Open Access
ARTICLE
Thermosensitive and Wound-Healing Gelatin-Alginate Biopolymer Hydrogels Modified with Humic Acids
1 The Department of Oil, Gas and Solid Fuel Refining Technologies, National Technical University «Kharkiv Polytechnic Institute», Kharkiv, 61002, Ukraine
2 The Department of Plastics and Biologically Active Polymers Technology, National Technical University «Kharkiv Polytechnic Institute», Kharkiv, 61002, Ukraine
3 Faculty of Physics, V.N. Karazin Kharkiv National University, Kharkiv, 61000, Ukraine
4 Technical University of Liberec, Liberec, 46117, Czech Republic
5 The Department of Organizations and Management Healthcare and Social Medicine, National Technical University «Kharkiv Polytechnic Institute», Kharkiv, 61002, Ukraine
6 The Common Pharmacy Department, National Technical University «Kharkiv Polytechnic Institute», Kharkiv, 61002, Ukraine
7 Department of Micro- and Nanoelectronics, National Technical University «Kharkiv Polytechnic Institute», Kharkiv, 61002, Ukraine
* Corresponding Author: Sergey Petrushenko. Email:
Journal of Renewable Materials 2024, 12(10), 1691-1713. https://doi.org/10.32604/jrm.2024.054769
Received 06 June 2024; Accepted 19 September 2024; Issue published 23 October 2024
Abstract
The main goal of the article is the creation and study of thermosensitive and wound-healing gelatin-alginate biopolymer hydrogels modified with humic acids. Their rheological properties, swelling and contraction behavior were experimentally investigated, elucidated using Fourier transform infrared spectroscopy and used to achieve the physiological melting point, which is necessary for successful drug delivery. It has been shown that in the gelatin-alginate-humic acid biopolymer hydrogels systems, it is possible to obtain a gel-sol transition temperature close to the physiological temperature of 37°C, which is important for drug delivery in the treatment of wounds. By changing the type and concentration of humic acids in the gelatin-alginate hydrogel, it turned out to be achievable to regulate the softening time of the gel on the human body in the range from 6 to 20 min, which provides the possibility of controlled prolonged delivery of drugs. Based on the study of the influence of calcium ions on the properties of humic acids and ion exchange, as well as the interaction of humic acids, sodium alginate and gelatin with the formation of tighter gel networks, approaches to regulate the rate of softening of hydrogels at physiological temperature and their swelling, which simulates the absorption of exudate, were proposed and implemented. In addition, low shrinkage of the hydrogel surface due to cross-linking of gelatin-alginate networks when modified with humic acids was experimentally confirmed, which is important for avoiding problems of wound contracture and contour deformations when using dressings for wound healing. Thus, the developed optimized innovative biopolymer hydrogels synergistically combine the outstanding properties of natural molecular polymers and humic acids and are promising for the creation of effective medicines for wound healing.Graphic Abstract
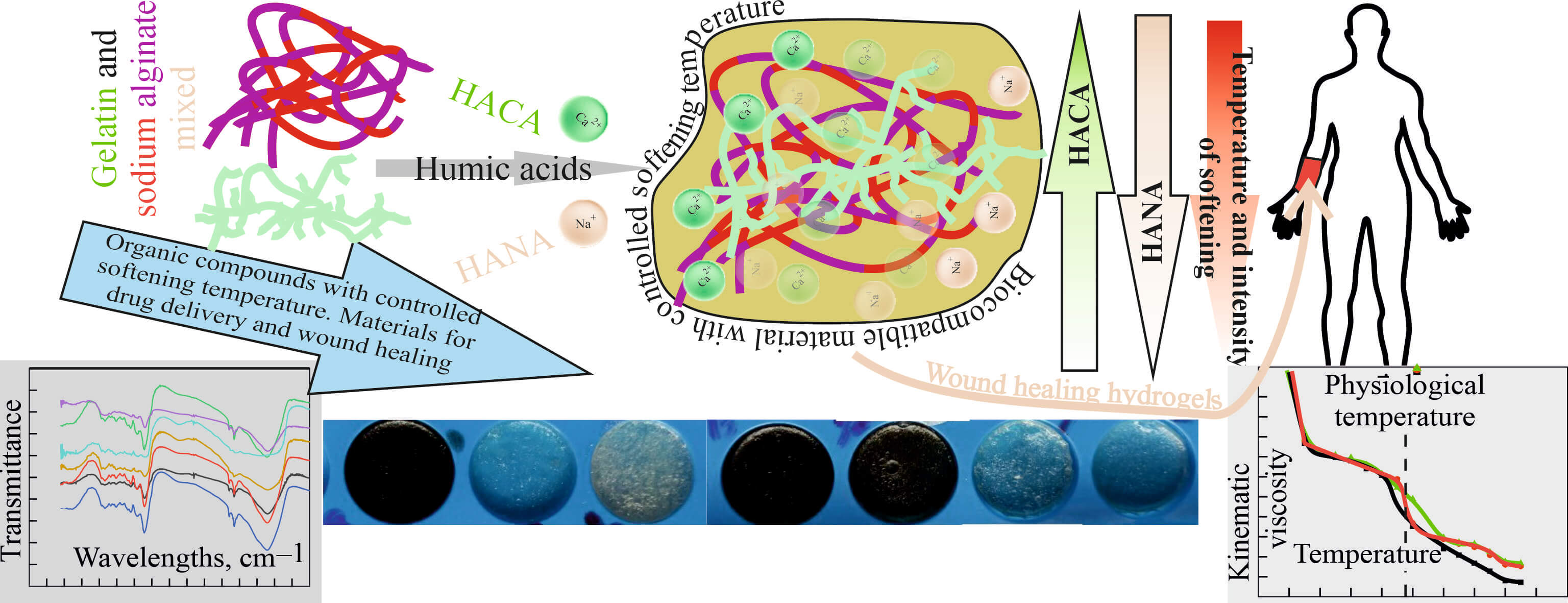
Keywords
Due to light weight, excellent mechanical properties, biocompatibility, non-toxicity and low cost biopolymers and their composites can be suitable for environmentally friendly and large-scale production and thus open new possibilities for functional electronics and biomedicine [1–3]. The use of green polymers and composites in biomedicine and bioengineering can help to restore and reconstruct damaged, lost or dysfunctional tissues of the body [4–8]. Another important application of biopolymers and their composites in biomedicine are temporary implants and functional medical actuators that can dissolve in the human body [4,5,8]. Biopolymers can also be used for biomedical purposes as hemostatic agents, wound dressings, and for drug delivery and wound healing, which is a dynamic and highly regulated process of regeneration of damaged skin through four overlapping stages: hemostasis, inflammation, proliferation and remodeling [9–13]. Among them, biopolymer hydrogels, the family of three-dimensional (3D) polymeric materials for which the bulk of the matrix consists of water (typically 75–90 wt. %), possess excellent diffusive properties and thus are widely used in drug delivery, regenerative medicine, tissue engineering, artificial organs, and related fields [13–17]. The shape and form of the gel can be customized via molding and fabrication techniques, which allows the biopolymer hydrogel components to mimic biotissues and organs as well as design actuators and sensors for various biomedical applications [16]. The association of biopolymer hydrogel molecules occurs due to non-covalent bonds, such as hydrogen bonds, π-π stacking, Van der Waals, metal coordination, electrostatic interactions and host-guest interactions. It is generally thermosensitive and thermoreversible. The resulting aggregates, usually with a high aspect ratio, form a three-dimensional network and impart viscoelastic properties to the mixtures [18,19]. According to [18], temperature-sensitive hydrogels would be subjected to water absorption or dehydration when the temperature changes, resulting in the expansion or contraction in volume or shape due to the variations in the enthalpy and entropy equilibriums, molecular rearrangement, interactions between hydrogel network and water [18]. The sol-gel transition temperature (TSG), as well as the gel-sol transition temperature (TGS), indicate the temperature range of thermodynamic stability of gels [19]. Variations in TGS depending on the concentration of the biopolymer hydrogel and its composition determine the boundary between two domains in the phase diagram: sol and gel. The shape/volume change property of biopolymer hydrogels is useful for creating actuators in soft robots and drug delivery systems [16–18]. In addition, hydrogels with a critical solution temperature close to human body temperature (i.e., 37°C) are particularly preferred for drug transport and release applications, so that drug release from the hydrogel-based system can be spontaneously activated as soon as the drug attaches to human skin or enters the human body [17].
Since their emergence, hydrogels have long been used as carriers for targeted drug delivery systems due to their large number of functional groups for drug immobilization, excellent biocompatibility with the in vivo environment, and controlled degradation period for self-destruction [9,10,12–16]. Novel stimulus-responsive hydrogels, including temperature-sensitive hydrogels, as a subset of hydrogel materials that exhibit significant deformation after exposure to environmental stimuli, may provide additional benefits such as precisely controlled drug release at the desired time and location [16–18]. Multiphase hydrogels consist of different phases, are heterogeneous and generally turbid. These gels contain cross-linking of different materials and fiber reinforcement, which combines the properties of different materials. The main reason for the occurrence of individual phases in these hydrogels are extensive cross-links that prevent entropy and growth of polymer chains [9,11–15,20]. Modern studies have shown the effectiveness of using multiphase hydrogels based on alginate or sodium alginate (SA) biopolymer in tissue engineering, as well as for biomedical delivery during hemostasis [10], as local bioactive wound dressings [11,12] and as biologically active transdermal patches in cosmetics [21–23].
SA is a hydrophilic and anionic polysaccharide that can be extracted from brown seaweed. Structurally, it is a linear copolymer consisting of alternating blocks of (1,4)-α-Lguluronate (G-unit) and (1,4)-β-d-mannuronate (unit M) [2,12,15,20,24,25]. As a natural polysaccharide, SA has a pH-dependent anionic nature and can interact with cationic polyelectrolytes and proteoglycans [2]. Pure solutions of SA do not exhibit temperature effects [20,25]. At the same time, SA are capable of forming hydrogels through ionotropic gelation with divalent or multivalent cations. For example, in the presence of calcium ions, the carboxyl groups of SA in the G-units of adjacent polymer chains are cross-linked through interaction with Ca2+ to form calcium alginate, which exhibits an egg-box model with excellent mechanical properties [2,24,25]. In addition, the authors of [26] described the formation of metal-free SA hydrogels in the presence of the free organic amino acid glutamine, when the protonated amino acid has a shielding effect upon electrostatic repulsion of SA chains, leading to the chain cross-linking and chain-chain stabilization.
One of the promising double-network and multiphase hydrogels based on SA is gelatin-SA hydrogel, the structure of which is formed by homopolymer and heteropolymer blocks [14,20,21,23,27]. Gelatin (GN) is a translucent protein compound produced by partial hydrolysis and denaturation of the insoluble fibrous protein collagen, which can be obtained from animals such as cattle, pigs, and marine organisms such as fish scales and fish skin [2]. It is a natural polyampholyte having both positively and negatively charged groups, as well as hydroxyl groups and hydrophobic groups [27]. The secondary structure of GN is formed by various polypeptide chains, including α-chains, β-chains and γ-chains and, unlike native collagen, it is soluble in water because its three-helix peptide structure “unwinds” into individual peptide chains [2]. Now, GN has shown wide application potential in various fields due to its large abundance and biocompatibility. In particular, GN is widely used in the synthesis of wound dressings and is one of the most commonly used biopolymers, the hydrogels of which are thermoreversible [20,28]. GN provides immediate homeostasis and also prevents wound contracture and contour deformities associated with traditional wound healing. Typically, thermoreversible physical GN hydrogels are prepared by cooling aqueous GN solutions to temperatures below 30°C, where a sol-gel transition occurs due to the GN chains undergoing progressive conformational changes known as the coil-to-helix transition, resulting in the formation of triple helix network [28]. According to [20,28], at GN concentrations above 13 wt. % and temperatures below 35°C, the thermodynamic conformation of GN chains is essentially intermolecular. The melting point TGS of a hydrogel with 2.5 wt. % GN is 32.4°C, and in a hydrogel containing 5 wt. % GN it increases to 34°C. Thus, the melting point TGS of pure GN hydrogel is always below the physiological temperature of 37°C [20]. In addition, the hygroscopic nature of GN films makes it difficult to be used in high moisture conditions [2].
As such, great efforts have been made to develop GN-based hydrogels with an increase in TGS to physiological level and improved mechanical and water resistance properties by combining with other biopolymers and other natural substances through stable and safe cross-linking. For example, GN-SA hydrogels, GN-SA-H2O, described in [20,27], demonstrate a joint synergistic effect of SA and GN on rheological and thermoresponsive properties due to intermolecular electrostatic interactions and hydrogen bonds. According to [27], complex formation in GN-SA-H2O hydrogels is accompanied by a change in the secondary structure of GN in the form of a decrease in the proportion of collagen-like helixes and an increase in the proportion of random coil conformation of GN chains. As a result of electrostatic interactions, hydrogen bonds and hydrophobic interactions between SA and GN, the characteristic temperatures of the gel-sol and sol-gel transitions in GN-SA-H2O shifted to higher values, the gelation temperature TSG increased to 27.3°C, and the melting temperature TGS increased to 35.9°С [20].
In [25], the addition of humic acids was used as a way to regulate the gelation of GN hydrogels as well as to improve their wound healing ability. Humic acids are the most studied group of naturally occurring humic substances, which are non-toxic complexing ligands [29–33]. It should be noted that humic substances constitute the main part of organic matter in soil, peat, brown coals, wastewater, natural waters and their sediments [33]. Therefore, humic acids can be considered as biological wastes that occur in nature in large quantities [28]. The chemical composition and properties of humic acids can vary depending on geographic location, origin, age, climate and biological conditions. According to the currently generally accepted opinion [28], humic acids consist of relatively low molecular weight compounds (2–150 kg/mol), which self-organize into supramolecular structures held by weak dispersion forces, such as Van der Waals, π-π and interactions CH-π. According to [28], the inclusion of humic acids in the GN network improves the viscoelastic properties and also slightly enhances the thermal stability of the resulting composite biopolymer hydrogel, in which the melting temperatures TGS increase up to 33°С–35°С. Industrial humic acids are isolated from peat and brown coals in the form of a fraction that is soluble in alkalis, but insoluble upon subsequent acidification [28–30]. Depending on the sodium hydroxide or calcium hydroxide used in their production process, humic acids called HANA may contain sodium ions, and humic acids called HACA, respectively, may contain calcium ions. Both HANA and HACA contain quinone, phenolic, carboxyl and hydroxyl groups in their main chains, which impart various properties such as antioxidant, antibacterial and anti-inflammatory activity [29–33]. According to [29], quinones are responsible for the formation of reactive oxygen species, which have a beneficial effect on wound healing and have fungicidal/bactericidal properties. Phenols and carboxylic acids deprotonated in neutral and alkaline environments are responsible for various other functions in humic acids, such as antioxidant and anti-inflammatory properties, in particular due to the ability to scavenge free radicals [29]. In [28], humic acids HANA obtained from Sigma-Aldrich at concentrations of HANA and GN up to 13.33 (w/w)% demonstrated an improvement in the thermal properties of the resulting hydrogels GN-HANA-H2O due to the close physical interaction of humic acids with GN. These interactions were carried out through non-covalent interactions such as hydrophobic and electrostatic interactions, H-bonding between the carbonyl groups of HANA and the hydroxyl groups of hydroxyproline, as well as proline residues and glycine amino moieties, which are the most abundant amino acids in GN chains [29]. Thus, up to a maximum concentration of GN and HANA of 13.33 (w/w)%, these humic acids had a beneficial effect on the gelation process, promoting the formation of a denser network. However, higher concentrations of HANA resulted in a disordered structure with a random coil organization of GN, since humic acids, due to their high affinity for water, establish preferential bonds with water molecules, preventing them from being coordinated with GN chains [29]. According to [29], since water plays a key role in stabilizing the triple helix structure of GN and the resulting gel network, being able to act as a link between GN chains through H-bonds, at high HANA content the secondary structure of GN lost its triple helix structure. In addition, in [29], HANA content above 13.3 wt. % caused swelling phenomena due to the absorption of water by humic acids and obtaining a hydrogel of GN and humic acids with reduced thermal stability. Moreover, it also caused partial coagulation of the GN to form aggregates, as well as precipitation phenomena that prevented the GN chains from returning to the helices of the ternary structure. In general, all thermosensitive hydrogels developed to date based on the GN-SA-H2O and GN-HANA-H2O systems [20,27,28] have a melting point below the physiological temperature of 37°C. Thus, given the relevance of creating biocompatible thermosensitive hydrogels with a physiological melting point based on non-toxic and accessible biomaterials that have wound-healing properties and are suitable for controlled drug delivery, a more complex GN-SA-HANA-H2O system was developed and studied in this work.
The authors of [34,35] confirmed the interactions of humic acids with calcium ions that can occur in the HACA obtained in this work. According to [34], Ca2+ is mainly associated with the carboxyl groups of humic acids, forming bidentate complexes that affect aggregation by linking the carboxyl groups of different molecules, bringing them closer and holding them together. In addition, calcium ions can also influence aggregation by simultaneously coordinating two carboxyl groups of the same humic acid molecule, resulting in the formation of a calcium-humic acid complex with a reduced net negative charge, allowing such complexes to approach each other and interact through hydrogen bonds. Thus, the presence of Ca2+ is fundamental to maintaining the stability of HACA aggregates as temporary calcium ion traps [34]. The dynamic aggregation–disaggregation processes in HACA should mediate calcium transport in GN/SA hydrogels modified with humic acids, which is important for their use in wound healing and drug delivery.
To improve the functionality of SA and SA-GN hydrogels in relation to their biomedical applications, such as wound healing and drug delivery, cross-linking of these hydrogels with divalent cations, especially Ca2+ from calcium salt solutions, is used [36]. Cross-linking of SA with Ca2+ cation is usually carried out by immersing the dried SA membrane in a calcium salt solution [36]. SA can also be ionic cross-linked in the sol phase by pouring a calcium salt solution on top of the SA solution, dialyzing a gel bath containing the calcium salt solution, and slowly releasing the Ca2+ ion from the calcium-gluconolactone complex [36]. However, according to [36], cross-linking in the sol phase can lead to wound contracture and contour deformities associated with conventional wound healing. In [36], the authors reduced gel compression during the sol-gel transition of an SA hydrogel by mixing a film-forming solution containing SA and Ca2+ with GN, which itself provides immediate homeostasis and is also widely used in the synthesis of wound dressings, and thus obtained modificated by calcium GN-SA-H2O hydrogel with a semi-interpenetrating network. Considering the antioxidant, antibacterial, fungicidal and anti-inflammatory activity of humic acids [28–33] and their effect on the viscoelastic properties of GN-SA-H2O hydrogels, it is expected that they will improve the biomedical characteristics of hydrogels in wound healing and drug delivery.
The main aim of the article is to design and research of thermosensitive and wound-healing GN-SA biopolymer hydrogels modified with humic acids. This work presents the results of a comparative analysis of the use of two types of humic acids HANA and HACA, obtained by our own methods, in GN-SA-H2O biopolymer hydrogels. It was necessary to investigate the influence of ion exchange of Ca2+ from HACA and Na+ from SA, which would lead to the production of a semi-interpenetrating biopolymer hydrogel matrix of GN-SA-HACA-H2O. Herein, we experimentally investigated the rheological properties, swelling and contraction behavior of the developed thermosensitive and wound-healing GN-SA biopolymer hydrogels modified with humic acids and used Fourier transform infrared spectroscopy to explain the obtained experimental results.
In this work we used such objects as GN food gelatin brand R-11 (TM Mriya, PJSC Ukroptbakalia, Chernihiv City, Ukraine) and SA sodium alginate (Lianyungang Fengyun Seaweed Manufacturer Co., Ltd., Lianyungang, China). Humic acids HANA and HACA were obtained by extraction from brown coal with a solution of sodium pyrophosphate (tetrasodium pyrophosphate, CAS 7722-88-5, Daily Chemicals, New York, NY, USA) and further extraction with 1% NaOH (sodium hydroxide, CAS:1310-73-2, Zhangfei Industrial Park, Hightech Zone, Liaocheng City, Shandong Province, China) or 0.2% Ca(OH)2 (calcium hydroxide, CAS:1305-62-0 Hebei Ruisite Technology Co., Ltd. Shijiazhuang, Hebei, China), respectively, followed by precipitation with HCl (hydrochloric acid, DSTU 3118, Trading House ATK Ukraine LLC, Kharkiv, Ukraine).
2.2 Extraction Methods of HANA and HACA
Below we show the flowchart of HANA or HACA provisioning:
1. Crushing 10–15 g of brown coal to <1 mm size.
2. Extracting with toluene (DSTU 5789, Ukraine), air drying and grinding residue to <0.2 mm size.
3. Transferring 1.5 g of residue to flask A (250 cm³), adding 100 cm³ sodium pyrophosphate solution to flask A, stirring for 1 h using a mechanical shaker.
4. Сentrifuging at 210 s−1 for 15 min, decanting the solution to flask B (1000 cm³).
5. Rinsing the residue twice with of 1 wt. % sodium hydroxide solution for HANA or 0.2 wt. % calcium hydroxide solution for HACA, centrifuging and collecting the rinse solution in flask B.
6. Transferring the residue to flask A, adding hydroxide solution, and heating for 2 h, cooling to room temperature, centrifuging, and decanting to flask B.
7. Rinsing the residue twice, centrifuging, and collecting in flask B, filtering the contents of flask B into a new 1000 cm³ flask, add water to make up to 1000 cm³.
8. Adding 60 cm³ of 5% HCl to 100 cm³ of filtrate to precipitate humic acids, centrifuging, decanting, and rinsing the precipitate with water.
9. Adding 5 cm³ of 5% HCl to colloidal solution to precipitate humic acids, filtering through ash-free filter, drying at (90 ± 5)°C, transferring the filter with precipitate to crucible, annealing at (600 ± 25)°C for 1–2 h.
10. Cooling, drying, and weighing until mass difference is <0.001 g, determination the mass of HANA or HACA by subtracting mass of crucible.
To prepare the thermosensitive and wound-healing biopolymer hydrogels, the calculated amount of GN was first placed in distilled water preheated to 90 ± 2°C and stirred on a water bath with a VEVOR 85-2 magnetic stirrer with a heating plate until a pure GN sol was obtained. Then SA was added to the pre-prepared GN sol and stirred with a BEVOR 85-2 magnetic stirrer with a heating plate until a homogeneous GN-SA-H2O sol was obtained. Before adding to the GN-SA sol, humic acids were partially dissolved in aqueous alkaline solutions. Fig. 1 shows optical micrographs of concentrated suspensions of HANA in a solution of 1 wt. % NaOH and HACA in a solution of 0.2 wt. % Ca(OH)2 before their addition to the GN-SA-H2O system.
Figure 1: Optical micrographs of aqueous solutions of humic acids: (a)–HANA in 1 wt. % aqueous solution of NaOH; (b)–HACA in 0.2 wt. % aqueous solution of Ca(OH)2
Thus, GN-SA biopolymer hydrogels modified with humic acids with the GN-SA-HANA-H2O and GN-SA-HACA-H2O systems were obtained, which are presented in Table 1.
Herein, rheological measurements were carried out to study the gel-sol transition temperature of the obtained biopolymer hydrogels. For this purpose, we used a standard method for determining kinematic viscosity (mm2/s) using a glass viscometer VPZh-2 3.35 with a capillary diameter of 3.35 mm and a viscometer constant of 10. Measurement of kinematic viscosity using a VPZh-2 3.35 viscometer was based on determining the time of flow of a certain volume of sol through a capillary from a measuring container. The kinematic viscosity for each temperature was recorded from the moment the sol began to flow freely through the widening in a capillary with a diameter of 3.35 mm.
The methodology for studying the gel-sol transition time of thermosensitive bioactive biopolymer hydrogels of the GN-SA-H2O, GN-SA-HANA-H2O and GN-SA-HACA-H2O systems included visual observation and determination of the time of softening and subsequent melting of the biopolymer hydrogels. To do this, molded biopolymer hydrogel washers with a height of 1 cm and a diameter of 1.5 cm were placed on a glass substrate preheated to a physiological temperature of 37°C to simulate the gel-sol transition process when applied to human skin. The results of the experiment were recorded with a digital camera.
To evaluate the ability of biopolymer hydrogels to absorb exudate at a wound site, we used free swelling absorption capacity measurements in accordance with [9,28,36] European Standard EN 13726:2023 “Test Methods for Wound Dressings. Aspects of absorption, moisture vapor transfer, waterproofing and stretchability”. For this, samples of GN-SA-H2O, GN-SA-HANA-H2O and GN-SA-HACA-H2O biopolymer hydrogels in the form of washers with a diameter of 1.5 cm, a thickness of 3 mm, and a dry weight W0 of 1.8 g, were immersed for 30 s into Petri dishes filled with an aqueous solutions of 142 mM NaCl and 2.5 mM CaCl2 heated to (37 ± 1)°C at a solution/hydrogel volume ratio of 40:1. After 30 s of swelling, the weight of sample W1 was recorded. The absorption capacity of hydrogels during free swelling was calculated using the Eq. (1):
Free swell absorptive capacity, =(W1−W0)W0×100 (1)
The contraction of the hydrogels during crosslinking and drying was determined to assess wound contracture and contour deformations associated with conventional wound healing. According to [36], surface contraction was determined as the percentage ratio of the surface of each swollen hydrogel sample A0 and the surface of the corresponding dry hydrogel sample A1 after 48 h of exposure to air. Surface contraction of the hydrogel was calculated using Eq. (2):
Surface contraction, =A1×100A0 (2)
The reduction in hydrogel volume was determined as the percentage of the volume of each swollen hydrogel sample V0 and the volume of the corresponding dry hydrogel sample V1 after 48 h of exposure to air. Volume contraction was calculated using Eq. (3):
Volume contraction, =V1×100V0 (3)
Fourier transform infrared spectroscopy was used to study the non-covalent interactions between GN, SA and humic acids HANA and HACA in these thermosensitive and wound-healing biopolymer hydrogels having a physiological melting point. FTIR spectra were recorded on a Nicolet 380 IR spectrophotometer (USA) at 20°C–25°C in the frequency range 4000–500 cm−1.
Considering the data [20] that in the thermosensitive biopolymer hydrogel of the GN-SA-H2O system with 5 wt. % GN and 5 wt. % SA, the melting point TGS is 35.9°C, it was decided to enhance the thermal stability of the GN-SA biopolymer hydrogel and thus bring TGS closer to the physiological level of 37°C by increasing the concentration of its components. When selecting the concentration of GN, we took into account the data [20,28] that in GN-H2O systems with a GN content of more than 13 wt. % at elevated temperatures not higher than 40°C, GN chains undergo a progressive conformational change from coil to helix, forming a triple helix networks. Therefore, the thermosensitive biopolymer hydrogels, the rheological studies of which were carried out at the first stage of this work, contained 14 wt. % GN and various concentrations of SA: 3.2 wt. % SA, 6.4 wt. % SA or 9.6 wt. % SA. Experimental studies revealed that for a biopolymer hydrogel containing 14 wt. % GN and 3.2 wt. % SA, the formation of a stable gel state was not observed in the entire range of kinematic viscosity studies, that is, at temperatures above 31°C. The SA-rich GN-SA-H2O hydrogel, containing 14 wt. % GN and 9.6 wt. % SA, had TGS ≈ 71°C, and the formation of its stable sol flow was not observed in the kinematic viscosity studies. According to [24], this behavior can be explained by the presence of a large number of polymer SA chains responsible for highly intertwined bonds, and are similar to chemical gels that are covalently cross-linked and therefore do not melt, i.e., are not thermosensitive biopolymer hydrogels. As can be clearly seen from Fig. 2, according to rheological studies, the GN-SA biopolymer hydrogel containing 14 wt. % GN and 6.4 wt. % SA is thermosensitive and has a gel-sol transition, manifested in a sharp decrease in its kinematic viscosity at a TGS temperature of ~36.4°C. According to [28] and based on the data of our previous works [21–23], which showed an improvement in the mechanical and transdermal characteristics of GN biopolymer hydrogels modified with humic acids, in this work we added humic acids to a thermosensitive bioactive GN-SA biopolymer hydrogel containing 6.4 wt. % SA and 14 wt. % GN.
Figure 2: Rheological studies of the thermosensitive bioactive biopolymer hydrogel GN-SA6.4-H2O containing 14 wt. % GN and 6.4 wt. % SA, and biopolymer hydrogels GN-SA-HANA2.5 and GN-SA-HANA5 modified with humic acids, obtained by adding to this biopolymer hydrogel 2.5 and 5 wt. % HANA, respectively
When modified with HANA humic acids, this hydrogel, as shown in Fig. 2, slightly increases the gel-sol transition temperature. The biopolymer hydrogel with the addition of 2.5 wt. % HANA has a TGS of ~36.9°C, and the biopolymer hydrogel with the addition of 5 wt. % HANA has a TGS of ~37.2°C. As seen in Fig. 2, both GN-SA-HANA2.5-H2O and GN-SA-HANA5-H2O sols obtained at temperatures above TGS showed a slight increase in the level of kinematic viscosity. At the same time, when the content of HANA was increased to 7.5 wt. % in this GN-SA hydrogel, the range of the gel-sol transition shifted to TGS temperatures in the range of 45°C–50°С (not shown in Fig. 2). The above correlates well with data [28] for the GN-HANA-H2O system, in which humic acids improved the mechanical and thermal properties of the GN hydrogel, promoting the formation of a tighter network. When modifying the thermosensitive bioactive biopolymer hydrogel GN-SA6.4-H2O with humic acids HACA to obtain biopolymer hydrogels GN-SA-HACA2.5-H2O, GN-SA-HACA5-H2O and GN-SA-HACA7.5-H2O, a significant increase in TGS occurred up to temperatures in the range of 55–60°С. In addition, when studying kinematic viscosity, the formation of a stable sol flow was not observed, as a result of which it was not possible to study the rheological properties of biopolymer hydrogels of the GN-SA-HACA-H2O system using the standard method of determining kinematic viscosity using a glass viscometer. According to [12,20,27,36], in biopolymer hydrogels containing humic acids from an aqueous solution of HACA and 0.2 wt. % Ca(OH)2, ion exchange occurred between Na+ from SA and Ca2+, including from HACA due to the anionic nature and chelating ability of SA, with the subsequent formation of tighter networks of more viscous and thermostable permanent gels.
Fig. 3 shows photographs of molded washers from thermosensitive and wound-healing bioactive biopolymer hydrogels containing 6.4 wt. % SA and 14 wt. % GN, modified with humic acids HANA and HACA, prepared in this work, the compositions of which are presented in Table 1. Due to differences in the methods for obtaining HANA and HACA, the colors of the biopolymer hydrogels of GN-SA-HANA-H2O and GN-SA-HACA-H2O systems are completely different. Optical micrographs of thermosensitive bioactive biopolymer hydrogels containing 6.4 wt. % SA and 14 wt. % GN, including those modified with humic acids HANA and HACA, are presented in Fig. 4. As confirmed by optical micrographs of biopolymer hydrogels of the GN-SA-HANA-H2O systems in Figs. 4e–g, they contain large undissolved dark particles up to tens of micrometers in size, the amount of which increases with increasing concentration of HANA in the GN-SA biopolymer hydrogel from 2.5 to 7.5 wt. %. These microparticles belong to HANA, as evidenced by the optical micrograph of HANA in Fig. 1a.
Figure 3: Photographs of molded washers from thermosensitive and wound-healing hydrogels prepared in this work, the compositions of which are presented in Table 1
Figure 4: Optical micrographs of thermosensitive and wound-healing biopolymer hydrogels prepared in this work, the compositions of which are presented in Table 1: (a)–GN-SA6.4-H2O; (b)–GN-SA-HACA 2.5-H2O; (c)–GN-SA-HACA5-H2O; (d)–GN-SA-HAСA7.5-H2O; (e)–GN-SA-HANA2.5-H2O; (f)–GN-SA-HANA5-H2O; (g)–GN-SA-HANA7.5-H2O
Photographs in Fig. 5 show visually the results of a study of the softening of the biopolymer hydrogels due to the destabilization of their numerous hydrogen bonds when the temperature rises to 37° for sample of the developed gelatin-alginate hydrogel GN-SA6.4-H2O, as well as for this biopolymer hydrogel modified with humic acids HACA to obtain GN-SA-HACA2.5-H2O, GN-SA-HACA5-H2O and GN-SA-HAСA7.5-H2O. A slower transition from hard gel to soft gel is seen with increasing HACA content compared to pure GN-SA hydrogel. At the same time, softening of the gel occurs mostly in the layers of the GN-SA-HACA2.5-H2O, GN-SA-HACA5-H2O and GN-SA-HAСA7.5-H2O biopolymer hydrogel samples near the heated glass surface, practically without passing into the volume of the material. Fig. 6 shows similar experiments for GN-SA-HANA2.5-H2O, GN-SA-HANA5-H2O and GN-SA-HANA7.5-H2O biopolymer hydrogels in comparison with pure GN-SA hydrogel, confirming the rapid softening of these biopolymer hydrogels. Fig. 7 shows graphs of the transition time between hard gel and soft gel at a temperature of 37°C on the concentration of humic acids HANA and HACA in the developed GN-SA biopolymer hydrogels, containing 6.4 wt. % SA and 14 wt. % GN, the compositions of which are presented in Table 1. A sharp increase in the time of this transition at 37°C from 6 to 11 min can be observed as a result of the addition of 2.5 wt. % HANA to the GN-SA hydrogel. Further increasing the HANA content in the biopolymer hydrogel to 5 and 7.5 wt. % resulted in a slight increase in the hard-soft transition time to 11.5 and 12 min, respectively. When modifying the GN-SA biopolymer hydrogel using HACA, it is observed in the graph in Fig. 7 significant growth in the hard gel-soft gel transition time with increasing HACA content from 15 to 17.5 and up to 20 min, for biopolymer hydrogels HACA2.5-H2O, GN-SA-HACA5-H2O and GN-SA-HACA7.5-H2O, respectively. The increase in the softening time of gelatin-alginate hydrogels by both types of humic acids can be explained by the need for additional time with increasing temperature to weaken and destroy new non-covalent intermolecular bonds that arise during the modification of HANA, and especially HACA. Overall, the study results showed that relatively small concentrations of both HANA and NAСA can provide prolonged drug delivery and wound healing effects using humic acid-modified GN-SA biopolymer hydrogels on the human body surface.
Figure 5: Rheological studies of the thermosensitive bioactive biopolymer hydrogel GN-SA6.4-H2O containing 14 wt. % GN and 6.4 wt. % SA, and biopolymer hydrogels GN-SA-HANA2.5 and GN-SA-HANA5 modified with humic acids, obtained by adding to this biopolymer hydrogel 2.5 and 5 wt. % HANA, respectively
Figure 6: Rheological studies of the thermosensitive bioactive biopolymer hydrogel GN-SA6.4-H2O containing 14 wt. % GN and 6.4 wt. % SA, and biopolymer hydrogels GN-SA-HANA2.5 and GN-SA-HANA5 modified with humic acids, obtained by adding to this biopolymer hydrogel 2.5 and 5 wt. % HANA, respectively
Figure 7: Rheological studies of the thermosensitive bioactive biopolymer hydrogel GN-SA6.4-H2O containing 14 wt. % GN and 6.4 wt. % SA, and biopolymer hydrogels GN-SA-HANA2.5 and GN-SA-HANA5 modified with humic acids, obtained by adding to this biopolymer hydrogel 2.5 and 5 wt. % HANA, respectively
The results of the analysis of the biopolymer hydrogels developed in this work using Fourier transform infrared spectroscopy are presented in Fig. 8. Table 2 shows their infrared spectral characteristics in comparison with literature data for GN and SA biopolymer hydrogels from [27], and for the GN-HANA-H2O biopolymer hydrogel from [28]. Absorption bands in the FTIR spectra in Fig. 8 are given in Table 2 for stretching (ν) and bending (δ) vibrations of the corresponding functional groups based on literature data for gelatin, SA and humic acids [27,28,30,31]. It can be seen that the FTIR spectra of the GN-SA-H2O and GN-SA-HANA-H2O systems are almost similar. Small red and blue shifts in the positions of stretching and bending vibrations occurred due to GN-SA, GN-HANA and SA-HANA interactions similar to those observed in [27,28], in particular, due to a conformational change in the secondary structure of GN and due to the interaction of humic acids with SA. At the same time, in GN-SA biopolymer hydrogels modified with HACA, the FTIR spectra in Fig. 8 show lower absorption of humic acids at a frequency of 2925 cm−1, which is associated with aliphatic C-H bands. Moreover, the weakening of absorption at 2925 cm−1 becomes more noticeable with increasing concentration of HACA in the GN-SA hydrogel. In addition, in biopolymer hydrogels of the GN-SA-HACA-H2O system, the stretching vibrations νC-O and νC-N at a frequency of 1643 cm−1 are reduced in GN-SA-HACA 2.5-H2O and GN-SA-HACA5-H2O biopolymer hydrogels, and in the GN-SA-HACA7.5-H2O biopolymer hydrogel they are completely absent. The reason may be a significant spatial reorientation of molecules in the modificated gelatin-alginate hydrogels due to their non-covalent bonds with HACA, which is associated with a change in the polarization of the aliphatic chains and vibrations νC-O and νC-N arising from the interaction of infrared radiation with dipole moments. This leads to a weakening of the IR active oscillations, clearly visible in Fig. 8 as the decrease in absorption peaks at 2925 and 1643 cm−1.
Figure 8: FTIR spectra of the developed thermosensitive and wound-healing biopolymer hydrogels prepared in this work, compositions of which are presented in Table 1
According to [12,20,27,36], the observed changes in the infrared spectra can be explained by the interaction of NAСA with SA anions to form tight gel networks. In general, the results of Fourier transform infrared spectroscopy are in good agreement with rheological studies on the measurement of kinematic viscosity using a glass viscometer in biopolymer hydrogels of the GN-SA-HANA-H2O system, as well as with a sharp increase in the softening temperature and an extension of the time of the hard-soft transition in biopolymer hydrogels of the GN-SA-HACA-H2O system.
Fig. 9 shows the results of free-swelling absorption measurements of the biopolymer hydrogels developed here, which were carried out to evaluate the ability of the biopolymer hydrogels to absorb exudate in the wound area.
Figure 9: The swelling ability of the developed thermosensitive and wound-healing biopolymer hydrogels containing GN 14 wt. % and SA 6.4 wt. % depending on the content of humic acids HACA and HANA
As can be seen in Fig. 9, even when small concentrations of HACA at the level of 2.5 wt. % were introduced into the GN-SA GN-SA6.4-H2O hydrogel, the swelling decreased from 8.3% to 2.1%. In general, physical connections between calcium ions, including those from humic acids HACA, with GN-SA biopolymer hydrogel molecules, leading to the formation of tight gel networks, manifest themselves in a significant reduction in the swelling ability of GN-SA-HACA 2.5-H2O, GN-SA-HACA5-H2O and GN-SA-HACA7.5-H2O biopolymer hydrogels. When HANA humic acids were added to this GN-SA biopolymer hydrogel at concentrations of 2.5 and 5 wt. %, a slight decrease in free swell absorptive capacity was observed to 6% and 7%, respectively. According to [28,29], this is explained by non-covalent interactions such as H-bonding, as well as hydrophobic and electrostatic interactions between the carbonyl groups of HANA and the hydroxyl groups of hydroxyproline, proline residues and glycine amino moieties of the GN chains, promoting the formation of a tighter gel network. However, as can be seen in Fig. 9, at high HANA concentrations of 7.5 wt. %, the free swelling absorption capacity of GN-SA biopolymer hydrogel significantly increased to 13.6%. According to [29], this was due to the high affinity of HANA for water, which established preferential bonds with water molecules, preventing them from coordinating with GN chains, as a result of which the secondary structure of GN lost its triple helical structure, and the gel network became weaker and larger. In addition, the high swelling ability of HANA, shown in [29], dramatically increased the swelling of the GN-SA biopolymer hydrogel with high HANA content. Overall, according to [36], the absorption capacity of the biopolymer hydrogels in this study is promising for the treatment of moderately and heavily exuding wounds.
Figs. 10 and 11 schematically show the structures of gelatin-alginate hydrogels modified with humic acids HANA and HACA, respectively. Their comparison explains the differences in the rheological properties and thermal stability of the two types of hydrogels by a sharp decrease in the kinematic viscosity and swelling of the hydrogels of the GN-SA-HACA-H2O system due to the egg-box effect. Fig. 12 schematically shows the exchange of Ca2+ ions between HACA and SA during the creation of biopolymer hydrogels of the GN-SA-HACA-H2O system.
Figure 10: Scheme of modification of gelatin-alginate hydrogel with humic acids HANA with creation of hydrogel structure of the GN-SA-HANA-H2O system
Figure 11: Scheme of modification of gelatin-alginate hydrogel with humic acids HACA with creation of hydrogel structure of the GN-SA-HACA-H2O system
Figure 12: Scheme of the exchange of Ca2+ ions between HACA and SA during the creation of biopolymer hydrogels of the GN-SA-HACA-H2O system
Next, the shrinkage of the developed in this work biopolymer hydrogels due to cross-linking and air drying that occurs after application of a wound dressing was assessed to mimic wound contracture and contour deformation associated with traditional wound healing. The results are presented in Table 3 and Fig. 13. Data on volume contraction of the developed biopolymer hydrogels in Table 3 predominantly reflect intense evaporation of water from biopolymer hydrogels during 48 h of observation, which was very slightly accelerated by both humic acids.
Figure 13: Photographs of experiments to observe shrinkage over two days of developed thermosensitive and wound-healing biopolymer hydrogels, the compositions of which are presented in Table 1, carried out to determine the degree of compression of their area (surface compression) and volume (volumetric compression) due to air drying and gel cross-linking reactions
Photographs in Fig. 13 confirmed a little reduction of the surface of all GN-SA biopolymer hydrogels, including those modified with HANA and HACA humic acids. The surface contraction of these biopolymer hydrogels, shown in Table 3, calculated using Eq. (2), was greater than 91.9%, confirming that for all biopolymer hydrogel samples, the surface shrinkage was less than 8.9%. The volume contraction, calculated using Eq. (3), was at the level of 70% and decreased slightly with the addition of humic acids. Thus, in accordance with [36], a slight increase in the shrinkage of biopolymer hydrogel surfaces due to cross-linking of GN-SA networks when modifying biopolymer hydrogels with humic acids, especially HACA, should not cause problems when using dressings for the treatment of wounds.
Herein we present innovative biopolymer hydrogel systems that synergistically combine the outstanding properties of natural molecular polymers GN and SA, such as low cost, non-toxicity, biocompatibility, biodegradability and non-immunogenicity, with the antioxidant, antibacterial and anti-inflammatory properties of humic acids. It has been shown that modification of GN-SA biopolymer hydrogel with different concentrations of two types of humic acids makes it possible to control the rheological properties of biopolymer hydrogels over a wide range. In particular, in the systems GN-SA-HANA2.5-H2O and GN-SA-HANA5-H2O it is possible to obtain a gel-sol transition temperature close to the physiological temperature of 37°C, which is important for drug delivery in the treatment of wounds. By changing the type and concentration of humic acids in the GN-SA hydrogel, it is possible to regulate the softening time of the gel on the human body in the range from 6 to 20 min, which provides the possibility of controlled prolonged delivery of drugs. Due to ion exchange in biopolymer hydrogels containing humic acids HACA between Ca2+ and Na+ from SA, it was possible to obtain viscous and thermostable biopolymer hydrogels of the GN-SA-HACA-H2O system. It has been shown that the swelling ability of GN-SA biopolymer hydrogels modified with humic acids can be adapted for the treatment of moderately and severely exuding wounds. A slight increase in the shrinkage of biopolymer hydrogel surfaces due to cross-linking of GN-SA networks when modifying biopolymer hydrogels with humic acids, especially HACA, should not cause problems of wound contracture and contour deformations when using dressings for wound healing.
The next step in our research will be to carefully select an appropriate terminal sterilization method, since sterilization of biopolymer hydrogels is challenging due to the well-known sensitivity of this type of material to common sterilizing agents such as heat and radiation. At the same time, terminal sterilization of composite biomaterials is critical for their use in wound healing. It is necessary to obtain regulatory approval and safely proceed to clinical trials in which we will evaluate the biocompatibility of the prepared thermosensitive hydrogels in vivo.
Acknowledgement: The authors sincerely thank the editors of the Journal of Renewable Materials for exemption from APC.
Funding Statement: The authors received no specific funding for this study.
Author Contributions: The authors confirm contribution to the paper as follows: Conceptualization: Denis Miroshnichenko, Vladimir Lebedev, Natalja Klochko; software: Sergey Petrushenko; validation: Denis Miroshnichenko, Olena Bogoyavlenska, Аnzhela Olkhovska; formal analysis: Аnna Cherkashina; investigation: Katerina Lebedeva, Vladimir Lebedev; resources: Liudmyla Maloshtan; data curation: Sergey Petrushenko; writing—original draft preparation: Natalja Klochko; writing—review and editing: Ihor Hrubnyk; visualization: Sergey Petrushenko. All authors reviewed the results and approved the final version of the manuscript.
Availability of Data and Materials: Data can be obtained from the corresponding author upon request.
Ethics Approval: The research does not involve the study of humans, animals, medical records and/or human tissue and teeth.
Conflicts of Interest: The authors declare that they have no conflicts of interest to report regarding the present study.
References
1. Phiri R, Sanjay MR, Siengchin S, Oladijo OP, Dhakal HN. Development of sustainable biopolymer-based composites for lightweight applications from agricultural waste biomass: a review. Adv Ind Eng Polym Res. 2023;6:436–50. doi:10.1016/j.aiepr.2023.04.004. [Google Scholar] [CrossRef]
2. Lan L, Ping J, Xiong J, Ying Y. Sustainable natural bio-origin materials for future flexible devices. Adv Sci. 2022;9(15):2200560–34. doi:10.1002/advs.202200560. [Google Scholar] [PubMed] [CrossRef]
3. Wang Z, Ma Z, Sun J, Yan Y, Bu M, Huo Y, et al. Recent advances in natural functional biopolymers and their applications of electronic skins and flexible strain sensors. Polymers. 2021;13:813–18. doi:10.3390/polym13050813. [Google Scholar] [CrossRef]
4. Baranwal J, Barse B, Fais A, Delogu G, Kumar A. Biopolymer: a sustainable material for food and medical applications. Polymers. 2022;14:983–22. doi:10.3390/polym14050983. [Google Scholar] [CrossRef]
5. Li X, Ding C, Li X, Yang H, Liu S, Wang X, et al. Electronic biopolymers: from molecular engineering to functional devices. Chem Eng J. 2020;397:125499–17. doi:10.1016/j.cej.2020.125499. [Google Scholar] [CrossRef]
6. Lavania S, Mehta J, Bhardwaj V, Tripathi A, Gupta NV, Gupta P. Biocomposites: prospects and manifold applications for human and environmental sustainability. ECS J Solid State Sci Technol. 2023;12(3):037002. doi:10.1149/2162-8777/acbe17. [Google Scholar] [CrossRef]
7. Islam MZ, Sarker ME, Rahman MM, Islam MR, Ahmed A, Mahmud MS, et al. Green composites from natural fibers and biopolymers: a review on processing, properties, and applications. Adv Ind Eng Polym Res. 2022;41:526–57. doi:10.1177/07316844211058708. [Google Scholar] [CrossRef]
8. Montoya C, Du Y, Gianforcaro AL, Orrego S, Yang M, Lelkes PI. On the road to smart biomaterials for bone research: definitions, concepts, advances, and outlook. Bone Res. 2021;9:12–6. doi:10.1038/s41413-020-00131-z. [Google Scholar] [PubMed] [CrossRef]
9. Uttayarat P, Chiangnoon R, Eamsiri J, Senawongse W. Processing and characterization of antibacterial hydrogel sheet dressings composed of poly(vinyl alcohol) and silk fibroin for wound healing application. Walailak J Sci Tech. 2019;16(5):349–59. doi:10.48048/wjst.2019.6292. [Google Scholar] [CrossRef]
10. Xie Y, Gao P, He F-F, Zhang C-Y. Application of alginate-based hydrogels in hemostasis. Gels. 2022;8:109–21. doi:10.3390/gels8020109. [Google Scholar] [CrossRef]
11. Abou-Okeil A, Fahmy HM, El-Bisi MK, Ahmed-Farid OA. Hyaluronic acid/Na-alginate films as topical bioactive wound dressings. Eur Polym J. 2018;109:101–9. doi:10.1016/j.eurpolymj.2018.09.003. [Google Scholar] [CrossRef]
12. Ahmad A, Mubarak N, Jannat FT, Ashfaq T, Santulli C, Rizwan M, et al. A critical review on the synthesis of natural sodium alginate based composite materials: an innovative biological polymer for biomedical delivery applications. Processes. 2021;9:137–27. doi:10.3390/pr9010137. [Google Scholar] [CrossRef]
13. Nosrati H, Heydari M, Khodaei M. Cerium oxide nanoparticles: synthesis methods and applications in wound healing. Mater Today Bio. 2023;23:100823–25. [Google Scholar] [PubMed]
14. Ndlovu SP, Ngece K, Alven S, Aderibigbe BA. Gelatin-based hybrid scaffolds: promising wound dressings. Polymers. 2021;13:2959–31. [Google Scholar] [PubMed]
15. Nosrati H, Khodaei M, Alizadeh Z, Banitalebi-Dehkordi M. Cationic, anionic and neutral polysaccharides for skin tissue engineering and wound healing applications. Int J Biol Macromol. 2021;192:298–322. [Google Scholar] [PubMed]
16. Banerjee H, Suhail M, Suhail M, Ren H. Hydrogel actuators and sensors for biomedical soft robots: brief overview with impending challenges. Biomimetics. 2018;3:15–41. doi:10.3390/biomimetics3030015. [Google Scholar] [PubMed] [CrossRef]
17. Shi Q, Liu H, Tang D, Li Y, Li XJ, Xu F. Bioactuators based on stimulus-responsive hydrogels and their emerging biomedical applications. NPG Asia Mater. 2019;11(1):64–21. doi:10.1038/s41427-019-0165-3. [Google Scholar] [CrossRef]
18. Chen L, Liu F, Abdiryim T, Liu X. Stimuli-responsive hydrogels as promising platforms for soft actuators. Mater Today Phys. 2024;40:101281–22. doi:10.1016/j.mtphys.2023.101281. [Google Scholar] [CrossRef]
19. Schwaller D, Mésini PJ. Beyond sol-gel: molecular gels with different transitions. Gels. 2023;9:273–36. doi:10.3390/gels9040273. [Google Scholar] [PubMed] [CrossRef]
20. Goudoulas TB, Germann N. Phase transition kinetics and rheology of gelatin-alginate mixtures. Food Hydrocoll. 2017;66(5):49–60. doi:10.1016/j.foodhyd.2016.12.018. [Google Scholar] [CrossRef]
21. Lebedeva K, Cherkashina A, Voronkin A, Lebedev V, Klochko N, Masikevych A. Design and researching smart biologically active polymeric hydrogel transdermal nanomaterial’s. In: 2023 IEEE 4th KhPI Week Adv Technol (KhPIWeek), 2023; Kharkiv, Ukraine; p. 1–5. doi:10.1109/KhPIWeek61412.2023.10312985. [Google Scholar] [CrossRef]
22. Miroshnichenko D, Lebedeva K, Cherkashina A, Lebedev V, Tsereniuk O, Krygina N. Study of hybrid modification with humic acids of environmentally safe biodegradable hydrogel films based on hydroxypropyl methylcellulose. C. 2022;8:71–10. doi:10.3390/c8040071. [Google Scholar] [CrossRef]
23. Lebedeva K, Cherkashina А, Tykhomyrova T, Moiseev V, Lebedev V. Research of biologically active polymeric hydrogel transdermal materials. In: Ivanov V, Pavlenko I, Liaposhchenko O, Machado J, Edl M, editors. Advances in design, simulation and manufacturing VI. Cham: Springer; 2023. doi10.1007/978-3-031-32774-2_21. [Google Scholar] [CrossRef]
24. Hernández R, Sacristan J, Mijangos C. Sol/gel transition of aqueous alginate solutions induced by Fe2+ cations. Macromol Chem Phys. 2010;211:1254–60. [Google Scholar]
25. Cao L, Lu W, Mata A, Nishinari K, Fang Y. Egg-box model-based gelation of alginate and pectin: a review. Carbohydr Polym. 2020;242:116389. [Google Scholar]
26. Ursini O, Angelini R, Franco S, Cortese B. Understanding the metal free alginate gelation process. H RSC Adv. 2021;11:34449–55. [Google Scholar]
27. Derkach SR, Voron’ko NG, Sokolan NI, Kolotova DS, Kuchina YA. Interactions between gelatin and sodium alginate: UV and FTIR studies. J Disp Sci Tech. 2020;41:690–8. [Google Scholar]
28. Venezia V, Avallone PR, Vitiello G, Silvestri B, Grizzuti N, Pasquino R, et al. Adding humic acids to gelatin hydrogels: a way to tune gelation. Biomacromolecule. 2022;23(1):443–53. doi:10.1021/acs.biomac.1c01398. [Google Scholar] [PubMed] [CrossRef]
29. Gomes de Melo BA, Motta FL, Santana MHA. Humic acids: structural properties and multiple functionalities for novel technological developments. Mat Sci Eng C. 2016;62:967–74. doi:10.1016/j.msec.2015.12.001. [Google Scholar] [CrossRef]
30. Nardi S, Schiavon M, Francioso O. Chemical structure and biological activity of humic substances define their role as plant growth promoters. Molecules. 2021;26:2256–1–2256–20. doi:10.3390/molecules26082256. [Google Scholar] [PubMed] [CrossRef]
31. Calderín García A, Ambrosio de Souza LG, Pereira MG, Castro RN, García-Mina JM, Zonta E, et al. Structure-property-function relationship in humic substances to explain the biological activity in plants. Sci Rep. 2016;6:20798. doi:10.1038/srep20798. [Google Scholar] [PubMed] [CrossRef]
32. Peña-Méndez EM, Havel J, Patočka J. Humic substances - compounds of still unknown structure: applications in agriculture, industry, environment, and biomedicine. J Appl Biomed. 2005;3:13–24. doi:10.32725/jab.2005.002. [Google Scholar] [CrossRef]
33. Rashad M, Hafez M, Popov AI. Humic substances composition and properties as an environmentally sustainable system: a review and way forward to soil conservation. J Pl Nutr. 2022;45(7):1072–122. doi:10.1080/01904167.2021.2005801. [Google Scholar] [CrossRef]
34. Kloster NS, Brigante ME, Zanini GP, Avena MJ. Aggregation kinetics of humic acids in the presence of calcium ions. Coll Surf A: Physicochem, Eng Aspects. 2013;427:76–82. doi:10.1016/j.colsurfa.2013.03.030. [Google Scholar] [CrossRef]
35. Zou H, Long Y, Shen L, He Y, Zhang M, Lin H. Impacts of calcium addition on humic acid fouling and the related mechanism in ultrafiltration process for water treatment. Membranes. 2022;12:1033. [Google Scholar] [PubMed]
36. Pribadi Perkasa D, Erizal E, Purwanti T, Tontowi AE. Characterization of semi-interpenetrated network alginate/gelatin wound dressing crosslinked at sol phase. Indones J Chem. 2018;18:367–75. doi:10.22146/ijc.25710. [Google Scholar] [CrossRef]
Appendix A
Cite This Article
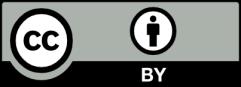
This work is licensed under a Creative Commons Attribution 4.0 International License , which permits unrestricted use, distribution, and reproduction in any medium, provided the original work is properly cited.