.023. [Google Scholar] [PubMed] [CrossRef]
38. Hill LE, Gomes C, Taylor TM. Characterization of beta-cyclodextrin inclusion complexes containing essential oils (trans-cinnamaldehyde, eugenol, cinnamon bark, and clove bud extracts) for antimicrobial delivery applications. LWT-Food Sci Technol. 2013;51(1):86–93. doi:10.1016/j.lwt.2012.11.011. [Google Scholar] [CrossRef]
39. Liu F, Avena-Bustillos RJ, Chiou B-S, Li Y, Ma Y, Williams TG, et al. Controlled-release of tea polyphenol from gelatin films incorporated with different ratios of free/nanoencapsulated tea polyphenols into fatty food simulants. Food Hydrocoll. 2017;62:212–21. doi:10.1016/j.foodhyd.2016.08.004. [Google Scholar] [CrossRef]
40. Cran MJ, Rupika L, Sonneveld K, Miltz J, Bigger SW. Release of naturally derived antimicrobial agents from LDPE films. J Food Sci. 2010;75(2):E126–33. doi:10.1111/j.1750-3841.2009.01506.x. [Google Scholar] [PubMed] [CrossRef]
41. Dorofte AL, Dima C, Bleoanca I, Aprodu I, Alexe P, Kharazmi MS, et al. Mechanism of β-cyclodextrin–thyme nanocomplex formation and release: In silico behavior, structural and functional properties. Carbohydr Polym Technol Appl. 2024;7:100422. doi:10.1016/j.carpta.2024.100422. [Google Scholar] [CrossRef]
42. Ahmed J, Alazemi A, Bini TB. Encapsulation of cinnamon and thyme essential oils in γ-cyclodextrin: studies on tribology and rheology of diluted emulsions and morphologies of encapsulates. ACS Food Sci Technol. 2024;4(8):1904–15. doi:10.1021/acsfoodscitech.4c00241. [Google Scholar] [CrossRef]
43. Lu N, Liu Y. Structural, physicochemical, and functional (antioxidant-antimicrobial) properties of 2-O-methyl-β-cyclodextrin inclusion with hexahydro-β-acids in chitosan films. Coll Surf B Biointerfaces. 2020;191:111002. doi:10.1016/j.colsurfb.2020.111002. [Google Scholar] [PubMed] [CrossRef]
44. Cai C, Ma R, Duan M, Lu D. Preparation and antimicrobial activity of thyme essential oil microcapsules prepared with gum arabic. RSC Adv. 2019;9(34):19740–7. doi:10.1039/C9RA03323H. [Google Scholar] [PubMed] [CrossRef]
45. Canales D, Montoille L, Rivas LM, Ortiz JA, Yañez-S M, Rabagliati FM, et al. Fungicides films of low-density polyethylene (LDPE)/inclusion complexes (Carvacrol and Cinnamaldehyde) against Botrytis cinerea. Coatings. 2019;9(12):795. doi:10.3390/coatings9120795. [Google Scholar] [CrossRef]
46. Hashemi SMB, Khaneghah AM. Characterization of novel basil-seed gum active edible films and coatings containing oregano essential oil. Prog Org Coat. 2017;110:35–41. doi:10.1016/j.porgcoat.2017.04.041. [Google Scholar] [CrossRef]
47. Rocca-Smith JR, Marcuzzo E, Karbowiak T, Centa J, Giacometti M, Scapin F, et al. Effect of lipid incorporation on functional properties of wheat gluten based edible films. J Cereal Sci. 2016;69:275–82. doi:10.1016/j.jcs.2016.04.001. [Google Scholar] [CrossRef]
48. Acosta S, Chiralt A, Santamarina P, Rosello J, González-Martínez C, Cháfer M. Antifungal films based on starch-gelatin blend, containing essential oils. Food hydrocoll. 2016;61:233–40. doi:10.1016/j.foodhyd.2016.05.008. [Google Scholar] [CrossRef]
49. Álvarez-Castillo E, Ramos M, Bengoechea C, Martínez I, Romero A. Effect of blend mixing and formulation on thermophysical properties of gluten-based plastics. J Cereal Sci. 2020;96:103090. doi:10.1016/j.jcs.2020.103090. [Google Scholar] [CrossRef]
50. Ramos M, Jiménez A, Peltzer M, Garrigós MC. Development of novel nano-biocomposite antioxidant films based on poly (lactic acid) and thymol for active packaging. Food Chem. 2014;162:149–55. doi:10.1016/j.foodchem.2014.04.026. [Google Scholar] [PubMed] [CrossRef]
51. Hwang SW, Shim JK, Selke S, Soto-Valdez H, Matuana L, Rubino M, et al. Migration of α-tocopherol and resveratrol from poly(L-lactic acid)/starch blends films into ethanol. J Food Eng. 2013;116(4):814–28. doi:10.1016/j.jfoodeng.2013.01.032. [Google Scholar] [CrossRef]
52. Yammine J, Gharsallaoui A, Fadel A, Mechmechani S, Karam L, Ismail A, et al. Enhanced antimicrobial, antibiofilm and ecotoxic activities of nanoencapsulated carvacrol and thymol as compared to their free counterparts. Food Control. 2023;143:109317. doi:10.1016/j.foodcont.2022.109317. [Google Scholar] [CrossRef]
53. Orhan-Yanıkan E, Gülseren G, Ayhan K. Antimicrobial characteristics of Thymus vulgaris and Rosa damascena oils against some milk-borne bacteria. Microchem J. 2022;183:108069. doi:10.1016/j.microc.2022.108069. [Google Scholar] [CrossRef]
54. Wang D, Li C, Pan C, Wang Y, Xiang H, Feng Y, et al. Antimicrobial activity and mechanism of action of oregano essential oil against Morganella psychrotolerans and potential application in tuna. LWT-Food Sci Technol. 2022;165:113758. doi:10.1016/j.lwt.2022.113758. [Google Scholar] [CrossRef]
55. Yuan L, Feng W, Zhang Z, Peng Y, Xiao Y, Chen J. Effect of potato starch-based antibacterial composite films with thyme oil microemulsion or microcapsule on shelf life of chilled meat. LWT- Food Sci Technol. 2021;139:110462. doi:10.1016/j.lwt.2020.110462. [Google Scholar] [CrossRef]
56. Rajapaksha SW, Shimizu N. Development and characterization of functional starch-based films incorporating free or microencapsulated spent black tea extract. Molecules. 2021;26(13):3898. doi:10.3390/molecules26133898. [Google Scholar] [PubMed] [CrossRef]
57. Miao Z, Lv R, Teng S, Cao C, Lu P. Development of antioxidant active packaging films with slow release properties incorporated with tea polyphenols-loaded porous starch microcapsules. Int J Biol Macromol. 2022;222:403–12. doi:10.1016/j.ijbiomac.2022.09.143. [Google Scholar] [PubMed] [CrossRef]
58. Alinaqi Z, Khezri A, Rezaeinia H. Sustained release modeling of clove essential oil from the structure of starch-based bio-nanocomposite film reinforced by electrosprayed zein nanoparticles. Int J Biol Macromol. 2021;173:193–202. doi:10.1016/j.ijbiomac.2021.01.118. [Google Scholar] [PubMed] [CrossRef]
59. Barba C, Eguinoa A, Maté JI. Preparation and characterization of β-cyclodextrin inclusion complexes as a tool of a controlled antimicrobial release in whey protein edible films. LWT-Food Sci Technol. 2015;64(2):1362–9. doi:10.1016/j.lwt.2015.07.060. [Google Scholar] [CrossRef]
60. Wu J, Sun X, Guo X, Ji M, Wang J, Cheng C, et al. Physicochemical, antioxidant, in vitro release, and heat sealing properties of fish gelatin films incorporated with β-cyclodextrin/curcumin complexes for apple juice preservation. Food Bioprocess Tech. 2018;11:447–61. doi:10.1007/s11947-017-2021-1. [Google Scholar] [CrossRef]
61. Li M, Zhang F, Liu Z, Guo X, Wu Q, Qiao L. Controlled release system by active gelatin film incorporated with β-cyclodextrin-thymol inclusion complexes. Food and Bioprocess Technol. 2018;11:1695–702. doi:10.1007/s11947-018-2134-1. [Google Scholar] [CrossRef]
62. Niazmand R, Razavizadeh BM. Active polyethylene films incorporated with β-cyclodextrin/ferula asafoetida extract inclusion complexes: sustained release of bioactive agents. Polym Test. 2021;95:107113. doi:10.1016/j.polymertesting.2021.107113. [Google Scholar] [CrossRef]
Cite This Article
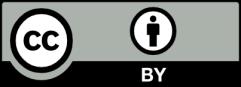
This work is licensed under a Creative Commons Attribution 4.0 International License , which permits unrestricted use, distribution, and reproduction in any medium, provided the original work is properly cited.