Open Access
ARTICLE
Optimisation Strategy of Carbon Dioxide Methanation Technology Based on Microbial Electrolysis Cells
College of Energy and Mechanical Engineering, Shanghai University of Electric Power, Shanghai, 200090, China
* Corresponding Author: Xiaoxiao Yan. Email:
(This article belongs to the Special Issue: Renewable Biomass as a Platform for Preparing Green Chemistry)
Journal of Renewable Materials 2023, 11(7), 3177-3191. https://doi.org/10.32604/jrm.2023.027749
Received 13 November 2022; Accepted 13 January 2023; Issue published 05 June 2023
Abstract
Microbial Electrolytic Cell (MEC) is an electrochemical reaction device that uses electrical energy as an energy input and microorganisms as catalysts to produce fuels and chemicals. The regenerative electrochemical system is a MEC improvement system for methane gas produced by biological carbon sequestration technology using renewable energy sources to provide a voltage environment. In response to the influence of fluctuating disturbances of renewable electricity and the long system start-up time, this paper analyzes the characteristics of two strategies, regulating voltage parameter changes and activated sludge pretreatment, on the methane production efficiency of the renewable gas electrochemical system. In this system, the methane production rate of regenerative electrochemical system is increased by 1.4 times through intermittent boosting start-up strategy; based on intermittent boosting, the methane production rate of regenerative electrochemical system is increased by 2 times through sludge pyrolysis pretreatment start-up strategy, and the start-up time is reduced to 10 days. Meanwhile, according to the simulation test results of power input fluctuation and intermittency, the stability standard deviation of its system operation is 75% of the original one, and the recovery rate is about 1 times higher. This study can provide a theoretical basis and technical reference for the early industrial application of microbial CO2 methanation technology based on renewable energy.Keywords
In 2020 General Secretary Xi proposed at the 75th United Nations General Assembly that China would ensure that it would achieve peak carbon emissions by 2030 and strive to become carbon neutral by 2060 [1]. This commitment has given a strong impetus to CO2 reduction efforts in China and globally. As a resource, the production of chemicals and fuels from CO2 through the use of physical, chemical and biological technologies is one of the most effective ways to control and reduce CO2 emissions. As CO2 is a chemically inert and thermodynamically stable gas, the key to its resource conversion is the effective activation of CO2, which breaks down its chemical structure and transforms it into other usable substances. At present, there are two main types of CO2 resource utilization: physical utilization and chemical utilization, and the main methods include biological fixation, electrochemical reduction, photocatalytic conversion and catalytic hydrogenation reduction. After a long period of research and progress, electrochemical reduction technology is an ideal way to reduce CO2 to high value-added products with low energy consumption, while the choice of catalysts for redox reactions using biocatalyst has the advantages of environmental friendliness, mild reaction conditions (atmospheric pressure and room temperature) and high product selectivity [2]. Therefore, Biological carbon sequestration, as one of the highly promising CO2 capture and utilization technologies, has gained much attention in recent years.
Microbial electrocatalytic reduction of CO2 to produce methane system (MEC) can produce hydrogen or reduce CO2 to produce methane by treating wastewater through the microbial electrocatalytic process. This, this system coupled energy, municipal environment, biology, and other fields. The, the process operation is simple, energy saving, low investment cost, and operation cost. The, environmentally friendly methane gas application technology field is more mature and, has great potential for development [3]. Microbial electrocatalytic reduction of CO2 to produce methane is a highly efficient and low-cost carbon-neutral energy storage technology that can store excess renewable energy and convert CO2 into easily stored methane through the catalytic action of anaerobic microorganisms in wastewater sludge. It, which not only effectively reduces CO2 emissions, but also converts them it into another important energy material, and treats organic wastewater and organic solid waste. The conversion of CO2 into easily stored methane is not only effective in reducing CO2 emissions but also in converting it into another important energy source and treating organic wastewater and solid waste. However, there are still many challenges that need to be solved, and the main technical bottlenecks are the need to significantly improve the operational performance and the lack of practical technology research. The operational performance of the dual-chamber MEC methanogenic system is mainly limited by the biocathode environment, which has a long start-up time, low current density and methane production rate, and poor operational stability. For example, the low methane production rates reported in the literature for CO2 methanogenic systems based on microbial electrolysis cells and the difficulty in rapidly enriching efficient and electrically active methanogenic biofilms at the cathode [4]. In addition, since the electrical input is the only source of energy to produce the reduction equivalent at the cathode, one of the greatest challenges for this technology is to improve its stability in the face of fluctuations and interruptions in the electrical input from renewable energy sources. Instability in power input may lead to changes in the structure and metabolic pathways of the biocathode microflora, resulting in reduced methane production [5]. Therefore, a large number of studies have been conducted to review the factors affecting the performance of microbial electrolytic cell CO2 methanogenesis systems, such as: electrochemical start-up strategies, cathode materials and reactor structures, pretreatment methods, voltage, temperature, pH, etc., to clearly identify the influence of these factors on the promotion of efficient microbial methane synthesis, with the aim of finding effective and easy-to-implement electrochemical start-up strategies to overcome these problems [6].
Cheng et al. set a potential of −0.5 V by the constant potential method and spent approximately one month to complete the initiation of a methanogenic biocathode in a single chamber EMG reactor with a methane production rate of 0.034 m3m−3d−1 [7]; Riedl et al. found that a continuous change in potential from −0.6 to 0.4 V with a continuous change in scan rate of (5 mVs−1) resulted in an increase in bioelectrode current density and turnover, mainly due to the enrichment of a more efficient electroactive biofilm [4]; Li et al. converted the electrode potential by polarity reversal from bioanode of −0.4 to −0.7 V at the cathode by polarity reversal. This was found to promote direct electron transfer and increased biomass in the biofilm, thereby promoting direct electron transfer and increased biomass in reducing the start-up time by approximately 40% [8]. Wang et al. found that intermittent power supply was more beneficial in promoting methane production than the commonly used continuous power supply, mainly because the intermittently applied electric field increased the biofilm charge storage capacity and electron mobility [9]. Xiang et al. operated at different voltages microbial electrosynthesis systems using bioenergy from wastewater to convert carbon dioxide to acetic acid, with high biomass on the anode and cathode surfaces of the bipolar membrane (BPM) and the relative abundance of acetic acid bacteria in the cathode [10]. Fu et al. applied a resting potential of 0.5 V to SHE in a two-chamber reactor and increased CH4 productivity of the biocathode by approximately 6-fold after 160 h of incubation, showing that the ability of the biocathode to catalyze electrogenic methanogenesis via direct electron transfer was enhanced during the adaptation process [11]. Borole et al. measured changes in anode, cathode, and solution/membrane impedance during enrichment of anodic microbial consortia and found that biological systems have the unique ability to reduce the electron transfer resistance of MFCs and their potential for stable energy production over long periods of time [12]. In addition, some researchers have tried to artificially lower the cathode potential during start-up to up-regulate the input power and found that dense biofilms with high metabolic and electrical activity were enriched at the cathode, allowing the biocathode to maintain stable coulombic efficiency despite fluctuations in electrode potential or current [13–15]. Rossi et al. also found that up-regulating the current was able to reduce the charge transfer impedance and diffusion impedance, fundamentally altering the performance of the bioelectrode [16]. In summary, the performance and stability of the biocathode can be significantly improved by adjusting the cathodic potential and current.
In order to speed up the anaerobic digestion of activated sludge and to shorten the digestion reaction time, a pre-treatment of the sludge is necessary. Zhang et al. used a high-pressure homogenization method to pretreat residual sludge and investigated the effects of homogenization pressure, homogenization cycle, and total sludge solids concentration on the sludge cracking rate [17]. Dohányos et al. used centrifugal lysis for anaerobic digestion of residual sludge after pretreatment for methane production, with methane yields ranging from 8.1% to 86.4% for different properties of sludge [18]. Jeong et al. used high-efficiency pulse technology for pretreatment of residual sludge, and the values of SCOD/TCOD and the content of extracellular polymers were increased compared to the untreated sludge by 4.5-fold and 6.5-fold [19]. Baier et al. increased the percentage of SCOD from 5% to 47% after pretreatment of the residual sludge using the rotating grinding ball method [20]. Boungier et al. used pyrolysis to pretreat the residual sludge, and the results showed that the cracking rate, protein and carbohydrate release rate, and methane yield of the sludge were more beneficial under 190°C compared to 135°C [21]. Feng et al. investigated the effect of heat treatment on the physical properties and dewatering properties of municipal residual sludge, and the results showed that: after heat treatment, the results showed that the dewatering properties, particle size and viscosity of the sludge decreased after heat treatment [22]. Tang et al. investigated the effect of three different garden wastes on the methanogenic effect of anaerobic digestion of kitchen waste using freeze-thaw pretreatment and found that freeze-thaw pretreatment could increase the gas yield by 25.0% and 85.4%, respectively [23]. Tang et al. performed anaerobic fermentation of mixed sludge from primary and secondary settling tanks after freeze-thaw pretreatment to produce methanogenic firing, and the gas yield was 1.31 m3/kgVS, which was 1.5 times higher compared to the original sludge [24]. In summary, sludge pretreatment technology can effectively promote the release of organic matter from sludge flocs and microbial cells into the liquid phase, improve sludge’s biochemical properties of sludge, accelerate the rate of sludge digestion rate, and increase the energy recovery rate. In practical engineering applications, the sludge should be pretreated according to the nature of the sludge, and the appropriate pretreatment method should be selected.
According to the literature review, better operational performance can be achieved when the biocathode has a variable potential and current, and its two electrochemical parameters are able to respond to changes in cathode kinetics during start-up and change simultaneously, but further studies are lacking. Therefore, this paper proposes an intermittent boosting strategy to increase the electrical output based on simultaneous changes in current, and potential is expected to further improve the methane production performance and its stability of renewable electrochemical systems; meanwhile, among the many foundations of sludge pre-treatment, there is a lack of a certain degree of research on the way in which pre-treatment technologies combine voltage and current influencing factors for incubation [25]. Therefore, this paper uses three sets of comparative experimental start-ups of reduced CO2 methanogenic systems using constant voltage start-up and intermittent boost start-up [26] and intermittent boost start-up after sludge pyrolysis treatment to investigate the changes in methane production rate, pH of the cathode chamber solution, current density, and the stability and recovery rate analysis of operation under fluctuating and intermittent power input under the three start-up methods. The aim is to reveal the effects of intermittent boost start-up strategies and activated sludge pyrolysis pretreatment processes on the performance of CO2 biomethanation, thus providing a theoretical basis and technical reference for the application of CO2 methanation engineering based on microbial electrolysis cells.
This paper presents the following innovations: (1) For the first time, the intermittent boosting strategy is combined with sludge thermal treatment to analyze the biofilm formation and enrichment characteristics of bacterial strains, which in turn affects the performance and stability of methane production in regenerative gas electrochemical systems. (2) The methanogenic performance and stability of the regenerative electrochemical system is characterized by simulating fluctuations in power input and intermittency to further validate the practical application results.
2 Experimental Materials and Methods
The reactor used in this experiment is an H-type double-chamber reactor, the main body of which is made of Plexiglas material and Its physical diagram is shown in Fig. 1. Three sets of control experiments were set up as shown in Table 1. three sets of single-chamber type H-type double-chamber reactors of 100 mL, the effective volume of both cathode and anode chambers is 155 mL, and the cathode and anode chambers are filled with 110 mL and 120 mL of electrolyte solution (PH = 7), respectively. The first set of two-chamber reactors provided an applied voltage of 0.8 v and was inoculated with 35 g of centrifuged sludge; the second set of two-chamber reactors provided a changing applied voltage and was inoculated with about 35 g of centrifuged sludge and the third set of two-chamber reactors provided a changing applied voltage and was inoculated with about 35 g of sludge that had been pretreated by pyrolysis and then centrifuged to extract water. The anode was made of platinum sheet electrode, and the reference electrode was Agcl electrode. The cathode was made of a 1 mm diameter titanium wire connected to a high-purity graphite felt.
Figure 1: Three sets of reactors during start-up
2.2 Pretreatment Types and Conditions
The thermal pretreatment method refers to the heating of the residual sludge, where the microbial cells are ruptured by the pressure difference generated in the heat process, and the organic matter inside the cells is released and rapidly hydrolyzed and dissolved under the action of high temperature. The temperature range of sludge heat treatment is generally in the range of 60°C–270°C, and the most commonly used temperature range is 60°C–180°C. Take 38.76 g of sludge in a beaker, add 1.2979 g of NaOH solids, stir well, and then place the beaker in a water bath at 65°C for 30 min to complete the pretreatment. The purpose of adding NaOH solid is to increase the degree of hydrolysis of sludge, reduce the temperature tolerance of biological cell walls in sludge, and promote the efflux of cellular organism inclusions, which improves good conditions for accelerating the process of anaerobic digestion of colonies.
The activated sludge was treated by pyrolysis, and after centrifugation at 5000 r/min for 10 min, the sludge obtained was 35.5624 g, and finally, the treated sludge was put into the reactor for methane production experiment.
Three start-up strategies, constant voltage, intermittent boosting, and intermittent boosting after pyrolysis pretreatment, were used to study the effect of the start-up process of the microbial electrolysis cell system on the operational performance of the methanogenic biocathode. The constant voltage start-up group was set at 0.8 v; the intermittent boost start-up group was increased from 0.2 to 0.8 v, and the voltage was increased by 0.2 v at an interval of 24 h; the intermittent boost start-up group after pyrolysis pretreatment was operated in the same way as the second group. Other conditions were kept constant, and after normal operation of the reactor, 1 ml of gas was extracted from the sampling place with a sampling needle at every measurement interval of 24 h and injected into the gas phase spectrometer for gas detection.
At the same time, aeration treatment was carried out, and CO2 with a flow rate of 0.3 L/min was passed into the cathode chamber for aeration, in which the aeration time was 30 min and the period was 24 h. During the period, the appropriate amount of cathode chamber solution was excluded using the air pressure difference for PH measurement, and the solution was reinjected into the cathode chamber at the end of the test.
2.4 Analysis and Calculation Methods
After the MEC reactor started operation, 1 ml of gas produced in the cathode chamber was removed every 24 h with a feeder and injected into a gas chromatograph (GC9800, Shanghai Tech Chromatography Instruments Co., Ltd., China) for detection. The different gases were distinguished according to their peak times, and the yields of each gas component were calculated from the peak areas. The equation for the production rate of methane gas is shown below:
Among them, VCH4 is the volume change of methane produced by the reactor (m3) and T is the time of reactor operation (S).
During the operation of the H-type dual-chamber reactor, the current variations of three different starting strategies were recorded in real time with a data logger, and the desired current values were selected from the logger at the end of the experiment. During the operation of the MES reactor, the current density is the magnitude of the current generated per unit area of the cathode electrode and is calculated as follows:
Among them, I is the circuit current (A), and A is the surface area of the cathode graphite felt (m2).
During the experiment, the pH value of the cathode chamber solution was measured every 48 h with a pH meter (PHS-3E type, Shanghai Yidian Scientific Instruments Co., Ltd., China). This was done by withdrawing a small amount of cathode chamber solution with a syringe before CO2 aeration at the end of each experimental cycle, and then measuring and recording it with a pH meter, and re-injecting the withdrawn solution into the cathode chamber after the measurement.
2.4.3 Stability Simulation Test
The effect of fluctuations in electrical power input on the operational stability of the regenerative gas electrochemical system was simulated using an electrochemical workstation by increasing the current density from 0.5 to 5 A/m2 (0.5 A/m2 every 2 h, with re-exposure to CO2 for about half an hour at the end of each current density plateau).
Intermittent power input was simulated by interrupting the power input for 20, 40, 60 and 80 h after 2 h of stable operation, and then reconnecting the power to test the system’s recovery rate of the system. The recovery time was defined as the time to restore the methane production rate to 90% of that before the power outage after the power input was restored, which was used to evaluate the recovery performance of the regenerative gas electrochemical system.
3 Experimental Results and Discussion
3.1 Comparative Analysis of Methane Production Performance under Different Conditions
3.1.1 Analysis of Methane and Hydrogen Production Rates under Different Conditions
The start-up process of the three groups of biocathodes is shown in the Fig. 2a. After using constant voltage start-up (0.8 v), the methane production rate of the cathodes increased slowly throughout the start-up period, and the methane production rate was stable at 6.5 ± 0.03 m3d−1 after 15 days. After using intermittent boost start (0.2–0.8 v), the methane production rate of the cathode increased faster in 0–15 days compared with the constant voltage group, and also the methane production rate was stable at 15.66 ± 0.02 m3d−1 after 15 days, and the start-up time of the methanogenic biocathode was about 15 days. After using activated sludge after heat treatment with intermittent boost start (Pretreatment, 0.2–0.8 v), methane production gradually increased from 1 to 5 days, and methane production rate continued to accelerate from 5 to 10 days, and methane production rate stabilized at 20.18 ± 0.01 m3d−1 after 10 days methane production start time was about 10 days.
Figure 2: (a) Methane production rates for the three groups of experiments. (b) Hydrogen production rates for the three sets of experiments
The methane production of the cathode in the constant voltage start group and intermittent boost start group increased continuously during the start and tended to be stable after 15 days and the methane production of cathode in the intermittent boost start group after activated sludge was heat treated increased continuously during the start and tended to be stable after 10 days and the start time of biological cathode was significantly shortened after the activated sludge was heat treated; meanwhile, the methane production of activated sludge after heat treatment in The methanogenic rate of the intermittent boost start group was stable at 20.18 ± 0.01 m3d−1 about three times higher than that of the constant voltage start group. It is inferred that the methanogenic bacteria in the sludge rupture under the effect of pressure difference generated during the heat treatment, and the organic matter inside the cells is released, which is rapidly hydrolyzed and dissolved under the effect of high temperature, which is favorable to the formation of methanogenic bacteria biofilm on the cathode surface. The methanogenic rate of the intermittent boost start group was stable at 15.66 ± 0.02 m3d−1, which was 2.4 times higher than that of the constant voltage start group, and the cathode was enriched with dense methanogenic bacteria biofilm with high metabolic and electrical activity by adjusting the cathode potential and current [13], while the constant voltage start group, in which the hydrogen-producing bacteria grew more vigorously at the beginning of the start, consumed excessive reduction equivalents, and the shear force generated by the hydrogen bubbles had an adverse effect on The shear force generated by the hydrogen bubbles also had a negative effect on biofilm attachment [27–29].
The process of H2 production from the three groups of biocathodes is shown in the Fig. 2b after starting with constant voltage, the amount of hydrogen produced by the biocathodes increased continuously from day 0 to day 5, and decreased continuously with the gradual increase of methane from day 5 to day 10, and the amount of hydrogen produced was stable at 5.13 m3d−1 after day 15. After the start-up of the activated sludge with heat treatment, the hydrogen production from the biocathode increased slowly from day 0–5 and decreased from day 5–10, stabilizing at 3.13 m3d−1 after day 15.
The production of by-product H2 is unavoidable for mixed strain inoculated biocathodes, and the variation of by-product H2 production during the three groups of the biocathode start-up is shown in the graph. The peak H2 production in the start-up phase (day 5) was six times higher in the constant voltage start-up group compared to the intermittent boost start-up group, and 2.5 times higher in the stabilization phase. It can be seen that this method significantly reduces the hydrogen production during the bio-initiation and stabilization phases while increasing the methane production from the biocathode during the same period. So the intermittent ramp-up strategy improves the product selectivity of the methanogenic biocathode, which is probably due to the incomplete enrichment of the methanogenic biofilm in the constant voltage start-up group, resulting in a lower utilization of CO2 and reducing equivalents and the escape of H2 before it is utilized by methanogenic bacteria utilize it in time. The peak H2 production rate in the start-up phase and the steady-state H2 rate in the intermittent boost start-up group after thermal treatment of activated sludge were slightly higher by about 1.1 m3d−1 compared to the intermittent boost start-up group, it is possible that not only the methanogenic bacteria are more active in the activated sludge pretreated by pyrolysis, but also other microorganisms (e.g., hydrogen-producing bacteria) are more active in reproduction and metabolism.
In previous studies of methane production from CO2 reduction in bioelectrochemical systems, hydrogen and methane have always co-existed in a single system therefore, to increase the efficiency of methane production, the amount of hydrogen produced must be minimised.
3.1.2 Analysis of the Variation of PH Values under Different Conditions
The pH of a solution is a key factor in the growth and metabolism of microorganisms. Therefore, it is essential to control the pH in solution. As shown in Fig. 3, the pH of the medium solution was adjusted to about 7 (phosphate buffer was added) when the medium was prepared before the start of each batch of experiments. The change in pH decreased and then increased in all three experimental groups. The activity of the biological strains was low at the beginning of the start-up phase and the H+ from the anode chamber reached the cathode through the ion exchange membrane but was not yet fully utilised, resulting in a low PH in the cathode chamber. The constant voltage start-up group had a PH value of 6.68 on day 4 (the lowest value) and the intermittent boost start-up group had a PH value of 6.17 on day 4. The reason for this may be that the intermittent boost strategy favours the enrichment of the methanogenic biofilm, with lower H+ utilization and lower H2 content compared to the constant voltage group. In contrast, the activated sludge was heat-treated to a PH value of 6.28 on day 4 for the intermittent boost start-up group, which was pre-treated by pyrolysis to improve the sludge cracking rate, giving the reactor better electrochemical performance and enriching the more intensive hydrogen-producing bacteria biofilm to synthesize lower H2 content at the biocathode. After day 4, the PH of all three sets of experiments increased at an accelerated rate, while the methane production of all three sets of experiments also increased at an accelerated rate. The reason for this may be that with the enrichment of the bioactive biofilm at the biocathode, H+ was used to synthesize methane and the rate at which H+ was utilized in the cathode chamber was much higher than that passed from the anode chamber, so H+ was consumed in large quantities and methane was generated at the cathode leading to a rise in pH of the solution. After the stabilization phase of the three sets of experiments, the pH is still slowly increasing, and their methane production remains essentially constant, which will decrease in the long term. The higher pH may also be one of the reasons for the inhibition of methane production, this is because the optimum pH environment for methanogenic strains is between 7 and 8, and high pH (>8.5) increases hydrogen production, as the alkaline environment induces the formation of an enrichment of the dominant hydrogen-producing strains and the same reduction equivalents are used more for hydrogen production, resulting in lower methane production of the target gas. At the same time the activity of the methanogenic archaea was affected and consequently, the overall reaction equilibrium of the fermentation system was disrupted, severely affecting the activity of the microorganisms and resulting in their inability to efficiently convert H2 and CO2 to CH4 [30]. Therefore, ensuring that the ph of the solution was maintained at a relatively stable level was more conducive to the stable synthesis of methane in the MES.
Figure 3: Solution pH of cathode chamber for three groups of experiments
3.1.3 Analysis of Current Density under Different Conditions
As shown in Fig. 4, the constant voltage starter group showed a sharp and unstable drop in current during the first 4 days of operation when the reactor was connected to voltage, probably due to the rapid loss of protons and electrons from the culture solution after being connected to voltage, with the current density gradually decreasing and slowly stabilizing after the 4th day. The change in methane production was also observed to accelerate from day 4 and then slowly stabilize. It can be seen that the decrease in current is due to the formation of a bio-cathode methanogenic biofilm resulting in an increase in the internal resistance of the bio-electrosynthesis system, as the applied voltage provided is constant.
Figure 4: Variation of current density in three groups of experiments
The intermittent ramp-up starter group also goes through an unstable phase and the current density starts to decrease gradually. However, the voltage in this experimental group changed in stages from small to large, with the current density increasing again after day 10 and then gradually decreasing towards stability. In the microbial electrochemical system, the magnitude of the current density also reflects, to some extent, the rate of electron transfer between the methanogenic bacteria and the electrode [14]. The cathode of the intermittent boost group was probably enriched with highly efficient electroactive biofilm, which reduced the internal resistance of the cathode. The reason why the intermittent boosting strategy results in a lower resistance of the biocathode may be the method’s ability to achieve simultaneous changes in cathode potential and current. By giving the flexibility that these two parameters can be adjusted simultaneously, the intermittent boosting method buffers the drop in potential and current and achieves a relatively constant current during start-up, thus steadily supplying the cathode biofilm with the appropriate reduction equivalents to promote its enriched growth [28]. The current density in the stable phase of the heat-treated activated sludge in the intermittent boost start group was 3.573 A/m2, slightly higher than the current density in the intermittent boost start group. This may be due to the fact that the pyrolysis pretreatment allows for an effective cracking of the activated sludge and a more complete release of organic matter, leaving the methanogenic microorganisms in a dominant position and more active, using a large amount of organic simple small molecules in the system for metabolism, synthesis of their own nutrients and methanogenic activities.
3.2 Stability Simulation Test Analysis
3.2.1 System Stability Testing during Fluctuations in Power Input
The results of the test to characterize the stability of the methanogenic performance of the microbial electrolyzer system through fluctuations in electrical input are shown in Fig. 5. As the input current density I increased from 0.5 to 5 A/m2, the methane production rate of the constant voltage start-up group started to decrease after I reached 3.5 A/m2, when the hydrogen production rate was gradually increasing, while the methane production rate of the intermittent boost start-up group increased continuously from 2.23 m3d−1. The methane production rate of the intermittent boost start group increased continuously from 2.23 to 16.84 m3d−1 and then started to decrease slowly; the methane production rate of the intermittent boost start group increased continuously from 3.34 to 21.47 m3d−1 after the activated sludge was heat-treated, and then also started to decrease slowly. The analysis of the graph shows that the standard deviation of stability of the intermittent boosting group and the intermittent boosting group with sludge heat treatment is 75% of that of the constant voltage group.
Figure 5: Impact of power input fluctuations on system operational performance
The stability standard deviation of the intermittent boost group and the intermittent boost group of sludge heat treatment was 85% of that of the constant voltage group. The intermittent boost starting strategy significantly improved the stability of methane production performance and effectively suppressed the by-product H2 production at high current density input. This may be attributed to the fact that the intermittent boosting strategy induced the construction of multiple extracellular electron transfer pathways for CO2 reduction methanogenesis through cathodic potential changes and increased the supply rate of reduction equivalents through intermittent steps, so that the biofilm could obtain higher electron mobility and electrical activity, thus forming an efficient and stable methanogenic biofilm [13].
Meanwhile, the intermittent step-up start-up strategy of activated sludge after thermal treatment was tested with fluctuation of electric power input, and the change trend was consistent with the intermittent step-up start-up strategy, except that the methanogenic production was high in all stages. The main reason may be that the biological community has been treated by pyrolysis at high temperature and the methanogenic bacteria are more active [31], meanwhile, the efficiency of biofilm using electrons has been enhanced by adjusting the cathode potential in the start-up stage, so that the methanogenic performance and stability of this group can reach the best.
3.2.2 System Stability Testing during Intermittent Power Input
The results of the intermittent test to characterize the stability of the methane production performance of the microbial electrolytic cell system by the power input (20, 40, 60, 80 h power outages in sequence) are shown in Fig. 6. Compared with the constant voltage starter group, the intermittent boost starter group showed a lower drop in methane production per power outage and a faster recovery rate, which was about twice as fast as the constant voltage starter group, and the methane production during the stabilization phase (13.84 m3d−1) was basically the same as that before the power outage (15.66 m3d−1) as the power outage time increased. In contrast, the constant voltage starter group had not only difficulty in recovering the methane rate before power failure after each power failure, but also its methanogenic capacity gradually weakened with the increase of power failure time until the methanogenic rate decreased to 0.43 m3d−1 at 80 h of power failure, because most of the methanogenic strains had lost their activity, and thus the methanogenic biofilm formed by the colony was judged to be basically ineffective in methanogenic function. Thus, the intermittent ramp-up strategy shortens the recovery time after power input interruptions and enhances the stability of the methanogenic performance of the microbial electrolyzer system, allowing it to withstand the adverse effects of power outages and maintain an excellent recovery capability [32]. This may be due to the enrichment of the cathode with highly stable methanogenic biofilm, which has a more stable extracellular electron transfer pathway compared to the constant voltage group and is more resilient to the periodic polarization of the cathode caused by repowering after a power outage [33,34].
Figure 6: Impact of intermittent power input on system operational performance
Compared with the intermittent boost-start group, the activated sludge heat-treated intermittent boost-start group had higher methanogenic production after each power outage and, subsequently higher stabilization phase of about 18.44 m3d−1. Therefore, the heat-treated intermittent boost-start strategy improved the methanogenic performance of its stabilization phase. The heat-treated intermittent boost start strategy enriched biofilms in the start-up phase with more stable metabolic pathways (including energy acquisition and carbon consumption) and a microbial community highly adapted to the cathodic environment.
In this experiment, an H-type dual-chamber MEC reaction system with anaerobic activated sludge from Shanghai Bailonggang Wastewater Treatment Plant as the inoculated sludge and CO2 storage bottle as the sole carbon source was constructed to investigate the effects on the performance of CO2 methanation based on microbial electrolysis cell under three sets of strategies: constant voltage, intermittent boosting and intermittent boosting after sludge thermal treatment, as well as system stability tests under fluctuating and intermittent power input. The study showed that:
(1) The cathode potential, the regulation of current, and the pretreatment method of activated sludge all affect the methane production by CO2 by the cathode-functional microorganisms in the MEC reactor. Under the same conditions, the intermittent boosting strategy cathode enriched dense methanogenic bacteria biofilm with high metabolic and electrical activity, which significantly increased methane production; the sludge pyrolysis treatment on the basis of intermittent boosting accelerated the formation of methanogenic bacteria biofilm on the cathode surface, which not only increased methane production but also significantly shortened the whole start-up time. In this experiment, the cumulative amount of methane material in the constant voltage experimental group, the intermittent boosting experimental group, and the intermittent boosting experimental group with sludge pretreatment were 6.52, 15.66, and 20.18 m3d−1, respectively, and the start-up times were about 15, 15, and 10 days, respectively.
(2) Instability of power input can potentially lead to changes in the structure and metabolic pathways of the biocathode microflora, which can result in reduced methane production. The intermittent boosting strategy reduces the dependence on external energy and carbon sources by building a stable and efficient biofilm. In this experiment, during the power input fluctuation test, the stability standard deviation of its system operation is 75% of that of constant voltage; during the power input intermittent test, the intermittent boost experimental group recovers faster, and its recovery speed is nearly one times higher relative to the constant voltage start-up group.
Funding Statement: This paper is supported by Shanghai Science and Technology Development Fund, China, No. 19DZ1205604.
Conflicts of Interest: The authors declare that they have no conflicts of interest to report regarding the present study.
References
1. Kou, J., Zhang, J. (2021). Who will continue to lead global climate governance after the epidemic-the EU’s decline and fightback. Journal of China University of Geosciences, 21(1), 87–104. [Google Scholar]
2. Wang, Y., Jiang, D., Peng, C. (2013). Advances in electrochemical methods for carbon dioxide fixation. Modern Chemicals, 33(10), 37–41. [Google Scholar]
3. Zhang, Y., Zhang, W. (2010). Progress in research on the integrated use of carbon dioxide. Chemical Engineer, 33(1), 53–55. [Google Scholar]
4. Riedl, S., Brown, R., Esquivel, D., Wichmann, H., Huber, K. (2019). Cultivating electrochemically active biofilms at continuously changing electrode potentials. Chemelectrochem, 6(8), 2238–2247. https://doi.org/10.1002/celc.201900036 [Google Scholar] [CrossRef]
5. Zaiat, M., Gonzalez, E., De, W., Pant, D. (2018). Effect of the electric supply interruption on a microbial electrosynthesis system converting inorganic carbon into acetate. Bioresource Technology, 266, 203–210. https://doi.org/10.1016/j.biortech.2018.06.074 [Google Scholar] [PubMed] [CrossRef]
6. Zeppilli, M., Paiano, P., Villano, M., Majone, M. (2019). Anodic vs cathodic potentiostatic control of a methane producing microbial electrolysis cell aimed at biogas upgrading. Biochemical Engineering Journal, 152, 107393. https://doi.org/10.1016/j.bej.2019.107393 [Google Scholar] [CrossRef]
7. Cheng, S., Xing, D., Call, D., Logan, B. (2009). Direct biological conversion of electrical current into methane by electromethanogenesis. Environmental Science & Technology, 43(10), 3953–3958. https://doi.org/10.1021/es803531g [Google Scholar] [PubMed] [CrossRef]
8. Li, Z., Fu, Q., Kobayashi, H., Xiao, S., Li, J. et al. (2019). Polarity reversal facilitates the development of biocathodes in microbial electrosynthesis systems forbiogas production. International Journal of Hydrogen Energy, 44(48), 26226–26236. https://doi.org/10.1016/j.ijhydene.2019.08.117 [Google Scholar] [CrossRef]
9. Wang, B., Liu, W., Zhang, Y., Wang, A. (2020). Bioenergy recovery from wastewater accelerated by solar power: Intermittent electro-driving regulation and capacitive storage in biomass. Water Research, 175, 115696. https://doi.org/10.1016/j.watres.2020.115696 [Google Scholar] [PubMed] [CrossRef]
10. Xiang, Y., Liu, G., Zhang, R., Lu, Y., Luo, H. (2017). High-efficient acetate production from carbon dioxide using a bioanode microbial electrosynthesis system with bipolar membrane. Bioresource Technology, 233, 227–235. https://doi.org/10.1016/j.biortech.2017.02.104 [Google Scholar] [PubMed] [CrossRef]
11. Fu, Q., Kuramochi, Y., Fukushima, N., Maeda, H., Sato, K. et al. (2015). Bioelectrochemical analyses of the development of a thermophilic biocathode catalyzing electromethanogenesis. Environmental Science & Technology, 49(2), 1225–1232. https://doi.org/10.1021/es5052233 [Google Scholar] [PubMed] [CrossRef]
12. Borole, A., Aaron, D., Hamilton, C., Tsouris, C. (2010). Understanding long-term changes in microbial fuel cell performance using electrochemicalimpedance spectroscopy. Environmental Science & Technology, 44(7), 2740–2744. https://doi.org/10.1021/es9032937 [Google Scholar] [PubMed] [CrossRef]
13. Li, J., Li, Z., Xiao, S., Fu, Q., Kobayashi, H. (2019). Startup cathode potentials determine electron transfer behaviours of biocathodes catalysing CO2 reduction to CH4 in microbial electrosynthesis. Journal of CO2 Utilization, 35, 169–175. https://doi.org/10.1016/j.jcou.2019.09.013 [Google Scholar] [CrossRef]
14. Mao, Z., Sun, Y., Zhang, Y., Ren, X., Lin, Z. (2021). Effect of start-up process using different electrochemical methods on the performance of CO2-reducing methanogenic biocathodes. International Journal of Hydrogen Energy, 46(4), 3045–3055. https://doi.org/10.1016/j.ijhydene.2020.02.002 [Google Scholar] [CrossRef]
15. Liu, D., Roca-Puigros, M., Geppert, F., Caizan-Juanarena, L., Ayudthaya, S. (2018). Granular carbon-based electrodes as cathodes in methane-producing bioelectrochemical systems. Frontiers in Bioengineering and Biotechnology, 6, 78. https://doi.org/10.3389/fbioe.2018.00078 [Google Scholar] [PubMed] [CrossRef]
16. Rossi, R., Logan, B. (2020). Impact of external resistance acclimation on charge transfer and diffusion resistance in bench-scale microbial fuel cells. Bioresource Technology, 318, 123–921. https://doi.org/10.1016/j.biortech.2020.123921 [Google Scholar] [PubMed] [CrossRef]
17. Zhang, Y., Zhang, P., Ma, B. (2012). Sewage sludge disintegration by high-pressure homogenization: A sludge disintegration model. Journal of Environmental Sciences, 24(5), 814–820. https://doi.org/10.1016/S1001-0742(11)60834-6 [Google Scholar] [PubMed] [CrossRef]
18. Dohányos, M., Zábranská, J., Jenícek, P. (1997). Enhancement of sludge anaerobic digestion by using of a special thickening centrifuge. Water Science and Technology, 36 (11), 145–153. https://doi.org/10.2166/wst.1997.0405 [Google Scholar] [CrossRef]
19. Jeong, S., Chung, Y. (2006). Enhanced anaerobic gas production of waste activated sludge pretreated by pxxlse power technique. Bioresource Technology, 97(2), 198–203. [Google Scholar] [PubMed]
20. Baier, U., Schmidheiny, P. (1997). Enhanced anaerobic degradation of mechanically disintegrated sludge. Water Science and Technology, 36(11), 137–141. https://doi.org/10.2166/wst.1997.0404 [Google Scholar] [CrossRef]
21. Bougrier, C., Delgenès, H. (2007). Impacts of thermal pre-treatments on the semi-continuous anaerobic digestion of waste activated sludge. Biochemical Engineering Journal, 34(1), 20–27. https://doi.org/10.1016/j.bej.2006.11.013 [Google Scholar] [CrossRef]
22. Feng, Q., Tan, W., Zhong, N. (2014). Effects of thermal treatment on physical and expression dewatering characteristics of municipal sludge. Chemical Engineering Journal, 247(1), 223–230. [Google Scholar]
23. Tang, X., Wan, S., Zhang, Y. (2012). Influence of garden green wastes addition and freeze-thaw pretreatment on food waste anaerobic digestion efficiency. Journal of Environmental Engineering Technology, 2(6), 512–518. [Google Scholar]
24. Montusiewicz, A., Lebiocka, M., Rożej, A. (2010). Freezing/thawing effects on anaerobic digestion of mixed sewage sludge. Bioresource Technology, 101(10), 3466–3473. [Google Scholar] [PubMed]
25. Liu, H., Grot, S., Logan, B. (2005). Electrochemically assisted microbial production of hydrogen from acetate. Environmental Science & Technology, 39(11), 4317–4320. [Google Scholar]
26. Wang, B., Liu, W., Zhang, Y. (2020). Bioenergy recovery from wastewater accelerated by solar power: Intermittent electro-driving regulation and capacitive storage in biomass. Water Research, 97(2), 115–696. https://doi.org/10.1016/j.watres.2020.115696 [Google Scholar] [PubMed] [CrossRef]
27. Cheng, S., Mao, Z., Sun, Y., Yang, J. (2020). A novel electrochemical oxidation-methanogenesis system for simultaneously degrading antibiotics and reducing CO2 to CH4 with low energy costs. Science of the Total Environment, 750, 141–732. [Google Scholar]
28. Villano, M., Aulenta, F., Ciucci, C., Ferri, T., Giuliano, A. (2010). Bioelectrochemical reduction of CO2 to CH4 via direct and indirect extracellular electron transfer by a hydrogenophilic methanogenic culture. Bioresource Technology, 101(9), 3085–3090. https://doi.org/10.1016/j.biortech.2009.12.077 [Google Scholar] [PubMed] [CrossRef]
29. Cai, W., Liu, W., Zhang, Z., Feng, K., Ren, G. (2018). McrA sequencing reveals the role of basophilic methanogens in a cathodic methanogenic community. Water Research, 136, 192–199. https://doi.org/10.1016/j.watres.2018.02.062 [Google Scholar] [PubMed] [CrossRef]
30. Hao, W. (2018). Study of H2/CO2 biomethanation processes, vol. 43, pp. 185–192. Beijing, China: China University of Petroleum. [Google Scholar]
31. W, W., Luo, H., Liu, G. (2013). Electricity-producing colonies in the treatment of milk wastewater using microbial electrolysis cells and relationship between hydrogen production performance. Microbiology Bulletin, 40(11), 2075–2082. [Google Scholar]
32. Molognoni, D., Bosch-Jimenez, P., Rodriguez-Alegre, R., Mari-Espinosa, A., Licon, E. (2018). How operational parameters affect electromethanogenesis in a bioelectrochemical power-to-gas prototype. Frontiers in Energy Research, 8, 174. [Google Scholar]
33. Flemming, H., Wingender, J., Szewzyk, U., Steinberg, P., Rice, S. (2016). Biofilms: An emergent form of bacterial life. Nature Reviews Microbiology, 14(9), 563–575. https://doi.org/10.1038/nrmicro.2016.94 [Google Scholar] [PubMed] [CrossRef]
34. Mateos, R., Sotres, A., Zaiat, M., Gonzalez, E., Escapa, A. (2018). Microbial electrosynthesis (MES) from CO2 is resilient to fluctuations in renewable energy supply. Energy Conversion and Management, 177, 272–279. https://doi.org/10.1016/j.enconman.2018.09.064 [Google Scholar] [CrossRef]
Cite This Article
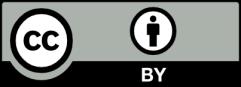
This work is licensed under a Creative Commons Attribution 4.0 International License , which permits unrestricted use, distribution, and reproduction in any medium, provided the original work is properly cited.