Open Access
REVIEW
A Review of Graphene Oxide Crosslinking as Enhanced Corrosion Shield Application
Nanotechnology & Catalysis Research Centre, Institute for Advanced Studies, University of Malaya, Kuala Lumpur, 50603, Malaysia
* Corresponding Authors: Nurul Anis Athirah Ab Aziz. Email: ; Chin Wei Lai. Email:
(This article belongs to the Special Issue: Carbon-Based Nanomaterials from Renewable Materials: Synthesis, Properties and Applications)
Journal of Renewable Materials 2023, 11(6), 2745-2770. https://doi.org/10.32604/jrm.2023.025899
Received 04 August 2022; Accepted 20 September 2022; Issue published 27 April 2023
Abstract
Nowadays, corrosion is not only undesirable, but it also has a significant influence on the industrial sectors and technical innovations that have demand for metals. The global economic damage is expected to reach $2.5 trillion, equivalent to more than 3.4% of the world’s GDP in 2013. It is linked with significant financial harm, manufacturing pollution, and safety issues. An electrochemical process primarily induces metal corrosion at the metalelectrolyte interface region, caused by steel oxidation and the reduction of oxygen, protons, and water. Therefore, organic and epoxy coatings can be applied as protective coatings. Additionally, it can prevent metal corrosion in various fields due to its unique properties, like the ability to manage its curing process over a long period. This review paper focuses on improving the physicomechanical properties of modified graphene oxide (GO) coating by attaching a polymer, epoxy resin (EP). Moreover, we reviewed recent achievements for different methods in minimizing a corrosion phenomenon. Further, we considered the modification of epoxy resin using the curing agent as a bridge to the polymer to strengthen the functionalization of nanocomposites during the reaction. Afterward, we discussed the relationship between the modified GO/EP anticorrosive coating and physicomechanical properties since it is currently being questioned and remains unresolved. As a result, extensive studies on the mechanism of synthesis of the modified GO/EP anticorrosive coating were highlighted.Graphic Abstract

Keywords
Corrosion is not only unfavorable, but it also has a huge impact on the economy of industrial zones, resulting in substantial losses of profits [1–4]. According to reports, due to corrosion, one-third of total metal production declined for industrial purposes [5]. Corrosion can be classified into numerous types, including general corrosion, localized corrosion, pit corrosion, galvanic corrosion, and corrosion chemicals. According to a prior publication citing a study from the National Association of Corrosion Engineers (NACE) International, corrosion damage led to an estimated 2.5 trillion dollars in financial damage globally, accounting for almost 3.4% of the world’s GDP during 2013 [6]. Both safety and effects on the environment are often excluded from such expenses. Generally, the high cost of corrosion is seen in several fields, such as agriculture, industry, and services. Some sectors have discovered that a shortage of corrosion control may be costly due to close encounters, accidents, compulsory closures, and disasters. thus, efficient corrosion control can result in considerable financial savings throughout the lifespan of the property [7]. As shown in Fig. 1, corrosion is a significant cost strain and among the country’s highest costs. Meanwhile, Table 1 lists the approach’s benefits and drawbacks in minimizing the corrosive phenomena. Such phenomena should be dealt with by researchers and technologists worldwide as they are connected to significant damage to the economy, production pollution, and hazard risks. It would be worth mentioning that coatings are commonly employed to shield steel from corrosion, as are other accepted procedures for creating quality protective coatings. It can be noted that metal corrosion is a widespread concern, as it is one of the most severe difficulties that people have encountered, especially ever since metals have been used in everyday life [8].
Figure 1: Corrosion expenses for 3.4% of GDP during 2013
Metal corrosion is typically induced through an electrochemical or chemical process at a metal-electrolyte interface region and leads to steel oxidation and the reduction of oxygen, protons, and water [9–11]. The alignment of cathodic and anodic reactions and the substance interaction occur at specific points. At the cathode, oxygen is reduced chiefly along with an active catalytic layer of oxidized metal. At the same time, many chemical reactions happen at the anode, culminating in the formation of ferrous ions when iron (Fe) atoms disperse through the wet layer and leave a negative charge in the metal, as in Fig. 2 [12]. Hence, as a depolarizer takes electrons from the metal, corrosion occurs. For example, aluminum and its alloys corrode when they begin to interact with corrosion attacks involving chlorine, water, oxygen, and many more. As a result, the essential way to avoid metal corrosion is to minimize direct interaction between metal surfaces and corrosive environments [13].
Figure 2: Graphic figure of the corrosion mechanism
Corrosion has become a leading detrimental problem and a long-term process throughout many industry sectors and technology advances involving the need for metals [14,15]. Presently, deteriorated coatings are generally replaced or cured, which is pricey and time-consuming, which has evolved into products with self-healing characteristics [16–18]. Various solutions have been presented in recent days to address this issue and increase the anticorrosion properties of coatings [19]. Several difficulties have pushed experts toward perfecting numerous tactics for controlling corrosion mechanisms and inventing an appropriate way to overcome these issues. Metal barrier technologies have been classified as (i) corrosion-resistant materials, (ii) corrosion inhibitors, (iii) cathodic protection, (iv) anodic protection, (v) conversion coatings and organic coating protection, (vi) protective polymeric films, and (vii) paint coatings as shown in Table 1 [20]. Therefore, to sum up, an organic coating is employed as one of the most anticorrosive barriers, which can be a protective layer that delays the corrosion of metals throughout a range of areas owing to its fascinating features, including the capability to regulate the corrosion process over a lengthy amount of time [21,22].
Recently, there has been a surge of interest in using graphene as an active layer, possibly as in addition to becoming a good corrosion protection coating additive. Compared to untreated graphene anticorrosive coatings, anticorrosive graphene-composite coatings are not just graphene’s outstanding chemical durability and efficient electrical properties but also its good results owing to its characteristics. Graphene’s overall corrosion protection effectiveness by self-healing, superior mechanical, impermeability, and self-cleaning capabilities [23–26]. Therefore, protective coatings based on graphene-composite can be a new entity as fillers in polymer composites in corrosion protection coating materials for various applications [27,28]. Hence, graphene substitutes like graphene oxide (GO), reduced graphene oxide (rGO), and other materials that are employed and other corrosion protection additives in self-healing coating materials to enhance corrosion resistance qualities, including adherence, durability, and long drying rate [29–31]. The synthesis of GO-polymer-functionalized nanocomposites is a promising technique for improving the coatings’ corrosion protection and physicomechanical performances [32,33]. As a result, GO might well be employed for various purposes and shows potential as an indispensable product in developing new materials [34]. Furthermore, GO can also act as a barrier, which is critical for long-term protection [18]. The low conductivity of GO, in comparison to graphene, reduces charge transport within the coating, enhancing protection against corrosion [35].
Recent studies have indicated that organic coating protection has emerged as the leading candidate method. It has been attracting increasing interest because of the efficacy of its composites and the ease of its synthesis process for keeping metals from corrosion [45]. Coating an organic coating onto a metal surface is a cost-effective technique of shielding the steel surface while retaining the ideal mechanical characteristics of the base material.
The corrosion resistance materials method was reported to have a better lifespan and low environmental toxicity but involves a high-cost operation. A solution was discovered when nickel aluminide, Ni3AI, was micro-alloyed with boron [46]. The use of Ni3AI-based alloys for high-temperature applications is predicated on their generally excellent mechanical, thermal, and physical features.
Next, the use of corrosion inhibitors is a low-cost technique and an expert one. Unfortunately, it may lead to the pollution of the environment. Graphene can create a protective barrier between the metal and a corrosive environment. According to cyclic voltammetry tests, it significantly inhibits metal oxidation and oxygen reductions [47]. Tafel’s research showed that metal with a multi-layered graphene sheet corroded 20 times slower than a raw nickel.
The cathodic protection method is easy to implement and has a low cathodic interruption, but it has inadequate performance. Dong et al. used localized electrochemical impedance spectroscopy (LEIS) to investigate localized steel corrosion at a defect [48]. Metal corrosion was shown to be dependent on cathodic protection potential and flaw shape. The rate-limiting stage in steel corrosion is the bulk of oxygen going through the flaw with a narrow, deep shape.
Through anodic protection, the corrosion behavior can be improved, but the surroundings will be easier to become corrosive due to the dissolution of oxide. Anodized coatings can efficiently reduce the corrosion of magnesium (Mg) materials by covering the substrate contact area and slowing the infiltration of the corrosive solution [49]. Coating thickness affects the number of through-pores, and as a result, anodized Mg alloys have high corrosion resistance.
Conversion coatings can improve efficiency and be a sustainable substitute. Nevertheless, the sensitivity of localization can easily be affected, and the deposition efficiency of the film will be reduced. Following a suitable process, the extracts were analyzed through the phosphate permanganate procedure, and the cathodic epoxy was electro coated [50]. The foremost serious aspect of generating high-quality conversion coating materials was pH stabilization.
Protective polymeric films were reported to be effective due to their practical synergistic effect and good adhesion to metal. However, they were also shown to have poor wettability. According to electrochemical impedance spectroscopy (EIS), for the anticorrosion coating of E32 marine steel developed under curing conditions, the polyurethane acrylic paint had positive results when using the epoxy base coat coating [51]. The paint coating method has excellent coating protection and anticorrosive properties. The resulting adherence is weak due to the increment of color concentration. Truong et al. [52] reported that acid acrylic paint incorporating electrically conductive polypyrrole (PPy) showed significant cathodic open circuit potential scans and EIS for the PPy-coated sample. The large cathodic current densities were attributed to the cathodic reactions with low voltage spikes at the PPy particles.
2 Graphene’s Properties and Characterization
Graphene is a recently discovered source of carbon allotrope that has stimulated research interest and broadened the research field on composite material uses due to its exceptionally developed surface area and mechanical, electro-optical, chemical inertness, and thermal properties [53–57]. Owing to its unique features, including solid thermal properties (3000–5000 W/mK), large surface area (2630 m2/g), high charge carrier mobility (200000 cm2/V), and inherent toughness (130 GPa) as a flat layer [58–60], graphene has piqued the curiosity of many researchers. These magnetic properties inspire substantial efforts to utilize graphene in various applications involving hydrogen generation, detectors, batteries, ultracapacitors, photovoltaic cells, and bioproducts. As a result, several studies and approaches have been explored to achieve a reliable graphene process.
Geim and Novoselov extracted graphene, a minor substance in history with scientific value, a single sheet of carbon atoms, exfoliated from graphite in 2004 by applying the “Scotch tape” technique [61]. Graphene can be defined as a one-atom-thick, uniform single-layer structure made of carbon atoms with a zero-gap two-dimensional (2D) atomic lattice with strong sp² carbon-carbon interaction in a hexagonal structure shape and large aspect ratio [28,62,63].
Graphene is distinguished by various aspects that make it a suitable protective layer. Surfaces covered with graphene, for instance, might keep their visual aspect owing to the excellent transmitted light of graphene (97%) in a broad range of wavelengths [64]. In addition, because graphene is adaptable, graphene coatings may adapt to such stiffness and distortion of the surface layer. The aromatic C=C bond structure in graphene spreads throughout the whole basal plane due to the delocalization of the outer electrons, giving graphene thermal stability. This chemical inertness is required for its application as a protective layer. Once exposed to intense pressure and overheated water, graphene is extremely durable than diamond [65].
To understand more about graphite’s composition, British scientist B. C. Brodie researched the reactivity of flaky graphite in 1859. He concluded that the molecular weight of graphite should be 33 [66]. Graphite was recognized as a natural resource after about 500 years. The use of graphite in the physical science industries began about 150 years after that. Graphene is an incredibly thin layer of carbon atoms with a surface thickness of 0.5–1 nanometer (nm), owing to the chemical imbalance between graphene and its substrate. Raman spectroscopy is among numerous methods that can characterize graphene and is now commonly used. In contact or tapping mode, surface topology, flaws, and bending characteristics can all be investigated using atomic force microscopy (AFM).
Graphene is hypothesized to be composed of pure aromatic ‘islands’ of different diameters that are not oxidized. They may be separated by aliphatic areas, including hydroxyl and epoxide groups, and double bonds [67–70]. Besides, nuclear magnetic resonance (NMR) spectroscopy has been employed for centuries to link spatial features of diverse non-crystalline materials [71]. X-ray diffraction (XRD) is also a method for characterizing several types of graphene. Raman spectroscopy is used to measure the conversion of sp³ polarized carbons, which returns to sp² during GO reduction.
Transmission electron microscopy (TEM) can precisely determine the number of layers of graphene using. Graphene layers are not perfectly flat and experience static disturbances from the plane with a size of 1 nm. The hanging graphene layers represent this in the TEM, which may provide a picture of the graphite structure and the well-defined electron diffraction patterns. In addition, contrary to a few of the preconceived notions about graphene layers’ performance, there is no inclination for them to be spiral or bent., Naebe et al. [72] said that the tensile properties of composite coatings with even a small amount (0.1–1.0 wt.%) of graphene loadings in the epoxy coating could improve the tensile strength, failure strain, and Young’s modulus.
Naebe et al. [72] discovered that the electrically charged graphene/polymer nanocomposite’s resistance constantly decreased as the graphene nanosheet concentration increased. Once the graphene nanosheets reached a specific number, the resistivity dropped precipitously. The resistance was steadily lowered as the loading of graphene nanosheets increased. It can be highlighted here that the number of graphene nanosheets may be less than 0.1 vol percent, implying that graphene/polymer nanocomposite with no corrosion-promotion activity must have a small number of graphene nanosheets. As a result, identifying the peak loading of graphene nanosheets is critical in developing graphene nanosheet-based corrosion protection coatings.
Various techniques can be employed for synthesizing graphenes, such as micromechanical exfoliation, chemical vapor deposition (CVD), graphitization, solvothermal, liquid-phase exfoliation, thermal and electrochemical exfoliation, and chemical reduction processes. Most studies have relied on the fabrication of graphene layers using various techniques. Graphene was initially created using micromechanical exfoliation, which included peeling away a coating of graphite powder with sticky tape while tailoring it to a millimeter size [73]. Nonetheless, the approach possesses yield limitations, rendering it unsuitable for sizable deployment.
Chemical vapor deposition (CVD) is a well-known way of depositing or growing nanostructured graphene, either crystalline or amorphous, from solid, liquid, or gaseous substrates. One method produces large single-crystal graphene and seems to have poor output; this output can be used in electrical devices [74]. Another technique uses porous flakes or layers to create amorphous graphene; despite its small volume, it has high productivity. Graphene has a large specific surface area (SSA) and excellent stability, so it is suitable as electrode material in supercapacitors.
Polymer graphitization is an affordable method for producing nanostructured graphene in various materials with configurable shapes, diameters, and properties. The precursor fabrication yields crystallite sizes of several ranges in nanometers, which are substantially smaller than conventionally generated polycrystalline graphene [75]. This method allows for the flexibility of graphene growing on multiple surfaces with fixed thicknesses and sequentially obtaining various forms. Solvothermal production is a typical humid process that uses liquid or an organic phase as the precursor solution in a closed chamber at temperatures higher than the solvents’ boiling points [76]. The approach is utilized to form extremely thin 2D nanomaterials, particularly for inorganic materials.
Liquid-phase exfoliation is the most flexible procedure for obtaining colloidally stable dispersions from diverse, layered materials. The energy content of the ultrasonic treatment or shearing is utilized to resist the van der Waals attraction within the massive, layered material. There are still challenges in handling graphene, particularly the graphene’s low water solubility and dispersion stability in certain conventional solvents [77]. A thermal exfoliation is an efficient tool for exfoliating graphite flakes. In a broad sense, it aids the incorporation through covalent or noncovalent functionalization, expansion, and swelling, organic molecule adsorption in gaseous form, solid nanoparticle implantation, or direct molecular peeling [78]. Thermal investigations have shown that some functional groups may be eliminated at temperatures lower than 300°C.
The electrochemical approach can scale graphene synthesis, but numerous downsides must be addressed. The process employs a liquid mixture (electrolyte) and an electric current to form a graphite electrode. The graphite-based electrode undergoes anodic oxidation or cathodic reaction during this procedure. Cathodic reactivity techniques are more suited for producing a few high-quality layers of conductive graphene [79].
A reducing agent and, in some instances, an additive is used in the chemical reduction of GO [80]. A substantial reduction in the capacity is required for the elimination of oxygen-containing compounds from GO. However, the procedure must leave zero residues that can harm the output. The creation of graphene-like substances from reducing GO can be expanded. The imperfection of GO and the inevitable formation of defects throughout the oxidation process may be obstacles.
2.1 Graphene Chemical Crosslinking
The most significant graphene derivative is graphene oxide (GO). Because of the presence of multiple polar groups, GO exhibits a high-water dissolution rate. According to Lerf and Klinowisk, the basal planes are populated by hydroxyl oxide and epoxy groups, while carboxyl groups are found on the edges of GO sheets [81]. These characteristics improve the compatibility of GO, where it can be easily dispersed in solvents or polymers.
Chemical functionalization, or crosslinking, is among the most common approaches for manipulating the physicochemical characteristics of different properties and studying the processes involved in engaging other properties with the surroundings [82]. There are two major forms of functionalization: covalent, through covalent bond creation, and noncovalent, through van der Waals forces alone. Such interactions are intended to reduce the polymer matrix’s dispersion features and improve the polymer’s solubility and GO nanostructures [45,83,84].
The covalent functionalization of graphene is chosen for its lasting efficiency and constancy. As a result, this may be the most advantageous method for producing graphene-based composite materials. The polymer matrix’s characteristics are often enhanced to satisfy the demands. Furthermore, graphene-polymer composite structures are adaptable and beneficial for additional functionalization, including integration with nanomaterials [85]. It offers many uses for composite materials, such as corrosion protection coatings.
Hence, covalent functionalization significantly alters the geometrical and electrical features of graphene. Meanwhile, several density functional codes applied today need to consider the inclusion of the consequences of the van der Waals force, which contribute significantly to noncovalent functionalization [86]. The density-functional theory (DFT) is today’s modern gold standard, and the typical local (LDA) and semi-local density approximations (GGA) have an extensive variety of applications [87]. Chemical functionalization in graphene can be associated with ionic covalent bonding.
Seifert et al. [88] discovered that graphene’s level of electrolysis of water had a direct influence on anchoring density. Styrene compounds can be bonded to copper-supported graphene mechanically. As a result, Poly(styrene-co-acrylate) copolymer brushes are created by copolymerizing styrene and acrylates and consecutive crosslinking between component groups. It is possible to achieve a perfect balance between the density of surface groups and the density of defective areas on graphene. It can also be used to construct graphene-based block copolymers for corrosion protection coatings quickly.
Meanwhile, Yu et al. used a monolayer of (3-aminopropyl) triethoxysilane (APTES) to modify GO and then coated it with TiO2 to create TiO2-GO anticorrosion epoxy coatings [89]. Covalently reacting the GO with the silanol moieties of the 3-glycidoxypropyl-trimethoxysilane (GPTMS) monomer, Xue et al. formed silane functionalized GO connected by C-O-Si structures [90]. Xu et al. [91] reported that they crosslinked functionalized GO to pentaerythritol (PER) in water and used it in a flame-retardant polypropylene (PP) solution. The GO and CNTs were bonded with 3-amino phenoxy phthalonitrile, and the resultant composite substance was employed to enhance the efficacy of the anticorrosive coating [92]. Water-stable dispersions of imidazole ionic liquids were effectively covalently bonded to the surface of GO nanosheets for use in polymer composite anticorrosive coatings [93].
The noncovalent functionalization of graphene is accomplished primarily through π-π stacking, hydrophobic links, van der Waals forces, and electrostatic connections [94,95]. This approach is intriguing as it does not disrupt the attached sp² bonds while retaining its excellent thermal and electrical conductivity. The π-π reactions have two parameters, one being the presence of a structure and the next being the presence of a correlation between the two interconnected elements, which is generally encouraged by the polarizability of the two components.
Xu et al. used a water-soluble pyrene compound, 1-pyrenebutyrate (PB), as a preservative to create stable aqueous suspensions of nanocomposite [96]. The pyrene moiety is shown to have a significant affinity for the graphite lattice by stacking [97]. GO was successfully fabricated using pyrene butyric acid without a base, and the resultant product was decreased with hydrazine. Wang et al. noncovalently altered a graphene sheet using pyrene butanoic acid succinimidyl ester (PBSA) to enhance the power efficiency of graphene [98]. The graphene reactions and PBSA do not influence the visible area of the optical absorption of the graphene layer. Compared to pristine graphene (0.21%), this enhanced power conversion efficiency to 1.71% for functionalized graphene.
Graphene oxide, moderately reduced graphene oxide (rGO), and functionalized graphene is used as reinforcing filler to the waterborne polyurethane (PU) substrate to make corrosion protection coatings with stable loadings as significant as 20% [99]. Tamilarasan et al. [100] created electroplating Ni-P-rGO coatings on low-carbon metal by adding various amounts of rGO into the electroplating Ni-P bath. Raghupathy et al. electrochemically formed Cu-GO composite coatings on mild steel substrates by scattering GO in the solution to achieve a homogenous and tight structure [101].
3 Graphene-Based Polymers as an Anticorrosive Coating
Based polymer coatings are now among the highly efficient and emerging trends used to inhibit the development of corrosion products owing to their excellent corrosion protection, significant cost efficiency, and limited usage restrictions [102–105]. Strengthening polymers has increasingly become the focus of study and scalable methods against corrosive media in polymer materials research [106]. Introducing flexible or elastic polymers such as elastomers and rubber has commonly been regarded as an effective method of toughening polymeric materials. Graphene and its derivatives efficiency in reinforcing polymers vary depending on the polymer chain for the polymer composites’ improvement [107–111]. The gains in hardness of various polymeric matrices assessed using that method vary. For instance, the strengthening efficacy of GO on epoxy, nylon, polyurethane, and polyamide, for example, differs greatly even when the GO concentration is the same [112]. As a result, incorporating functional GO into the barrier property of polymer coatings is a potential approach improving their corrosion resistance [31,113–119].
Epoxy resin (EP) has become a very valuable polymer matrix that is utilized in a wide range of applications in industries for building components, cell replacements, anticorrosion coatings, adhesion, semiconductors implementation, and insulation in production [58,89,120,121]. Owing to its good chemical resistance, outstanding barrier qualities, minimal cure shrinking, and superior insulation, epoxy resin has been developed as a long-lasting anticorrosive organic coating material for several uses [20,122,123]. However, numerous solutions, such as nanofillers, have enhanced surface coatings’ barrier and corrosive resistance of surface coatings [124]. Naebe et al. [72] revealed that introducing only 0.1 wt.% of functionalized graphene platelets into an epoxy resin, increased the accelerated condition and storage elasticity to 22% and 18%, respectively. Even though epoxy resin offers high chemical resistance, excellent adherence to metallic substrates, great electricity generation, and other properties, it can be fragile as it has brittle nature and poor heat resistance [121,125–127].
Due to the rich hydrophilic chemical groups, the metal beneath it suffers a reduction in protection when epoxy attracts water from the environment to which it is exposed [128]. Several attempts have been made to enhance the epoxies’ protectiveness using different nanofillers to address this issue [125]. As a result, the epoxy resin becomes a common solvent-borne dispersion medium that includes a few volatile organic compounds (VOCs) because it contains a specific amount of volatile organic chemicals. An epoxy matrix is considered undesirable for the environment and health [129].
Unbleached epoxies are distinguished primarily by their epoxy concentration, color density, hydrolyzable chlorine, and volatility substance. Epoxide equivalent weight or weight per epoxy (WPE) is commonly used to express the epoxy content of aqueous polymers. WPE is characterized by the resin accumulating 1 g of epoxide equivalent weight. The standard molecular weight of commercial-grade liquid epoxy resins is around 370. At 25°C, the viscosity is 11–15 Pa⋅s, and the weight per epoxide (wpe) is approximately 188. FT-IR and NMR spectroscopy approaches can identify hydroxyl reactivity. Thermogravimetric analysis (TGA) and differential thermal analysis (DTA) are employed for measuring heat transfer characteristics. DSC and DMTA analyses can investigate curing behavior and glass transition.
3.1.1 Curing Agent for Epoxy Resin
Curing agents and hardeners are relatively similar in that they are required to promote or regulate the hardening of the polymers. A hardener added to the epoxy polymer allows it to be bonded. Major transitions occur inside the epoxy resin throughout the hardening process. The composition of curing chemicals determines the speed and temperature of the epoxies’ cure process. Due to their chemical composition, strengthening agents are classified as amino groups, alkaline, anhydrides, or catalyst-curing agents.
Some curing agents enhance curing while others directly participate in the reaction while remaining bonded covalently to the resin, such as in Table 2. The coated material can be a homo epoxy polymer, a heteropolymer composed of epoxy resin and curing agent, or a combination of the two. Promising epoxy curing agents with appropriate adaptive group functions and extra reinforcing functions in epoxy composites have piqued the curiosity of many researchers. Thus, a curing procedure with relevant functionalities can result in higher bond strength. The adhesive strength of epoxy resins is determined based on the curing environment and temperature, where low temperatures lead to weak strength, while high temperatures increase the strength.
Along with its extended lattice structure, epoxy is sensitive to various curing agents, including amines, anhydrides, carboxylic acids, alcohols, and thiols [130]. Since sensitivity seems unlikely to be an issue, the choice of hardener has mainly been influenced by the predicted form and characteristics. Co-reactive curing agents generally produce polymer chains, whereas catalyzed curing agents produce chain-growth polymers.
3.1.2 Epoxy Resin-Based Composite Materials
Strongly crosslinked epoxy resins are often rigid and robust while having low resistance to crack formation, which limits their utilization across numerous applications. Numerous methods have been utilized for enhancing physical qualities, including toughening or reinforcing chemicals, as shown in Table 3.
As for the elastomer-modified epoxy, it typically results in many forfeitures in modulus and strength to attain high toughness. Elastomers in epoxy have long been considered a viable alternative to rubber toughening to resolve epoxy’s brittleness. Elastomer components, including poly (ether sulfone) (PES), poly (phenylene oxide) (PPO), poly (ether imide) (PEI), poly(acrylonitrile-co-butadiene-co-styrene) (ABS), polysulfones (PSF), poly (ether ketone) (PEK), and polyimides (PI), are added into the epoxy to form elastomer modified epoxy [136,137].
Hence, the functionalization of epoxy/inorganic nanocomposites can increase the impact strength, where certain inorganic particles are also used for epoxy resins. Using of inorganic materials offers resistance to deformation and fracture initiation via hardening processes. Inorganic nanoparticles added to epoxy resins can enhance elasticity, stiffness, and impact resistance. Carbon fibers with excellent mechanical properties can be absorbed into a range of polymer matrices. If a mass is added to a carbon fiber composite, the stress is mainly transmitted via the polymer composite from one carbon fiber toward another carbon fiber. If somehow, the binding between the epoxy and the fibers is poor, this mass transmission will diminish or break down the interface. Sized, a polymer mixture that enhances carbon composite adherence to the polymer resin, is typically used for fibers [138–140].
The synthesis of polymer/clay composite materials may lead to clay aggregation, leading to high structural and hardness degradation. Different composites are formed when surface-treated ceramics are incorporated into substrate polymer matrices, intercalated or disintegrated composite structures [141,142]. Compared to pure epoxy matrices and conventional, tiny hybrids, epoxy/clay nanoparticles with minimal clay content demonstrate significantly better tensile properties, thermal properties, susceptibility, and heat resistance. The improved method for epoxy/clay composites results from the nanocomposites’ robust interface/volume relation, which is related to the nanoscale-level clay particle dimensions [143,144].
Numerous researchers focus on carbon nanotubes (CNTs) due to their sizeable interfacial region and intrinsic properties for formulating a more efficient polymeric matrix with excellent mechanical properties, better stiffening efficiency, and greater thermal conductivity [145]. There are mainly three types of CNTs, which are single-walled (SW), double-walled (DW), and multiple-walled (MW) CNTs.
3.1.3 Application Fields of Epoxy
Paints and Coatings
Due to their various advantages, including ease of handling, good safety, excellent solvent, and chemical stability, high hardness, good dimensional stability on healing, good structural and strength properties, and excellent adhesion to several substrates, epoxy materials are commonly used as corrosion protection coatings for heavily loaded items. Steel cartons and bottles are routinely coated using epoxies to prevent corrosion, especially while preparing low-pH foods such as tomatoes. Epoxy resins are used in textured and somewhat raised flooring applications.
Adhesive
The adhesive is a vital characteristic of the gluing form known as “adhesive tapes”. These improved adhesives are exploited in manufacturing airplanes, vehicles, bicycles, boats, golf clubs, skis, skateboards, and other interfacial uses. For it to be utilized as a sealant in cryogenic engineering fields, the epoxy tensile toughness must be optimized in both cryogenic and ambient environments. The toughness of manufactured epoxy additives is engineered by inserting sequence thermoplastics, rubber particles, or stiff inorganic materials through the substrate. Silicone additives have often been treated at high temperatures to improve their strength and initiate complexation at the surface contact.
Industrial Tooling
Throughout advanced manufacturing equipment operations, epoxy systems create molds, master approaches, fiberboard, castings, fittings, and several essential manufacturing tools. Such “plastic tooling” can become substitutes for metal, wood, and some conventional items and enhance operational efficiencies by decreasing expenses or cutting timeframes for numerous production lines. Fiber-reinforced epoxy polymers are helpful in the restoration of metal materials and cylindrical pipelines.
Aerospace Industry
Owing to their outstanding adhesion characteristics and minimal cost, epoxy resins are already widely employed for systemic adhesive purposes in the aircraft sector. The aircraft industry is most interested in epoxy resins that are strengthened with extra-strong silica, carbon, Kevlar, or tungsten fibers.
Electronic Material
The semiconductor industry uses epoxy resin compositions in engines, turbines, transformers, switchgear, bearings, and capacitors. Epoxy resins are good electrical conductors that shield circuit boards from short circuits, dust, and moisture. Electromagnetic interference protection is a common need for steel composites. Epoxy molding compounds (EMCs) are widely utilized as encapsulating materials for electronic components to protect them from humidity, portable pollutants, and adverse climatic factors such as temperature, ultraviolet light, humidity, and physicomechanical harm. In electronic packaging applications, epoxy composites incorporating particle additives such as quartz glass, marble powder, and mineral silica are also employed as matrix materials.
Biomedical Systems
In the clinical field, epoxy resins are commonly employed. For human therapeutic uses, collagen-based substances are utilized as bandages for wounds, bone grafts, and aortic heart valves. Elastic polymer foams, such as embolic sponges, have great potential as biomaterials. Due to their extreme toughness, remarkable chemical inertness, poor electrical and solid thermal resistance, broad spectral opacity, and several specific characteristics, nanodiamond epoxy substitutes have been significantly used in biomedical fields.
4 Graphene Oxide/Epoxy Nanocomposites
One of the thorniest issues in the fabrication of GO/epoxy composite materials is achieving a proper dispersion of the nanostructure. A well-dispersed phase implies that the largest surface area of the filler is accessible, which also influences the surrounding crosslinks and, as a result, the characteristics of the nanocomposite. The fabrication methods used for epoxy or any matrix considerably impact dispersion. Extensive studies have been undertaken regarding fabrication approaches for generating a homogenous and evenly distributed system. Solvent blending and epoxy bonding are the most commonly utilized GO/epoxy composite material approaches.
One of the primary and typically preferred approaches for synthesizing GO/epoxy nanocomposites involves capitalizing on the availability of hydroxyl groups linked to the graphene sheets, allowing for the spontaneous dispersion of graphene in various chemical solvents. This approach also correlates the nanostructured graphene’s extensive organic or inorganic reactivity with different polymers. Usually, synthesized graphene is dissolved using a specific solvent, such as via ultrasonic treatment, after which it is coated into the epoxy matrix, where the solution is evaporated under specified parameters. A guiding idea will be to use an organic solvent for the surface hydroxyl groups of graphene and a chemical bonding that is ideal for the polymer matrix. Numerous solvents are being explored to improve the dispersion of nanocomposites, as in Table 4.
4.2 Water-Based Epoxy (WEP) Resin Coating
Water-based epoxy (WEP) coating has recently piqued scientists’ interest as it satisfies pollution control requirements [156]. Furthermore, it offers several benefits over solvent-borne epoxy coating, including good adaptability and firm adherence to a wide range of steel substrates [156]. Researchers have discovered that nanofillers can be employed to increase corrosion resistance and fix flaws. As a result, fillers have demonstrated the highest permeation since they have a capable shield for nanopores [89].
To remedy these shortcomings, GO has attracted attention as it can be exploited as an ideal additive for WEP coatings, mainly because of its polar functional groups, such as hydroxyl, carbonyl, carboxyl, and epoxy [157]. However, the performance of GO as a filler in corrosion inhibitors is still being debated [158]. Furthermore, owing to the intrinsic properties of water-based coatings, deformation, fractures, and micro-cracks are probable to occur inside WEP following layer formation [159,160]. In theory, the surface modification or functionalization of GO not only enhances the adhesion of the WEP coating method but also considerably improves the epoxy coating’s mechanical, electrical, thermal, and barrier qualities.
5 Graphene Oxide/Nanofiller Composites Materials
Recently, adding nanofiller has been an excellent technique to promote corrosion resistance [161]. Ongoing efforts are made to enhance the physicomechanical and anticorrosive characteristics of protective coatings by using an optimal number of crosslinking agents and additives, as shown in Table 5. Ramezanzadeh et al. [162] stated that moist transition was used to introduce polyisocyanate nanostructured GO nanosheets through the polyurethane substrate. Hence, the use of 0.1 wt.% GO, and PI-GO significantly improves the coating’s anticorrosive capabilities and electrical resistance. The study on this issue is in its initial phases and is rapidly developing.
Zheng et al. [163] demonstrated that GO sheets significantly increased the protection against corrosion of epoxy coatings over carbon steel substrates. The excellent dispersal of modified GO films in the polymer matrix was maintained, resulting in the improved reactivity of a prepolymer of urea–formaldehyde (UF) resin with the polymer matrix. It can be concluded that TiO2-GO hybrids can be proactively applied as nanofillers for anticorrosive epoxy coats, according to Yu et al. [89].
Both graphene oxide layers and polymer materials have lower utilization fillers for WEP coatings as they have low water dissolving rates and linking, which may result in free spaces and reduced coating barrier efficiency [158]. Nevertheless, graphene with strong electron conductivity has a better capacity than others and offers long-term barrier properties in WEP coatings [129].
Because of its weak water dissolution rate and crosslinking, both graphene oxide layers and organic polymers possess ineffective compliance fillers for WEP coating, which may generate free spaces and subsequently degrade the barrier efficacy of the coatings [158]. However, graphene with strong electron conductivity possesses a better ability than others and can have long-term corrosive resistance in WEP coatings [129].
Several countries are currently employing green chemistry frequently to drastically reduce the overall usage of harmful substances and thereby repair the ecosystem to encourage ecologically friendly corrosive corrective actions [167]. Functional biofilms have recently grown in popularity as an environmentally safe corrosion protection technique [168]. It is critical to highlight those specific stabilizers, toxic-free reagents, and sustainable reducing agents have become the leading and crucial components in the production and stabilization of nanoparticles. Chen et al. [169] have reportedly devised an eco-friendly manufacturing method epoxidized biomass Eucommia gum (EEUG), as a nanocomposite in epoxy polymer composites by utilizing water as a surfactant. The authors discovered that high functionalization densities with 1 wt.% EEUG led to a significant improvement in corrosion prevention efficacy.
We can infer from our review research on the evolution of graphene that its transport characteristics have been effectively established. However, there is still space for improvement regarding its mechanical qualities. Relevant suggestions that bridged the gap between theoretical behavior and the actual achievement of mechanical behavior has already received much interest. These will be summed up as follows.
Due to the scale involved, the most widely investigated methods to produce graphene are through GO. Although all processes begin with the same source, the structural and surface properties of the GO can vary dramatically depending on how it is exfoliated and decreased. Graphite oxidation and heat or chemical reduction cause severe distortion of graphitic carbons, altering their micromechanical and electrical transport characteristics. Nonetheless, the processes of defect development and their impact on nanocomposite characteristics are still unexplored. To optimize the capability and gain of polymeric materials, synthetic methods to produce graphene sheets that prevent structural distortion or excessive functionalization, as well as post-processes that can recover graphitic planar domains, must be pursued.
The adhesion of graphene to polymers is another significant issue to address when creating composite materials. Carbon nanofillers’ improved dynamics can be wholly exploited when tightly bound to matrix polymers. Enhanced adherence may reduce phonon contact dispersion and lead to better composite thermal conductivity and a reduction in surface-free volume, which impacts gas penetration. Thermal conductivity over the graphene/hydrocarbon junction can be improved by chemically adding alkyl groups to the graphene margins.
Graphene/polymer nanocomposites can satisfy thermal or energy storage requirements, static charge disposal, and electromagnetic pulse reflecting medium with electrical solid or thermal conductivity. Composite resistance can differ wildly with temperature, chemical assault, and external pressure, specifically around the threshold. This externally driven on-off phenomenon in electrical characteristics is used to identify stress and electric shifting. If microscopic sheets of highly charged graphene are put on glass or polymer materials, elastic screens, thin-film transistors, and photovoltaic, and crystalline systems will become viable.
Even though graphene has anticorrosion characteristics, imperfections in graphene can accelerate and intensify corrosion. Adding additional components to graphene or its compounds, including graphene oxide, improves its corrosion resistance over time. As a result of its flexibility, such approaches can be commonly applied by researchers in academic and industrial fields to create graphene-based polymer composite coatings. However, combining corrosion inhibitors covalently with polymer-functionalized graphene flakes or metal oxide nanoparticles can aid in increasing corrosion attacks. These composite materials are predicted to exhibit synergistic effects, improving corrosion protection characteristics. Once reliable sources of chemical bonding are employed for chemical functionalization, additional properties such as self-healing qualities, can be exploited to address any downsides. Using polydopamine, polyaniline, polypyrrole, and metallic nanoparticles such as TiO2, Al2O3, or SiO2 are examples of such applications. Hence, these composite materials’ synergistic chemical resistance can lead to effective barriers that inhibit the penetration of corrosion attacks from going further into the coating. As a result, exceptional corrosion protection qualities, long-term rigidity, and toughness are guaranteed.
Funding Statement: This research work was financially supported by Fundamental Research Grant Scheme FRGS/1/2020/TK0/UM/02/8 (No. FP023-2020).
Conflicts of Interest: The authors declare that they have no conflicts of interest to report regarding the present study.
References
1. Sun, D., Yan, J., Ma, X., Lan, M., Wang, J. et al. (2021). Tribological investigation of self-healing composites containing metal/Polymer microcapsules. ES Materials and Manufacturing, 14, 59–72. https://doi.org/10.30919/esmm5f469 [Google Scholar] [CrossRef]
2. Jalgham, R. T. T. (2021). Theoretical, monte carlo simulations and QSAR studies on some triazole derivatives as corrosion inhibitors for mild steel in 1 M HCl. ES Energy & Environment, 13, 37–49. https://doi.org/10.30919/esee8c476 [Google Scholar] [CrossRef]
3. Dey, S., Chatterjee, S., Singh, B. P., Bhattacharjee, S., Rout, T. K. et al. (2018). Development of superhydrophobic corrosion resistance coating on mild steel by electrophoretic deposition. Surface and Coatings Technology, 341, 24–30. https://doi.org/10.1016/j.surfcoat.2018.01.005 [Google Scholar] [CrossRef]
4. Liu, J., Zhang, J., Tang, J., Pu, L., Xue, L. et al. (2020). Polydimethylsiloxane resin nanocomposite coating with alternating multilayer structure for corrosion protection performance. ES Materials and Manufacturing, 10, 29–38. https://doi.org/10.30919/esmm5f912 [Google Scholar] [CrossRef]
5. Othman, N. H., Che Ismail, M., Mustapha, M., Sallih, N., Kee, K. E. et al. (2019). Graphene-based polymer nanocomposites as barrier coatings for corrosion protection. Progress in Organic Coatings, 135, 82–99. https://doi.org/10.1016/j.porgcoat.2019.05.030 [Google Scholar] [CrossRef]
6. Verma, C., Ebenso, E. E., Bahadur, I., Quraishi, M. A. (2018). An overview on plant extracts as environmental sustainable and green corrosion inhibitors for metals and alloys in aggressive corrosive media. Journal of Molecular Liquids, 266, 577–590. https://doi.org/10.1016/j.molliq.2018.06.110 [Google Scholar] [CrossRef]
7. Koch, G. (2017). Cost of corrosion. DNV GL USA, Dublin, OH, USA: Elsevier Ltd. https://doi.org/10.1016/B978-0-08-101105-8.00001-2 [Google Scholar] [CrossRef]
8. Li, X. M., Reinhoudt, D., Crego-Calama, M. (2007). What do we need for a superhydrophobic surface? A review on the recent progress in the preparation of superhydrophobic surfaces. Chemical Society Reviews, 36(8), 1350–1368. https://doi.org/10.1039/b602486f [Google Scholar] [PubMed] [CrossRef]
9. Wei, H., Ding, D., Wei, S., Guo, Z. (2013). Anticorrosive conductive polyurethane multiwalled carbon nanotube nanocomposites. Journal of Materials Chemistry A, 1(36), 10805–10813. https://doi.org/10.1039/c3ta11966a [Google Scholar] [CrossRef]
10. Luo, X., Zhong, J., Zhou, Q., Du, S., Yuan, S. et al. (2018). Cationic reduced graphene oxide as self-aligned nanofiller in the epoxy nanocomposite coating with excellent anticorrosive performance and its high antibacterial activity. ACS Applied Materials & Interfaces, 10(21), 18400–18415. https://doi.org/10.1021/acsami.8b01982 [Google Scholar] [PubMed] [CrossRef]
11. Zhu, X., Ni, Z., Dong, L., Yu, Z., Cheng, L. et al. (2019). In-situ modulation of interactions between polyaniline and graphene oxide films to develop waterborne epoxy anticorrosion coatings. Progress in Organic Coatings, 133, 106–116. https://doi.org/10.1016/j.porgcoat.2019.04.016 [Google Scholar] [CrossRef]
12. Sørensen, P. A., Kiil, S., Dam-Johansen, K., Weinell, C. E. (2009). Anticorrosive coatings: A review. Journal of Coatings Technology and Research, 6(2), 135–176. https://doi.org/10.1007/s11998-008-9144-2 [Google Scholar] [CrossRef]
13. Ma, Y., Ye, Y., Wan, H., Chen, L., Zhou, H. et al. (2020). Chemical modification of graphene oxide to reinforce the corrosion protection performance of UV-curable polyurethane acrylate coating. Progress in Organic Coatings, 141. https://doi.org/10.1016/j.porgcoat.2020.105547 [Google Scholar] [CrossRef]
14. Ikechukwu, E. E., Pauline, E. O. (2015). Environmental impacts of corrosion on the physical properties of copper and aluminium: A case study of the surrounding water bodies in port harcourt. Open Journal of Social Sciences, 3(2), 143–150. https://doi.org/10.4236/jss.2015.32019 [Google Scholar] [CrossRef]
15. Hosseinpour, A., Rezaei Abadchi, M., Mirzaee, M., Ahmadi Tabar, F., Ramezanzadeh, B. (2021). Recent advances and future perspectives for carbon nanostructures reinforced organic coating for anticorrosion application. Surfaces and Interfaces, 23. https://doi.org/10.1016/j.surfin.2021.100994 [Google Scholar] [CrossRef]
16. An, S., Lee, M. W., Yarin, A. L., Yoon, S. S. (2018). A review on corrosion-protective extrinsic self-healing: Comparison of microcapsule-based systems and those based on core-shell vascular networks. Chemical Engineering Journal, 344, 206–220. https://doi.org/10.1016/j.cej.2018.03.040 [Google Scholar] [CrossRef]
17. Zhang, F., Ju, P., Pan, M., Zhang, D., Huang, Y. et al. (2018). Self-healing mechanisms in smart protective coatings: A review. Corrosion Science, 144, 74–88. https://doi.org/10.1016/j.corsci.2018.08.005 [Google Scholar] [CrossRef]
18. Habibiyan, A., Ramezanzadeh, B., Mahdavian, M., Bahlakeh, G., Kasaeian, M. (2020). Rational assembly of mussel-inspired polydopamine (PDA)-Zn (II) complex nanospheres on graphene oxide framework tailored for robust self-healing anticorrosion coatings application. Chemical Engineering Journal, 391, 123630. https://doi.org/10.1016/j.cej.2019.123630 [Google Scholar] [CrossRef]
19. Ding, J., Zhao, H., Xu, B., Zhao, X., Su, S. et al. (2019). Superanticorrosive graphene nanosheets through π deposition of boron nitride nanodots. ACS Sustainable Chemistry & Engineering, 7(12), 10900–10911. https://doi.org/10.1021/acssuschemeng.9b01796 [Google Scholar] [CrossRef]
20. Zhang, C., Dai, X., Wang, Y., Sun, G., Li, P. et al. (2019). Preparation and corrosion resistance of ETEO modified graphene oxide/epoxy resin coating. Coatings, 9(1), 46. https://doi.org/10.3390/coatings9010046 [Google Scholar] [CrossRef]
21. Monetta, T., Acquesta, A., Carangelo, A., Naddeo, C., Guadagno, L. (2019). Enhancement of photooxidative and corrosion resistance of epoxy/graphene water-based coatings on metallic substrate. Progress in Organic Coatings, 135, 7–18. https://doi.org/10.1016/j.porgcoat.2019.05.031 [Google Scholar] [CrossRef]
22. Sari, M. G., Ramezanzadeh, B. (2020). Epoxy composite coating corrosion protection properties reinforcement through the addition of hydroxyl-terminated hyperbranched polyamide non-covalently assembled graphene oxide platforms. Construction and Building Materials, 234, 117421. https://doi.org/10.1016/j.conbuildmat.2019.117421 [Google Scholar] [CrossRef]
23. Ma, M., Hill, R. M. (2006). Superhydrophobic surfaces. Current Opinion in Colloid and Interface Science, 11(4), 193–202. https://doi.org/10.1016/j.cocis.2006.06.002 [Google Scholar] [CrossRef]
24. García, S., Fishcer, H. R., White, P. A., Mardel, J., González-García, Y. et al. (2011). Self-healing anticorrosive organic coating based on an encapsulated water reactive silyl ester: Synthesis and proof of concept. Progress in Organic Coatings, 70(2–3), 142–149. https://doi.org/10.1016/j.porgcoat.2010.11.021 [Google Scholar] [CrossRef]
25. Cui, C., Lim, A. T. O., Huang, J. (2017). A cautionary note on graphene anticorrosion coatings. Nature Nanotechnology, 12(9), 834–835. https://doi.org/10.1038/nnano.2017.187 [Google Scholar] [PubMed] [CrossRef]
26. Huang, H., Sheng, X., Tian, Y., Zhang, L., Chen, Y. et al. (2020). Two-dimensional nanomaterials for anticorrosive polymeric coatings: A review. Industrial and Engineering Chemistry Research, 59(35), 15424–15446. https://doi.org/10.1021/acs.iecr.0c02876 [Google Scholar] [CrossRef]
27. Gergely, A. (2018). A review on corrosion protection with single-layer, multilayer, and composites of graphene. Corrosion Reviews, 36(2), 155–225. https://doi.org/10.1515/corrrev-2017-0016 [Google Scholar] [CrossRef]
28. Ding, R., Li, W., Wang, X., Gui, T., Han, P. et al. (2018). A brief review of corrosion protective films and coatings based on graphene and graphene oxide. Journal of Alloys and Compounds, 764, 1039–1055. https://doi.org/10.1016/j.jallcom.2018.06.133 [Google Scholar] [CrossRef]
29. Kasaeian, M., Ghasemi, E., Ramezanzadeh, B., Mahdavian, M., Bahlakeh, G. (2018). Construction of a highly effective self-repair corrosion-resistant epoxy composite through impregnation of 1H-benzimidazole corrosion inhibitor modified graphene oxide nanosheets (GO-BIM). Corrosion Science, 145, 119–134. https://doi.org/10.1016/j.corsci.2018.09.023 [Google Scholar] [CrossRef]
30. Zhang, L., Wu, H., Zheng, Z., He, H., Wei, M. et al. (2019). Fabrication of graphene oxide/multi-walled carbon nanotube/urushiol formaldehyde polymer composite coatings and evaluation of their physico-mechanical properties and corrosion resistance. Progress in Organic Coatings, 127, 131–139. https://doi.org/10.1016/j.porgcoat.2018.10.026 [Google Scholar] [CrossRef]
31. Zhang, F., Liu, W., Liang, L., Wang, S., Shi, S. et al. (2020). The effect of functional graphene oxide nanoparticles on corrosion resistance of waterborne polyurethane. Colloids and Surfaces A: Physicochemical and Engineering Aspects, 591. https://doi.org/10.1016/j.colsurfa.2020.124565 [Google Scholar] [CrossRef]
32. Zhan, Y., Wan, X., He, S., Yang, Q., He, Y. (2018). Design of durable and efficient poly (arylene ether nitrile)/bioinspired polydopamine coated graphene oxide nanofibrous composite membrane for anionic dyes separation. Chemical Engineering Journal, 333, 132–145. https://doi.org/10.1016/j.cej.2017.09.147 [Google Scholar] [CrossRef]
33. Zhan, Y., Zhang, J., Wan, X., Long, Z., He, S. et al. (2018). Epoxy composites coating with Fe3O4 decorated graphene oxide: Modified bio-inspired surface chemistry, synergistic effect and improved anticorrosion performance. Applied Surface Science, 436, 756–767. https://doi.org/10.1016/j.apsusc.2017.12.095 [Google Scholar] [CrossRef]
34. Smith, A. T., LaChance, A. M., Zeng, S., Liu, B., Sun, L. (2019). Synthesis, properties, and applications of graphene oxide/reduced graphene oxide and their nanocomposites. Nano Materials Science, 1(1), 31–47. https://doi.org/10.1016/j.nanoms.2019.02.004 [Google Scholar] [CrossRef]
35. Zhu, G., Cui, X., Zhang, Y., Chen, S., Dong, M. et al. (2019). Poly (vinyl butyral)/Graphene oxide/poly (methylhydrosiloxane) nanocomposite coating for improved aluminum alloy anticorrosion. Polymer, 172, 415–422. https://doi.org/10.1016/j.polymer.2019.03.056 [Google Scholar] [CrossRef]
36. Huang, J., Lou, C., Xu, D., Lu, X., Xin, Z. et al. (2019). Cardanol-based polybenzoxazine superhydrophobic coating with improved corrosion resistance on mild steel. Progress in Organic Coatings, 136. https://doi.org/10.1016/j.porgcoat.2019.06.037 [Google Scholar] [CrossRef]
37. Alibakhshi, E., Ramezanzadeh, M., Bahlakeh, G., Ramezanzadeh, B., Mahdavian, M. et al. (2018). Glycyrrhiza glabra leaves extract as a green corrosion inhibitor for mild steel in 1 M hydrochloric acid solution: Experimental, molecular dynamics, Monte Carlo and quantum mechanics study. Journal of Molecular Liquids, 255, 185–198. https://doi.org/10.1016/j.molliq.2018.01.144 [Google Scholar] [CrossRef]
38. Xie, F., Li, J., Zou, T., Wang, D., Wu, M. et al. (2021). Stress corrosion cracking behavior induced by sulfate-reducing bacteria and cathodic protection on X80 pipeline steel. Construction and Building Materials, 308. https://doi.org/10.1016/j.conbuildmat.2021.125093 [Google Scholar] [CrossRef]
39. Fernández, A. G., Cabeza, L. F. (2020). Anodic protection assessment using alumina-forming alloys in chloride molten salt for CSP plants. Coatings, 10(2). https://doi.org/10.3390/coatings10020138 [Google Scholar] [CrossRef]
40. Mitton, D. B., Carangelo, A., Acquesta, A., Monetta, T., Curioni, M. et al. (2017). Selected Cr(VI) replacement options for aluminum alloys: A literature survey. Corrosion Reviews, 35(6), 138. https://doi.org/10.1515/corrrev-2016-0059 [Google Scholar] [CrossRef]
41. Carreira, A. F., Pereira, A., Vaz, E., Cabral, A., Ghidini, T. et al. (2017). Alternative corrosion protection pretreatments for aluminum alloys. Journal of Coatings Technology and Research, 14(4), 879–892. https://doi.org/10.1007/s11998-017-9922-9 [Google Scholar] [CrossRef]
42. Pandis, P. K., Papaioannou, S., Siaperas, V., Terzopoulos, A., Stathopoulos, V. N. (2020). Evaluation of Zn- and Fe-rich organic coatings for corrosion protection and condensation performance on waste heat recovery surfaces. International Journal of Thermofluids, 3–4, 100025. https://doi.org/10.1016/j.ijft.2020.100025 [Google Scholar] [CrossRef]
43. Monetta, T., Acquesta, A., Carangelo, A., Donato, N., Bellucci, F. (2017). Durability of AZ31 magnesium biodegradable alloys polydopamine aided: Part 1. Journal of Magnesium and Alloys, 5(4), 412–422. https://doi.org/10.1016/j.jma.2017.09.006 [Google Scholar] [CrossRef]
44. Zheng, D., Gui, Q., Xu, Y., Song, G. L. (2021). Modified AC-DC-AC method for evaluation of corrosion damage of acrylic varnish paint coating/q215 steel system. Progress in Organic Coatings, 159. https://doi.org/10.1016/j.porgcoat.2021.106401 [Google Scholar] [CrossRef]
45. Kasaeian, M., Ghasemi, E., Ramezanzadeh, B., Mahdavian, M., Bahlakeh, G. (2018). Construction of a highly effective self-repair corrosion-resistant epoxy composite through impregnation of 1H-benzimidazole corrosion inhibitor modified graphene oxide nanosheets (GO-BIM). Corrosion Science, 145, 119–134. https://doi.org/10.1016/j.corsci.2018.09.023 [Google Scholar] [CrossRef]
46. Deevi, S. C., Sikka, V. K. (1996). Nickel and iron aluminides: An overview on properties, processing, and applications. Intermetallics, 4(5), 357–375. https://doi.org/10.1016/0966-9795(95)00056-9 [Google Scholar] [CrossRef]
47. Prasai, D., Tuberquia, J. C., Harl, R. R., Jennings, G. K., Bolotin, K. I. (2012). Graphene: Corrosion-inhibiting coating. ACS Nano, 6(2), 1102–1108. https://doi.org/10.1021/nn203507y [Google Scholar] [PubMed] [CrossRef]
48. Dong, C. F., Fu, A. Q., Li, X. G., Cheng, Y. F. (2008). Localized EIS characterization of corrosion of steel at coating defect under cathodic protection. Electrochimica Acta, 54(2), 628–633. https://doi.org/10.1016/j.electacta.2008.07.016 [Google Scholar] [CrossRef]
49. Song, G. L., Shi, L. (2014). Corrosion mechanism and evaluation of anodized magnesium alloys. Corrosion Science, 85, 126–140. https://doi.org/10.1016/j.corsci.2014.04.008 [Google Scholar] [CrossRef]
50. Gray, J. E., Luan, B. (2002). Protective coatings on magnesium and its alloys—A critical review. Journal of Alloys and Compounds, 336(1), 88–113. https://doi.org/10.1016/S0925-8388(01)01899-0 [Google Scholar] [CrossRef]
51. Benea, L., Simionescu, N., Mardare, L. (2020). The effect of polymeric protective layers and the immersion time on the corrosion behavior of naval steel in natural seawater. Journal of Materials Research and Technology, 9(6), 13174–13184. https://doi.org/10.1016/j.jmrt.2020.09.059 [Google Scholar] [CrossRef]
52. Truong, V., Lai, P., Moore, B., Muscat, R., Russo, M. (2000). Corrosion protection of magnesium by electroactive polypyrrolerpaint coatings. Synthetic Metal, 110(1), 7–15. https://doi.org/10.1016/S0379-6779(99)00174-5 [Google Scholar] [CrossRef]
53. Yu, S., Zhao, L., Liu, R., Zhang, C., Zheng, H. et al. (2018). Performance enhancement of Cu-based AZO multilayer thin films via graphene fence engineering for organic solar cells. Solar Energy Materials and Solar Cells, 183, 66–72. https://doi.org/10.1016/j.solmat.2018.04.008 [Google Scholar] [CrossRef]
54. Ma, X., Liu, Y., Liu, H., Zhang, L., Xu, B. et al. (2018). Fabrication of novel slurry containing graphene oxide-modified microencapsulated phase change material for direct absorption solar collector. Solar Energy Materials and Solar Cells, 188, 73–80. https://doi.org/10.1016/j.solmat.2018.08.021 [Google Scholar] [CrossRef]
55. Sarkar, A., Chakraborty, A. K., Bera, S. (2018). NiS/rGO nanohybrid: An excellent counter electrode for dye sensitized solar cell. Solar Energy Materials and Solar Cells, 182, 314–320. https://doi.org/10.1016/j.solmat.2018.03.026 [Google Scholar] [CrossRef]
56. Zhang, C., Fan, S., Shao, H., Hu, X., Zhu, B. et al. (2019). Graphene trapped silk scaffolds integrate high conductivity and stability. Carbon, 148, 16–27. https://doi.org/10.1016/j.carbon.2019.03.042 [Google Scholar] [CrossRef]
57. Cheng, Y., Bi, H., Che, X., Li, D., Ji, W. (2019). Suppression of graphene nucleation by plasma treatment of Cu foil for the rapid growth of large-size single-crystal graphene. Carbon, 147, 51–57. https://doi.org/10.1016/j.carbon.2019.02.025 [Google Scholar] [CrossRef]
58. Zhang, X., Alloul, O., He, Q., Zhu, J., Verde, M. et al. (2013). Strengthened magnetic epoxy nanocomposites with protruding nanoparticles on the graphene nanosheets. Polymer, 54(14), 3594–3604. https://doi.org/10.1016/j.polymer.2013.04.062 [Google Scholar] [CrossRef]
59. Huang, X., Qi, X., Boey, F., Zhang, H. (2012). Graphene-based composites. Chemical Society Reviews, 41(2), 666–686. https://doi.org/10.1039/c1cs15078b [Google Scholar] [PubMed] [CrossRef]
60. Balaji, V., Lau, K., Hui, D., Bhattacharyya, D. (2018). Graphene-based materials and their composites: A review on production, applications and product limitations. Composites Part B: Engineering, 142(1), 200–220. https://doi.org/10.1016/j.compositesb.2018.01.013 [Google Scholar] [CrossRef]
61. Novoselov, K. S., Jiang, D., Schedin, F., Booth, T., Khotkevitch, V. et al. (2005). Two-dimensional atomic crystals. Proceedings of the National Academy of Sciences, 102(30), 10451–10453. https://doi.org/10.1073/pnas.0502848102 [Google Scholar] [PubMed] [CrossRef]
62. Wolf, E. L. (2014). Applications of graphene: An overview. Springer Cham. [Google Scholar]
63. Li, J., Jiang, Z., Gan, L., Qiu, H., Yang, G. et al. (2018). Functionalized graphene/polymer composite coatings for autonomous early-warning of steel corrosion. Composites Communications, 9, 6–10. https://doi.org/10.1016/j.coco.2018.04.002 [Google Scholar] [CrossRef]
64. Ollik, K., Lieder, M. (2020). Review of the application of graphene-based coatings as anticorrosion layers. Coatings, 10(9). https://doi.org/10.3390/coatings10090883 [Google Scholar] [CrossRef]
65. Xuan Lim, C. H. Y., Sorkin, A., Bao, Q., Li, A., Zhang, K. et al. (2013). A hydrothermal anvil made of graphene nanobubbles on diamond. Nature Communications, 4, 1556. https://doi.org/10.1038/ncomms2579 [Google Scholar] [PubMed] [CrossRef]
66. Dreyer, D. R., Park, S., Bielawski, C. W., Ruoff, R. S. (2010). The chemistry of graphene oxide. Chemical Society Reviews, 39(1), 228–240. https://doi.org/10.1039/b917103g [Google Scholar] [PubMed] [CrossRef]
67. Toto, E., Laurenzi, S., Santonicola, M. G. (2022). Recent trends in graphene/polymer nanocomposites for sensing devices: Synthesis and applications in environmental and human health monitoring. Polymers, 14(5), 1030. https://doi.org/10.3390/polym14051030 [Google Scholar] [PubMed] [CrossRef]
68. He, H., Klinowski, J., Forster, M., Lerf, A. (1998). A new structural model for graphite oxide. Chemical Physics Letters, 287(1–2), 53–56. https://doi.org/10.1016/S0009-2614(98)00144-4 [Google Scholar] [CrossRef]
69. Lerf, A., He, H., Forster, M., Klinowski, J. (1998). Structure of graphite oxide revisited. Journal of Physical Chemistry B, 102(23), 4477–4482. https://doi.org/10.1021/jp9731821 [Google Scholar] [CrossRef]
70. Park, S., Ruoff, R. S. (2009). Chemical methods for the production of graphenes. Nature Nanotechnology, 4(4), 217–224. https://doi.org/10.1038/nnano.2009.58 [Google Scholar] [PubMed] [CrossRef]
71. Casabianca, L. B., Shaibat, M., Cai, W., Park, S., Piner, R. et al. (2010). NMR-Based structural modeling of graphite oxide using multidimensional 13C solid-state NMR and ab initio chemical shift calculations. Journal of the American Chemical Society, 132(16), 5672–5676. https://doi.org/10.1021/ja9030243 [Google Scholar] [PubMed] [CrossRef]
72. Naebe, M., Wang, J., Amini, A., Khayyam, H., Hameed, N. et al. (2014). Mechanical property and structure of covalent functionalised graphene/Epoxy nanocomposites. Scientific Reports, 4. https://doi.org/10.1038/srep04375 [Google Scholar] [PubMed] [CrossRef]
73. Bonaccorso, F., Sun, Z., Hasan, T., Ferrari, A. C. (2010). Graphene photonics and optoelectronics. Nature Photonics, 4(9), 611–622. https://doi.org/10.1038/nphoton.2010.186 [Google Scholar] [CrossRef]
74. Yang, Z., Tian, J., Yin, Z., Cui, C., Qian, W. et al. (2019). Carbon nanotube- and graphene-based nanomaterials and applications in high-voltage supercapacitor: A review. Carbon, 141, 467–480. https://doi.org/10.1016/j.carbon.2018.10.010 [Google Scholar] [CrossRef]
75. Kumar, C. N. S., Chakravadhanula, V. S. K., Riaz, A., Dehmn, S., Wang, D. et al. (2017). Understanding the graphitization and growth of free-standing nanocrystalline graphene using: In situ transmission electron microscopy. Nanoscale, 9(35), 12835–12842. https://doi.org/10.1039/c7nr03276e [Google Scholar] [PubMed] [CrossRef]
76. Tan, C., Zhang, H. (2015). Wet-chemical synthesis and applications of non-layer structured two-dimensional nanomaterials. Nature Communications, 6, 7873. https://doi.org/10.1038/ncomms8873 [Google Scholar] [PubMed] [CrossRef]
77. Amiri, A., Naraghi, M., Ahmadi, G., Soleymaniha, M., Shanbedi, M. (2018). A review on liquid-phase exfoliation for scalable production of pure graphene, wrinkled, crumpled and functionalized graphene and challenges. FlatChem, 8, 40–71. https://doi.org/10.1016/j.flatc.2018.03.004 [Google Scholar] [CrossRef]
78. Acik, M., Chabal, Y. J. (2012). A review on reducing graphene oxide for band Gap engineering. Journal of Materials Science Research, 2(1). https://doi.org/10.5539/jmsr.v2n1p101 [Google Scholar] [CrossRef]
79. Raccichini, R., Varzi, A., Passerini, S., Scrosati, B. (2015). The role of graphene for electrochemical energy storage. Nature Materials, 14(3). https://doi.org/10.1038/nmat4170 [Google Scholar] [PubMed] [CrossRef]
80. de Silva, K., Huang, H., Joshi, R., Yoshimura, M. (2017). Chemical reduction of graphene oxide using green reductants. Carbon, 119, 190–199. https://doi.org/10.1016/j.carbon.2017.04.025 [Google Scholar] [CrossRef]
81. Gong, X., Liu, G., Li, Y., Yu, D. Y. W., Teoh, W. Y. (2016). Functionalized-graphene composites: Fabrication and applications in sustainable energy and environment. Chemistry of Materials, 28(22), 8082–8118. https://doi.org/10.1021/acs.chemmater.6b01447 [Google Scholar] [CrossRef]
82. Boukhvalov, D. W., Katsnelson, M. I. (2009). Chemical functionalization of graphene. Journal of Physics Condensed Matter, 21(34). https://doi.org/10.1088/0953-8984/21/34/344205 [Google Scholar] [PubMed] [CrossRef]
83. Li, Z., Li, J., Cui, J., Qui, H., Yang, G. et al. (2019). Dispersion and parallel assembly of sulfonated graphene in waterborne epoxy anticorrosion coatings. Journal of Materials Chemistry A, 7(30), 17937–17946. https://doi.org/10.1039/C9TA03995C [Google Scholar] [CrossRef]
84. Zhu, Q., Li, E., Liu, X., Song, W., Li, Y. et al. (2020). Epoxy coating with in-situ synthesis of polypyrrole functionalized graphene oxide for enhanced anticorrosive performance. Progress in Organic Coatings, 140, 105488. https://doi.org/10.1016/j.porgcoat.2019.105488 [Google Scholar] [CrossRef]
85. Amin, I., Batyrev, E., De Vooys, A., Van Der Weijde, H., Shiju, N. R. (2022). Covalent polymer functionalization of graphene/graphene oxide and its application as anticorrosion materials. 2D Materials, 9(3). https://doi.org/10.1088/2053-1583/ac54ee [Google Scholar] [CrossRef]
86. Janotti, A., Wei, S. H., Singh, D. J. (2001). First-principles study of the stability of BN and C. Physical Review B: Condensed Matter and Materials Physics, 64, 174107. https://doi.org/10.1103/PhysRevB.64.174107 [Google Scholar] [CrossRef]
87. Rydberg, H., Dion, M., Jacobson, N., Schroder, E., Hyldgaard, P. et al. (2003). Van der waals density functional for layered structures. Physical Review Letters, 91, 126402. https://doi.org/10.1103/PhysRevLett.91.126402 [Google Scholar] [PubMed] [CrossRef]
88. Seifert, M., Koch, A., Deubel, F., Simmet, T., Hess, L. et al. (2013). Functional polymer brushes on hydrogenated graphene. Chemistry of Materials, 25(3), 466–470. https://doi.org/10.1021/cm3036983 [Google Scholar] [CrossRef]
89. Yu, Z., Di, H., Ma, Y., He, Y., Liang, L. et al. (2015). Preparation of graphene oxide modified by titanium dioxide to enhance the anticorrosion performance of epoxy coatings. Surface and Coatings Technology, 276, 471–478. https://doi.org/10.1016/j.surfcoat.2015.06.027 [Google Scholar] [CrossRef]
90. Xue, B., Yu, M., Liu, J., Liu, J., Li, S. et al. (2017). Corrosion protection of AA2024-T3 by sol-gel film modified with graphene oxide. Journal of Alloys and Compounds, 725, 84–95. https://doi.org/10.1016/j.jallcom.2017.05.091 [Google Scholar] [CrossRef]
91. Xu, J., Liu, J., Li, K. (2015). Application of functionalized graphene oxide in flame-retardant polypropylene. Journal of Vinyl and Additive Technology, 21(4), 278–284. https://doi.org/10.1002/vnl.21415 [Google Scholar] [CrossRef]
92. Hu, H., He, Y., Long, Z., Zhan, Y. (2017). Synergistic effect of functional carbon nanotubes and graphene oxide on the anticorrosion performance of epoxy coating. Polymers for Advanced Technologies, 28(6), 754–762. https://doi.org/10.1002/pat.3977 [Google Scholar] [CrossRef]
93. Liu, C., Qiu, S., Du, P., Zhao, H., Wang, L. (2018). An ionic liquid–graphene oxide hybrid nanomaterial: Synthesis and anticorrosive applications. Nanoscale, 10(17), 8115–8124. https://doi.org/10.1039/C8NR01890A [Google Scholar] [PubMed] [CrossRef]
94. Georgakilas, V., Otyepka, M., Bourlinos, A., Chandra, V., Kim, N. (2012). Functionalization of graphene: Covalent and noncovalent approaches, derivatives and applications. Chemical Reviews, 112(11), 6156–6214. https://doi.org/10.1021/cr3000412 [Google Scholar] [PubMed] [CrossRef]
95. Georgakilas, V., Tiwari, J., Kemp, K., Perman, J., Bourlinos, A. et al. (2016). Noncovalent functionalization of graphene and graphene oxide for energy materials, biosensing, catalytic, and biomedical applications. Chemical Reviews, 116(9), 5464–5519. https://doi.org/10.1021/acs.chemrev.5b00620 [Google Scholar] [PubMed] [CrossRef]
96. Xu, Y., Bai, H., Lu, G., Li, C. X., Shi, G. (2008). Flexible graphene films via the filtration of water-soluble noncovalent functionalized graphene sheets. Journal of the American Chemical Society, 130(18), 5856–5857. https://doi.org/10.1021/ja800745y [Google Scholar] [PubMed] [CrossRef]
97. Jaegfeldt, H., Kuwana, T., Johansson, G. (1983). Electrochemical stability of catechols with a pyrene side chain strongly adsorbed on graphite electrodes for catalytic oxidation of dihydronicotinamide adenine dinucleotide. Journal of the American Chemical Society, 105(7), 1805–1814. https://doi.org/10.1021/ja00345a021 [Google Scholar] [CrossRef]
98. Wang, Y., Chen, X., Zhong, Y., Zhu, F., Loh, K. P. (2009). Large area, continuous, few-layered graphene as anodes in organic photovoltaic devices. Applied Physics Letters, 95(6), 063302. https://doi.org/10.1063/1.3204698 [Google Scholar] [CrossRef]
99. Li, J., Cui, J., Yang, J., Li, Y., Qiu, H. et al. (2016). Reinforcement of graphene and its derivatives on the anticorrosive properties of waterborne polyurethane coatings. Composites Science and Technology, 129, 30–37. https://doi.org/10.1016/j.compscitech.2016.04.017 [Google Scholar] [CrossRef]
100. Tamilarasan, T. R., Sanjith, U., Siva Shankar, M., Rajagopal, G. (2017). Effect of reduced graphene oxide (rGO) on corrosion and erosion-corrosion behaviour of electroless Ni-P coatings. Wear, 390–391, 385–391. https://doi.org/10.1016/j.wear.2017.09.004 [Google Scholar] [CrossRef]
101. Raghupathy, Y., Kamboj, A., Rekha, M. Y., Narasimha Rao, N. P., Srivastava, C. (2017). Copper-graphene oxide composite coatings for corrosion protection of mild steel in 3.5% NaCl. Thin Solid Films, 636, 107–115. https://doi.org/10.1016/j.tsf.2017.05.042 [Google Scholar] [CrossRef]
102. Cui, G., Bi, Z., Zhang, R., Liu, J., Yu, X. et al. (2019). A comprehensive review on graphene-based anticorrosive coatings. Chemical Engineering Journal, 373, 104–121. https://doi.org/10.1016/j.cej.2019.05.034 [Google Scholar] [CrossRef]
103. Kausar, A. (2018). Corrosion prevention prospects of polymeric nanocomposites: A review. Journal of Plastic Film & Sheeting, 35(2), 181–202. https://doi.org/10.1177/8756087918806027 [Google Scholar] [CrossRef]
104. Kumar, S. S. A., Bashir, S., Ramesh, K., Ramesh, S. (2021). New perspectives on graphene/graphene oxide based polymer nanocomposites for corrosion applications: The relevance of the graphene/polymer barrier coatings. Progress in Organic Coatings, 154. https://doi.org/10.1016/j.porgcoat.2021.106215 [Google Scholar] [CrossRef]
105. Davoodi, M., Ghasemi, E., Ramezanzadeh, B., Mahdavian, M. (2020). Designing a zinc-encapsulated Feldspar as a unique rock-forming tectosilicate nanocontainer in the epoxy coating; improving the robust barrier and self-healing anticorrosion properties. Construction and Building Materials, 243. https://doi.org/10.1016/j.conbuildmat.2020.118215 [Google Scholar] [CrossRef]
106. Jin, T., Xie, Z., Fullston, D., Huang, C., Zeng, R. et al. (2019). Corrosion resistance of copolymerization of acrylamide and acrylic acid grafted graphene oxide composite coating on magnesium alloy. Progress in Organic Coatings, 136, 105222. https://doi.org/10.1016/j.porgcoat.2019.105222 [Google Scholar] [CrossRef]
107. Adrar, S., Habi, A., Ajji, A., Grohens, Y. (2018). Synergistic effects in epoxy functionalized graphene and modified organo-montmorillonite PLA/PBAT blends. Applied Clay Science, 157, 65–75. https://doi.org/10.1016/j.clay.2018.02.028 [Google Scholar] [CrossRef]
108. Mahmood, H., Vanzetti, L., Bersani, M., Pegoretti, A. (2018). Mechanical properties and strain monitoring of glass-epoxy composites with graphene-coated fibers. Composites Part A: Applied Science and Manufacturing, 107, 112–123. https://doi.org/10.1016/j.compositesa.2017.12.023 [Google Scholar] [CrossRef]
109. Lakshmanan, A., Srivastava, S., Ramazani, A., Sundararaghavan, V. (2018). Thermal conductivity of pillared graphene-epoxy nanocomposites using molecular dynamics. Applied Physics Letters, 112, 151902. https://doi.org/10.1063/1.5022755 [Google Scholar] [CrossRef]
110. Shi, Y., Yu, B., Zheng, Y., Yang, J., Duan, Z. et al. (2018). Design of reduced graphene oxide decorated with DOPO-phosphanomidate for enhanced fire safety of epoxy resin. Journal of Colloid and Interface Science, 521, 160–171. https://doi.org/10.1016/j.jcis.2018.02.054 [Google Scholar] [PubMed] [CrossRef]
111. Aradhana, R., Mohanty, S., Nayak, S. K. (2018). Comparison of mechanical, electrical and thermal properties in graphene oxide and reduced graphene oxide filled epoxy nanocomposite adhesives. Polymer, 141, 109–123. https://doi.org/10.1016/j.polymer.2018.03.005 [Google Scholar] [CrossRef]
112. Wang, J., Jin, X., Li, C., Wang, W., Wu, H. et al. (2019). Graphene and graphene derivatives toughening polymers: Toward high toughness and strength. Chemical Engineering Journal, 370, 831–854. https://doi.org/10.1016/j.cej.2019.03.229 [Google Scholar] [CrossRef]
113. Ye, Y., Chen, H., Zou, Y., Ye, Y., Zhao, H. (2020). Corrosion protective mechanism of smart graphene-based self-healing coating on carbon steel. Corrosion Science, 174, 108825. https://doi.org/10.1016/j.corsci.2020.108825 [Google Scholar] [CrossRef]
114. Zhu, Q., Li, E., Song, W., Zhao, M., Zi, L. et al. (2020). Synergistic effect of polypyrrole functionalized graphene oxide and zinc phosphate for enhanced anticorrosion performance of epoxy coatings. Composites Part A: Applied Science and Manufacturing, 130, 105752. https://doi.org/10.1016/j.compositesa.2019.105752 [Google Scholar] [CrossRef]
115. Chen, Y., Ren, B., Gao, S., Cao, R. (2020). The sandwich-like structures of polydopamine and 8-hydroxyquinoline coated graphene oxide for excellent corrosion resistance of epoxy coatings. Journal of Colloid and Interface Science, 565, 436–448. https://doi.org/10.1016/j.jcis.2020.01.051 [Google Scholar] [PubMed] [CrossRef]
116. Nguyen, T. D., Nguyen, A. S., Tran, B. A., Vu, K. O., Tran, D. L. et al. (2020). Molybdate intercalated hydrotalcite/graphene oxide composite as corrosion inhibitor for carbon steel. Surface and Coatings Technology, 399. https://doi.org/10.1016/j.surfcoat.2020.126165 [Google Scholar] [CrossRef]
117. Rajitha, K., Mohana, K. N. S., Mohanan, A., Madhusudhana, A. M. (2020). Evaluation of anticorrosion performance of modified gelatin-graphene oxide nanocomposite dispersed in epoxy coating on mild steel in saline media. Colloids and Surfaces A: Physicochemical and Engineering Aspects, 587. https://doi.org/10.1016/j.colsurfa.2019.124341 [Google Scholar] [CrossRef]
118. Liu, C., Li, J., Jin, Z., Hou, P., Zhao, H. et al. (2019). Synthesis of graphene-epoxy nanocomposites with the capability to self-heal underwater for materials protection. Composites Communications, 15, 155–161. https://doi.org/10.1016/j.coco.2019.07.011 [Google Scholar] [CrossRef]
119. Pourhashem, S., Vaezi, M. R., Rashidi, A., Bagherzadeh, M. R. (2017). Distinctive roles of silane coupling agents on the corrosion inhibition performance of graphene oxide in epoxy coatings. Progress in Organic Coatings, 111, 47–56. https://doi.org/10.1016/j.porgcoat.2017.05.008 [Google Scholar] [CrossRef]
120. Tarawneh, M. A., Saraireh, S. A., Chen, R. S., Hj Amad, S., Al-Tarawni, M. A. M. et al. (2020). Mechanical reinforcement with enhanced electrical and heat conduction of epoxy resin by polyaniline and graphene nanoplatelets. Nanotechnology Reviews, 9(1), 1550–1561. https://doi.org/10.1515/ntrev-2020-0118 [Google Scholar] [CrossRef]
121. Bao, C., Guo, Y., Song, L., Kan, Y., Qian, X. et al. (2011). In situ preparation of functionalized graphene oxide/epoxy nanocomposites with effective reinforcements. Journal of Materials Chemistry, 21(35), 13290–13298. https://doi.org/10.1039/c1jm11434d [Google Scholar] [CrossRef]
122. Deng, F., Wang, L., Zhou, Y., Gong, X., Hu, T. et al. (2017). Effect of nanosilica content on the corrosion inhibition of composite coatings of a filled epoxy resin grafted with a hydrophobic fluoroalkylsilane: A dual critical concentrations interpretation. RSC Advances, 7(77), 48876–48893. https://doi.org/10.1039/c7ra10315h [Google Scholar] [CrossRef]
123. Taheri, N. N., Ramezanzadeh, B., Mahdavian, M. (2019). Application of layer-by-layer assembled graphene oxide nanosheets/polyaniline/zinc cations for construction of an effective epoxy coating anticorrosion system. Journal of Alloys and Compounds, 800, 532–549. https://doi.org/10.1016/j.jallcom.2019.06.103 [Google Scholar] [CrossRef]
124. Zheng, H., Guo, M., Shao, Y., Wang, Y., Liu, B. et al. (2018). Graphene oxide–Poly(urea–formaldehyde) composites for corrosion protection of mild steel. Corrosion Science, 139, 1–12. https://doi.org/10.1016/j.corsci.2018.04.036 [Google Scholar] [CrossRef]
125. Conradi, M., Kocijan, A., Kek-Merl, D., Zorko, M., Verpoest, I. (2014). Mechanical and anticorrosion properties of nanosilica-filled epoxy-resin composite coatings. Applied Surface Science, 292, 432–437. https://doi.org/10.1016/j.apsusc.2013.11.155 [Google Scholar] [CrossRef]
126. Bakhshandeh, E., Jannesari, A., Ranjbar, Z., Sobhani, S., Saeb, M. R. (2014). Anticorrosion hybrid coatings based on epoxy-silica nanocomposites: Toward relationship between the morphology and EIS data. Progress in Organic Coatings, 77(7), 1169–1183. https://doi.org/10.1016/j.porgcoat.2014.04.005 [Google Scholar] [CrossRef]
127. Xie, Y., Liu, C., Liu, W., Liang, L., Wang, S. et al. (2020). A novel approach to fabricate polyacrylate modified graphene oxide for improving the corrosion resistance of epoxy coatings. Colloids and Surfaces A: Physicochemical and Engineering Aspects, 593, 124627. https://doi.org/10.1016/j.colsurfa.2020.124627 [Google Scholar] [CrossRef]
128. Jeon, H. R., Park, J. H., Shon, M. Y. (2013). Corrosion protection by epoxy coating containing multi-walled carbon nanotubes. Journal of Industrial and Engineering Chemistry, 19(3), 849–853. https://doi.org/10.1016/j.jiec.2012.10.030 [Google Scholar] [CrossRef]
129. Cui, M., Ren, S., Zhao, H., Xue, Q., Wang, L. (2018). Polydopamine coated graphene oxide for anticorrosive reinforcement of waterborne epoxy coating. Chemical Engineering Journal, 335, 255–266. https://doi.org/10.1016/j.cej.2017.10.172 [Google Scholar] [CrossRef]
130. Li, Y., Ambrogi, V., Cerruti, P., Goswami, M., Yang, Z. et al. (2021). Functional liquid crystalline epoxy networks and composites: From materials design to applications. International Materials Reviews, 67, 201–229. https://doi.org/10.1080/09506608.2021.1937811 [Google Scholar] [CrossRef]
131. Ramaswamy, R., Shine, K. G. (1987). Some aspects on the synthesis and characterization of dodecenyl succinic anhydride (DDSA)-A curing agent for epoxy resins. Journal of Applied Polymer Science, 33, 49–65. https://doi.org/10.1002/app.1987.070330105 [Google Scholar] [CrossRef]
132. Yoshida, S. (2014). Quantitative evaluation of an epoxy resin dispersion by infrared spectroscopy. Polymer Journal, 46(7), 430–434. https://doi.org/10.1038/pj.2014.15 [Google Scholar] [CrossRef]
133. Mohan, P. (2010). Comprehensive study of metal on the characteristic properties of epoxy resins. Journal of Applied Polymer Science, 116(6), 3271–3277. https://doi.org/10.1002/app.31867 [Google Scholar] [CrossRef]
134. Srivastava, A., Rai, J. S. P. (2005). Synthesis, characterization and curing behaviour of partial esters of cycloaliphatic epoxy resins. Designed Monomers and Polymers, 8(4), 319–334. https://doi.org/10.1163/1568555054460097 [Google Scholar] [CrossRef]
135. Kimura, H., Matsumoto, A., Ohtsuka, K. (2009). New type of phenolic resin: Curing reaction of phenol-novolac based benzoxazine with bisoxazoline or epoxy resin using latent curing agent and the properties of the cured resin. Journal of Applied Polymer Science, 112(3), 1762–1770. https://doi.org/10.1002/app.29301 [Google Scholar] [CrossRef]
136. Vijayan, P., Puglia, P. D., Al-Maadeed, M. A. S. A., Kenny, J. M., Thomas, S. (2017). Elastomer/thermoplastic modified epoxy nanocomposites: The hybrid effect of “micro” and “nano” scale. Materials Science and Engineering R: Reports, 116, 1–29. https://doi.org/10.1016/j.mser.2017.03.001 [Google Scholar] [CrossRef]
137. Jin, F. L., Li, X., Park, S. J. (2015). Synthesis and application of epoxy resins: A review. Journal of Industrial and Engineering Chemistry, 29, 1–11. https://doi.org/10.1016/j.jiec.2015.03.026 [Google Scholar] [CrossRef]
138. Dhand, V., Prasad, J. S., Rhee, K. Y., Anjaneyulu, Y. (2013). Fabrication of high pressure hydrogen adsorption/desorption unit-adsorption study on flame synthesized carbon nanofibers. Journal of Industrial and Engineering Chemistry, 19(3), 944–949. https://doi.org/10.1016/j.jiec.2012.11.013 [Google Scholar] [CrossRef]
139. Yao, S. S., Jiang, D., Zhang, Q. Z., Wang, X. X. (2020). Preparation and properties of graphite/epoxy resin conductive composites. IOP Conference Series: Materials Science and Engineering, 740(1), 012064. https://doi.org/10.1088/1757-899X/740/1/012064 [Google Scholar] [CrossRef]
140. Wang, C., Ji, X., Roy, A., Silberschmidt, V. V., Chen, Z. (2015). Shear strength and fracture toughness of carbon fibre/epoxy interface: Effect of surface treatment. Materials & Design, 85, 800–807. https://doi.org/10.1016/j.matdes.2015.07.104 [Google Scholar] [CrossRef]
141. Chonkaew, W., Sombatsompop, N., Brostow, W. (2013). High impact strength and low wear of epoxy modified by a combination of liquid carboxyl terminated poly(butadiene-co-acrylonitrile) rubber and organoclay. European Polymer Journal, 49(6), 1461–1470. https://doi.org/10.1016/j.eurpolymj.2013.03.022 [Google Scholar] [CrossRef]
142. Hassan, M. S., El-Nemr, K. F. (2013). Dye sorption characters of gamma irradiated foamed ethylene propylene diene monomer (EPDM) rubber/clay composites. Journal of Industrial and Engineering Chemistry, 19(4), 1371–1376. https://doi.org/10.1016/j.jiec.2012.12.042 [Google Scholar] [CrossRef]
143. Mohamed, R. M. (2013). Radiation induced modification of NBR and SBR montmorillonite nanocomposites. Journal of Industrial and Engineering Chemistry, 19(1), 80–86. https://doi.org/10.1016/j.jiec.2012.07.005 [Google Scholar] [CrossRef]
144. Chen, Y., Chia, J. Y. H., Su, Z. C., Tay, T. E., Tan, V. B. C. (2013). Mechanical characterization of interfaces in epoxy-clay nanocomposites by molecular simulations. Polymer, 54(2), 766–773. https://doi.org/10.1016/j.polymer.2012.11.040 [Google Scholar] [CrossRef]
145. Domun, N., Hadavinia, H., Zhang, T., Sainsbury, T., Liaghat, G. H. et al. (2015). Improving the fracture toughness and the strength of epoxy using nanomaterials–A review of the current status. Nanoscale, 7(23), 10294–10329. https://doi.org/10.1039/c5nr01354b [Google Scholar] [PubMed] [CrossRef]
146. Rosetti, Y., Alcouffe, P., Pascault, J. P., Gérard, J. F., Lortie, F. (2018). Polyether sulfone-based epoxy toughening: From micro- to nano-phase separation via PES end-chain modification and process engineering. Materials, 11(10). https://doi.org/10.3390/ma11101960 [Google Scholar] [PubMed] [CrossRef]
147. Hsieh, T. H., Kinloch, A. J., Masania, K., Taylor, A. C., Sprenger, S. (2010). The mechanisms and mechanics of the toughening of epoxy polymers modified with silica nanoparticles. Polymer, 51(26), 6284–6294. https://doi.org/10.1016/j.polymer.2010.10.048 [Google Scholar] [CrossRef]
148. Ounaies, Z., Sun, L. H., Gao, X. L., Whalen, C. A., Yang, Z. G. (2011). Preparation, characterization, and modeling of carbon nanofiber/epoxy nanocomposites. Journal of Nanomaterials, 2011. https://doi.org/10.1155/2011/307589 [Google Scholar] [CrossRef]
149. Zappalorto, M., Salviato, M., Quaresimin, M. (2013). Mixed mode (I + II) fracture toughness of polymer nanoclay nanocomposites. Engineering Fracture Mechanics, 111, 50–64. https://doi.org/10.1016/j.engfracmech.2013.09.006 [Google Scholar] [CrossRef]
150. Gojny, F. H., Wichmann, M. H. G., Köpke, U., Fiedler, B., Schulte, K. (2004). Carbon nanotube-reinforced epoxy-composites: Enhanced stiffness and fracture toughness at low nanotube content. Composites Science and Technology, 64(15), 2363–2371. https://doi.org/10.1016/j.compscitech.2004.04.002 [Google Scholar] [CrossRef]
151. Li, Z., Young, R., Wang, R., Yang, F., Hao, L. (2013). The role of functional groups on graphene oxide in epoxy nanocomposites. Polymer, 54(21), 5821–5829. https://doi.org/10.1016/j.polymer.2013.08.026 [Google Scholar] [CrossRef]
152. Azimi, R., Roghani-Mamaqani, H., Gholipour-Mahmoudalilou, M. (2017). Grafting poly (amidoamine) dendrimer-modified silica nanoparticles to graphene oxide for preparation of a composite and curing agent for epoxy resin. Polymer, 126, 152–161. https://doi.org/10.1016/j.polymer.2017.08.037 [Google Scholar] [CrossRef]
153. Roghani-Mamaqani, H., Khezri, K. (2016). Polystyrene-attached graphene nanolayers by reversible addition-fragmentation chain transfer polymerization: A grafting from epoxy groups with various densities. Journal of Polymer Research, 23(9). https://doi.org/10.1007/s10965-016-1082-6 [Google Scholar] [CrossRef]
154. Wan, Y. J., Tang, L. C., Yan, D., Zhao, L., Li, Y. B. et al. (2013). Improved dispersion and interface in the graphene/epoxy composites via a facile surfactant-assisted process. Composites Science and Technology, 82, 60–68. https://doi.org/10.1016/j.compscitech.2013.04.009 [Google Scholar] [CrossRef]
155. Yousefi, N., Lin, X., Zheng, Q., Shen, X., Pothnis, J. R. et al. (2013). Simultaneous in situ reduction, self-alignment and covalent bonding in graphene oxide/epoxy composites. Carbon, 59, 406–417. https://doi.org/10.1016/j.carbon.2013.03.034 [Google Scholar] [CrossRef]
156. Wang, J., Du, P., Zhao, H., Pu, J., Yu, C. (2019). Novel nitrogen doped carbon dots enhancing the anticorrosive performance of waterborne epoxy coatings. Nanoscale Advances, 1(9), 3443–3451. https://doi.org/10.1039/c9na00155g [Google Scholar] [PubMed] [CrossRef]
157. Yu, Z., Di, H., Ma, Y., Lv, L., Pan, Y. et al. (2015). Fabrication of graphene oxide-alumina hybrids to reinforce the anticorrosion performance of composite epoxy coatings. Applied Surface Science, 351, 986–996. https://doi.org/10.1016/j.apsusc.2015.06.026 [Google Scholar] [CrossRef]
158. Meng, F., Zhang, T., Liu, L., Cui, Y., Wang, F. (2019). Failure behaviour of an epoxy coating with polyaniline modified graphene oxide under marine alternating hydrostatic pressure. Surface and Coatings Technology, 361, 188–195. https://doi.org/10.1016/j.surfcoat.2019.01.037 [Google Scholar] [CrossRef]
159. Tian, Y., Xie, Y., Dai, F., Huang, H., Zhong, L. et al. (2020). Ammonium-grafted graphene oxide for enhanced corrosion resistance of waterborne epoxy coatings. Surface and Coatings Technology, 383, 125227. https://doi.org/10.1016/j.surfcoat.2019.125227 [Google Scholar] [CrossRef]
160. Wu, Y., He, Y., Chen, C., Zhong, F., Li, H. et al. (2020). Non-covalently functionalized boron nitride by graphene oxide for anticorrosive reinforcement of waterborne epoxy coating. Colloids and Surfaces A: Physicochemical and Engineering Aspects, 587, 124337. https://doi.org/10.1016/j.colsurfa.2019.124337 [Google Scholar] [CrossRef]
161. Zhao, Z., Guo, L., Feng, L., Lu, H., Xu, Y. et al. (2019). Polydopamine functionalized graphene oxide nanocomposites reinforced the corrosion protection and adhesion properties of waterborne polyurethane coatings. European Polymer Journal, 120. https://doi.org/10.1016/j.eurpolymj.2019.109249 [Google Scholar] [CrossRef]
162. Ramezanzadeh, B., Ghasemi, E., Mahdavian, M., Changizi, E., Moghadam, M. H. M. (2015). Covalently-grafted graphene oxide nanosheets to improve barrier and corrosion protection properties of polyurethane coatings. Carbon, 93, 555–573. https://doi.org/10.1016/j.carbon.2015.05.094 [Google Scholar] [CrossRef]
163. Zheng, H., Shao, Y., Wang, Y., Meng, G., Liu, B. (2017). Reinforcing the corrosion protection property of epoxy coating by using graphene oxide–Poly (urea–formaldehyde) composites. Corrosion Science, 123, 267–277. https://doi.org/10.1016/j.corsci.2017.04.019 [Google Scholar] [CrossRef]
164. Ramezanzadeh, B., Haeri, Z., Ramezanzadeh, M. (2016). A facile route of making silica nanoparticles-covered graphene oxide nanohybrids (SiO2-GO); fabrication of SiO2-GO/epoxy composite coating with superior barrier and corrosion protection performance. Chemical Engineering Journal, 303, 511–528. https://doi.org/10.1016/j.cej.2016.06.028 [Google Scholar] [CrossRef]
165. Zhu, X., Ni, Z., Dong, L., Yang, Z., Cheng, L. et al. (2019). In-situ modulation of interactions between polyaniline and graphene oxide films to develop waterborne epoxy anticorrosion coatings. Progress in Organic Coatings, 133, 106–116. https://doi.org/10.1016/j.porgcoat.2019.04.016 [Google Scholar] [CrossRef]
166. Rajitha, K., Mohana, K. N. (2020). Application of modified graphene oxide–polycaprolactone nanocomposite coating for corrosion control of mild steel in saline medium. Materials Chemistry and Physics, 241, 122050. https://doi.org/10.1016/j.matchemphys.2019.122050 [Google Scholar] [CrossRef]
167. Kalliola, S., Repo, E., Sillanpää, M., Singh Arora, J., He, J. et al. (2016). The stability of green nanoparticles in increased pH and salinity for applications in oil spill-treatment. Colloids and Surfaces A: Physicochemical and Engineering Aspects, 493, 99–107. https://doi.org/10.1016/j.colsurfa.2016.01.011 [Google Scholar] [CrossRef]
168. Zarras, P., Stenger-Smith, J. D. (2015). Smart inorganic and organic pretreatment coatings for the inhibition of corrosion on metals/alloys, pp. 59–91. Butterworth-Heinemann: Elsevier Inc. https://doi.org/10.1016/B978-0-12-411467-8.00003-9 [Google Scholar] [CrossRef]
169. Chen, B., Wu, Q., Li, J., Lin, K., Chen, D. et al. (2020). A novel and green method to synthesize a epoxidized biomass eucommia gum as the nanofiller in the epoxy composite coating with excellent anticorrosive performance. Chemical Engineering Journal, 379, 122323. https://doi.org/10.1016/j.cej.2019.122323 [Google Scholar] [CrossRef]
Cite This Article
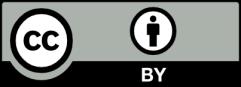
This work is licensed under a Creative Commons Attribution 4.0 International License , which permits unrestricted use, distribution, and reproduction in any medium, provided the original work is properly cited.