Open Access
ARTICLE
Optimization of Preparation of Fe3O4-L by Chemical Co-Precipitation and Its Adsorption of Heavy Metal Ions
College of Civil Engineering, Liaoning Technical University, Fuxin, 123000, China
* Corresponding Author: Xueying Sun. Email:
(This article belongs to the Special Issue: Porous Materials for Sustainable Development)
Journal of Renewable Materials 2023, 11(5), 2209-2232. https://doi.org/10.32604/jrm.2023.025241
Received 30 June 2022; Accepted 16 August 2022; Issue published 13 February 2023
Abstract
To address the serious pollution of heavy metals in AMD, the difficulty and the high cost of treatment, Fe3O4-L was prepared by the chemical co-precipitation method. Based on the single-factor and RSM, the effects of particle size, total Fe concentration, the molar ratio of Fe2+ to Fe3+ and water bath temperature on the removal of AMD by Fe3O4-L prepared by chemical co-precipitation method were analyzed. Static adsorption experiments were conducted on Cu2+, Zn2+ and Pb2+ using Fe3O4-L prepared under optimal conditions as adsorbents. The adsorption properties and mechanisms were analyzed by combining SEM-EDS, XRD and FTIR for characterization. The study showed that the effects of particle size, total Fe concentration and the molar ratio of Fe2+ to Fe3+ are larger. Obtained by response surface optimization analysis, the optimum conditions for the preparation of Fe3O4-L were a particle size of 250 mesh, a total Fe concentration of 0.5 mol/L, and a molar ratio of Fe2+ to Fe3+ of 1:2. Under these conditions, the removal rates of Cu2+, Zn2+, and Pb2+ were 94.52%, 88.49%, and 96.69% respectively. The adsorption of Cu2+, Zn2+ and Pb2+ by Fe3O4-L prepared under optimal conditions reached equilibrium at 180 min, with removal rates of 99.99%, 85.27%, and 97.48%, respectively. The adsorption reaction of Fe3O4-L for Cu2+ and Zn2+ is endothermic, while that for Pb2+ is exothermic. Fe3O4-L can still maintain a high adsorption capacity after five cycles of adsorption-desorption experiments. Cu2+, Zn2+ and Pb2+ mainly exist as CuFe2O4, Zn(OH)2, ZnFe2O4 and PbS after being adsorbed by Fe3O4-L, which is the result of the combination of physical diffusion, ion exchange and surface complexation reaction.Graphic Abstract
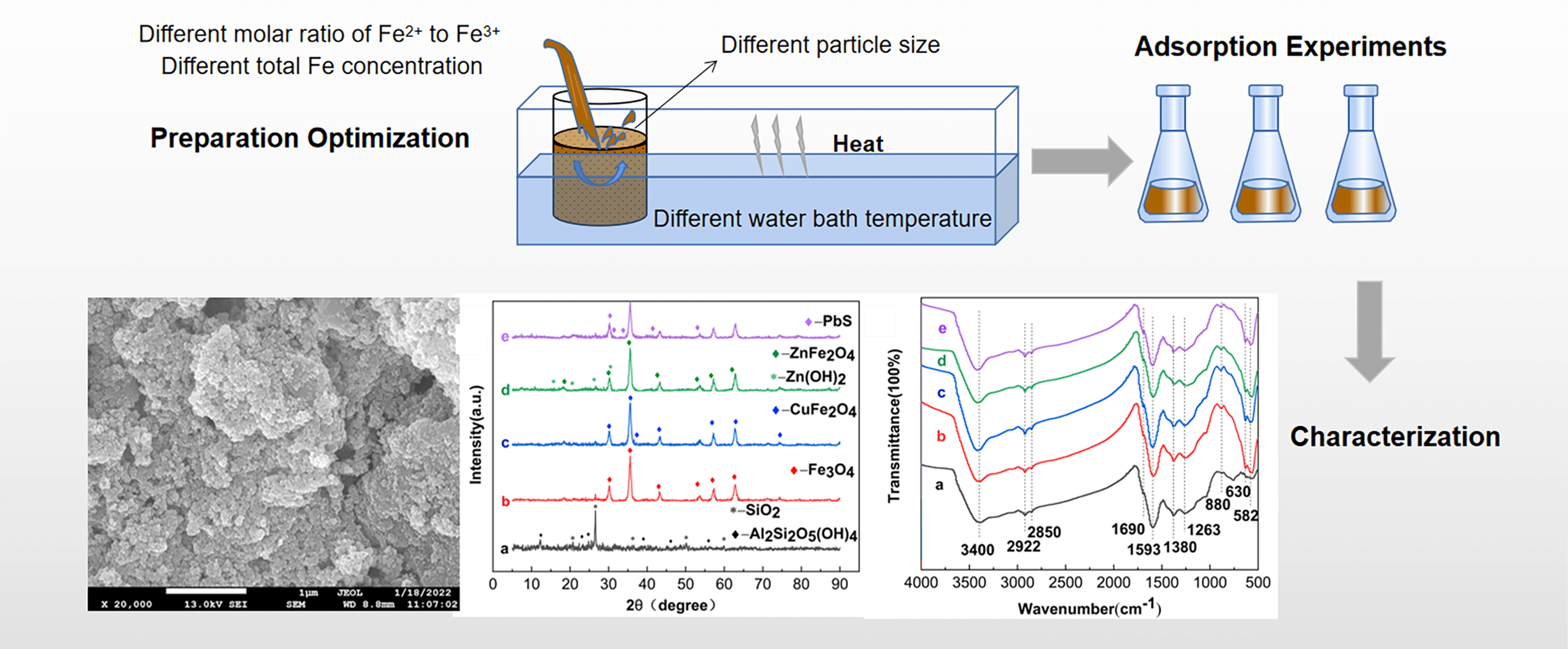
Keywords
With the increasing demand for industrial society development and the increasing use of mineral resources, Acid Mine Drainage (AMD) from coal mining has increased dramatically. AMD is characterized by low pH and high heavy metal ion content. The heavy metal ions such as Cu2+, Zn2+ and Pb2+ have a continuous and cumulative impact on the environment. If improperly treated and discharged, they will seriously pollute waters and negatively affect the ecological environment [1,2]. The adsorption method is widely used in the treatment of AMD because of its simple design, easy operation and high economic efficiency [3]. The choice of adsorbent is very important when using the adsorption method to treat AMD. Thus, the cost, adsorption capacity and post-use recycling of it should be reasonably considered when selecting the adsorbent [4].
Common natural adsorbents include activated carbon, zeolite, bentonite and lignite, etc. Yin et al. [5] designed response surface experiments to optimize the preparation and adsorption conditions of activated carbon. The optimum preparation conditions of impregnation ratio, activation time and activation temperature were 2.3, 100 min and 510°C, respectively, and the optimum adsorption capacity for Pb2+ was 42.85 mg/g. Activated carbon performs poorly in treating wastewater containing heavy metal ions, so a chelating agent is needed to improve its performance, which will greatly increase the cost [3]. Lu et al. [3] proposed in a study on the adsorption and thermal stability of zeolites on Pb2+ and Cu2+ that zeolites are inexpensive and porous media that facilitate effective removal of heavy metal ions, but so far the problem of treatment of second-hand zeolite dealing with heavy metal ions has not been solved. Karapinar et al. [6] studied the adsorption behavior of natural bentonite for Cd2+ and Cu2+, and carried out thermodynamic analysis under the optimized conditions. The results show that natural bentonite can effectively adsorb Cd2+ and Cu2+ from an aqueous solution. Olu-Owolabi et al. [7] modified bentonite with sulfate and phosphate to enhance the ion exchange capacity and improve the adsorption of Zn2+ and Cu2+. Bentonite can effectively adsorb heavy metal ions, but powdered bentonite will be mixed into mud when put into AMD, which is difficult to separate and recover. Lignite has the advantage of wide sources and low prices due to its large reserves and lower market prices than other coal-based fuels [8,9]. Lignite is considered as a good adsorbent because of its low density, large specific surface area and a large number of active groups that can chelate and complex with heavy metal ions. Chu et al. [10] studied the adsorption effect of lignite for Hg and As with kinetic fitting, and the results showed that the adsorption capacities of lignite for Hg and As were 6.8995 and 7.3995 mg/g, respectively, and the SEM characterization analysis showed that the pore structure of lignite was favorable for the adsorption of Hg and As. However, Di et al. [11] investigated the adsorption effect of native lignite and NaCl-modified lignite on Fe2+ and Mn2+, respectively, and the results showed that the adsorption effect of unmodified lignite on Fe2+ and Mn2+ was average, while the removal rates of Fe2+ and Mn2+ by NaCl-modified lignite was significantly increased by 28.44% and 22.47% compared with that of unmodified lignite. Therefore, in order to achieve the desired effect, modification of the native lignite is required [12,13]. There are many ways to modify lignite, and considering the recycling problem, we have to find a modification method that is easy to separate after adsorption [1]. Magnetic modification technology can effectively improve the adsorption effect of materials, and the separated lignite can be reused after desorption and regeneration. The preparation methods of magnetic modified materials mainly include the co-precipitation method and the hydrothermal method [14–16]. Mahmud et al. [17] compared the properties of Fe3O4 particles prepared by chemical co-precipitation and hydrothermal methods. The particle size and thermal stability of Fe3O4 particles prepared by two methods were studied by particle size analysis and thermogravimetric analysis. The results showed that Fe3O4 particles with small particle size and high thermal stability can be more easily obtained by chemical co-precipitation method. The chemical co-precipitation method is to prepare magnetic materials by mixing Fe2+ and Fe3+ iron salts in an alkaline aqueous medium [18]. The method can obtain Fe3O4 particles with better quality, higher yield and controllable performance according to different chemical environments without the need for special chemicals and complex and harmful procedures [15,19–21]. Therefore, the chemical co-precipitation method is the most suitable, the most widely used and the most promising method to synthesize Fe3O4 particles [22].
In this paper, Fe3O4-loaded lignite (Fe3O4-L) was prepared based on chemical co-precipitation method. The effect of Fe3O4-L prepared under different reaction conditions on the removal of Cu2+, Zn2+ and Pb2+ from AMD was investigated by single-factor experiments, and the factors with greater influence were analyzed and screened. The optimal preparation conditions of Fe3O4-L were optimized by response surface methodology (RSM). Static adsorption experiments were conducted on Cu2+, Zn2+ and Pb2+ using Fe3O4-L prepared under optimal conditions as adsorbent to obtain the removal rate laws for Fe3O4-L on Cu2+, Zn2+ and Pb2+ at different initial concentrations for each temperature system and at different reaction times, and to investigate the effect of optimized Fe3O4-L for adsorption on Cu2+, Zn2+ and Pb2+. The lignite before and after modification and Fe3O4-L before and after adsorption were characterized by SEM-EDS, XRD and FTIR, and the mechanism of Fe3O4-L adsorption of Cu2+, Zn2+ and Pb2+ was explored.
2.1 Experimental Materials and Water Samples
The lignite from Shanxi Fuhong Mineral Products Co., Ltd. (China) is crushed and passed through 150 mesh, 200 mesh and 250 mesh sieves respectively, deionized water is washed 2–3 times and then dried at 80°C. 200 mL iron solution with a total iron concentration of 0.7 mol/L was prepared according to the Fe2+ to Fe3+ molar ratio of 1:2 and heated in a constant temperature water bath at 60°C. 10 g of lignite was added into the iron solution and stirred at 350 r/min for 1 h. The concentrated ammonia water with a mass fraction of 25% was added drop by drop to a pH value of 9, continued stirring for 1 h, and then stood for 2 h. The precipitate was cleaned with deionized water, separated from solid and liquid by a rubidium magnet, repeatedly cleaned to the supernatant as neutral, dried in a vacuum for 12 h, and taken out. The sample solutions were prepared with analytically pure FeSO4⋅7H2O, Fe2(SO4)3, CuSO4⋅5H2O, ZnSO4⋅7H2O, Pb(NO3)2 and NaOH. The concentration of the sample solution was prepared according to the actual AMD water quality parameters in a mining area in Huludao: Cu2+ concentration was 30 mg/L, Zn2+ concentration was 30 mg/L, Pb2+ concentration was 50 mg/L, and pH was 4.
2.2 Detection Indicators and Methods
In this experiment, the concentrations of Cu2+, Zn2+ and Pb2+ were measured by a Z-2000 flame atomic spectrophotometer (GB/T 7475-2015), and the pH was measured by PHS-3C pH meter through the glass electrode method (GB/T 6920-2015).
Previous studies have shown that the main influencing factors for the preparation of Fe3O4 particles by chemical co-precipitation are particle size, the ratio of Fe2+ to Fe3+ species, total iron concentration, water bath temperature, type of precipitant and pH [22,23]. When Fe3O4 particles are prepared by chemical co-precipitation, Fe2+ can be completely precipitated only when the pH reaches 8.9. Therefore, the preparation of Fe3O4 particles by chemical co-precipitation method needs to add an alkaline precipitant to adjust the pH [24]. Common precipitants include NaOH and NH3·H2O. NaOH is a strong base and has a high alkali concentration of −OH, which is beneficial to the growth of the cell nucleus and can improve the speed of a chemical reaction, but the particle size of the generated Fe3O4 particles grows too fast and is prone to agglomeration [24,25]. NH3·H2O is a weak base, and its reaction rate is slightly slower than that of NaOH, which can better control the particle size of Fe3O4. Generally, NH3·H2O is used as the precipitant. A large number of research results show that pH will have a certain influence on the magnetic properties of Fe3O4 particles. When the pH value is 9, the prepared Fe3O4 particles have the best magnetic properties [24,26]. For lignite: the active ingredient HA in lignite will continuously dissolve under low alkalinity, which is beneficial to adsorption. However, HA will be degraded into small molecules under strong alkalinity, which reduces the chelating ability of metal ions and affects the inhibitory effect of HA on the release of metals in adsorption products [27,28]. Therefore, in this study, NH3·H2O was chosen as the precipitant to prepare Fe3O4-L under the condition of pH = 9.
Fe3O4-L is prepared by loading Fe3O4 particles on the lignite matrix. The performance of Fe3O4-L is affected by the loading capacity of lignite and the properties of Fe3O4 particles. The particle size of lignite is different, the surface area is different, and the loading amount of Fe3O4 particles on the lignite surface is also different. Therefore, the particle size of lignite should be discussed as a single factor of preparation conditions. When Fe3O4 particles are prepared by chemical co-precipitation, the particle size and magnetic properties of Fe3O4 particles can be strictly controlled by changing the reaction temperature [23]. The desired magnetization and nanoparticle size can be achieved by adjusting the concentration of iron salts in the solution and the molar ratio of Fe2+ to Fe3+ [22,24]. Therefore, the ratio of the amount of Fe2+ to Fe3+, the total iron concentration, and the temperature of the water bath should also be considered as single factors of the preparation conditions.
In order to prepare Fe3O4-L adsorption materials with excellent performance, NH3·H2O was chosen as the precipitant in this study, and the preparation conditions were explored under the condition of pH = 9. A single-factor experimental method was used to investigate the effects of particle size, the molar ratio of Fe2+ to Fe3+, total Fe concentration and water bath temperature on the removal performance of Cu2+, Zn2+ and Pb2+ under a single metal system in AMD-treated with Fe3O4-L.
Effect of particle size: Fe3O4-L was prepared in a constant temperature water bath at 60°C in the molar ratio of Fe2+ to Fe3+ of 1:2 and a total Fe concentration of 0.7 mol/L. The prepared Fe3O4-L was crushed and screened to 150 mesh, 200 mesh and 250 mesh. A 250 mL, pH = 4 solutions containing 30 mg/L Cu2+, 30 mg/L Zn2+, and 50 mg/L Pb2+ were prepared, respectively. 1.0 g of 150-mesh, 200-mesh, and 250-mesh Fe3O4-L were weighed and added to Cu2+, Zn2+ and Pb2+ solutions, respectively. Three replicates of each experiment were made. The adsorption was shaken at 150 r/min and samples were collected every 30 min to detect the concentrations of Cu2+, Zn2+ and Pb2+ in different Fe3O4-L particle size systems.
The following experimental procedures for the preparation of Fe3O4-L to remove Cu2+, Zn2+ and Pb2+ are the same as the above experiments except that the materials of Fe3O4-L are different. Effect of the molar ratio of Fe2+ to Fe3+: The Fe3O4-L with 250 mesh particle size was prepared in a constant temperature water bath at 60°C according to the molar ratio of Fe2+ to Fe3+ of 1:1, 1:1.5 and 1:2, respectively, and the total Fe concentration of 0.7 mol/L. Effect of total Fe concentration: The Fe3O4-L with a particle size of 250 mesh was prepared in a constant temperature water bath at 60°C according to the molar ratio of Fe2+ to Fe3+ of 1:2 and the total iron concentration of 0.5, 0.7 and 0.9 mol/L, respectively. Effect of water bath temperature: The Fe3O4-L with a particle size of 250 mesh was prepared in a constant temperature water bath of 50°C, 60°C and 70°C with the molar ratio of Fe2+ to Fe3+ of 1:2 and the total Fe concentration as 0.7 mol/L, respectively.
3.2 Response Surface Experiments
In this study, the Box-Benhnken method (BBD) was used to design response surface experiments using the results of single-factor experiments. Three independent variables, particle size (A), total Fe concentration (B) and the molar ratio of Fe2+ to Fe3+ (C), were used as response factors, which were varied at low (−1), medium (0) and high (1) levels, respectively, and the removal rates of Cu2+, Zn2+ and Pb2+ from the experimental sample solutions were used as response values (Y) to design 17 sets of experiments. The response experimental design scheme and the results are given in Table 1. The second-order polynomial model was fitted using Design-Expert software, as in Eq. (1), to obtain the regression equation. Statistical significance of the model was assessed by analysis of variance (ANOVA), and variable interaction effects were analyzed using response surface plots [5,24].
where, Y is the system response value; β0 is the offset factor of the offset term; βi is the linear offset coefficient; βii is the second-order offset coefficient; βij is the interaction effect coefficient; Xi, Xj and XiXj are the main and interaction effects of each factor for each factor level value analysis [29].
3.3 Adsorption of Cu2+, Zn2+ and Pb2+ by Fe3O4-L
Fe3O4-L was prepared for the adsorption of Pb2+, Cu2+, and Zn2+ according to the optimal results of RSM. To investigate the adsorption effect of Fe3O4-L at different reaction times, 1.0 g Fe3O4-L was injected into the solutions of Cu2+ (30 mg/L), Zn2+ (30 mg/L) and Pb2+ (50 mg/L) at pH = 4, respectively. Adsorption by shaking at 150 r/min. The remaining ion concentrations were measured at 5, 10, 15, 30, 45, 60, 90, 120, 150 and 180 min. Each experiment was repeated three times. To investigate the removal effect of Fe3O4-L on metal ions at different initial concentrations in different temperature systems, 1.0 g Fe3O4-L was added to different concentrations of Cu2+ solution (10, 20, 30, 40, 50 mg/L), Zn2+ solution (10, 20, 30, 40, 50 mg/L) at pH = 4, and Pb2+ solution (10, 20, 30, 50, 70 mg/L) at pH = 4. The adsorption was carried out at temperatures of 25°C, 35°C and 45°C, respectively, for 180 min at an oscillation speed of 150 r/min. Each experiment was repeated three times. The residual Cu2+, Zn2+ and Pb2+ concentrations were detected at different initial concentrations in different temperature systems.
In order to explore the reusability of Fe3O4-L, adsorption-desorption cycle experiments were carried out. Add 1.0 g Fe3O4-L to Cu2+ (30 mg/L), Zn2+ (30 mg/L), and Pb2+ (50 mg/L) solutions at pH = 4, and shake at 150 r/min for 180 min at 25°C. The remaining Cu2+, Zn2+, and Pb2+ concentrations in the supernatant were detected. Then, Fe3O4-L after adsorption of Cu2+, Zn2+, and Pb2+ was added to 250 mL of 0.1 mol/L HNO3 and desorbed by shaking at 150 r/min for 180 min at 25°C to achieve the desorption of Cu2+, Zn2+, and Pb2+. The desorbed Fe3O4-L was washed with deionized water until neutral, dried under vacuum for 12 h, and the above adsorption-desorption process was repeated five times. Each group of experiments was repeated three times.
A scanning electron microscope (JSM-7610Plus, Japan) was used to observe the surface morphology of lignite as well as Fe3O4-L. The lignite and Fe3O4-L adsorbent before and after adsorption of metal ions were analyzed for elemental composition testing using an UltraDry EDS detector (Thermo ScientificTM, USA). The changes in the composition and surface functional groups of Fe3O4-L adsorbent before and after modification and adsorption were determined by an X-ray diffractometer (Shimadzu XRD-6100, Japan) and Fourier transform infrared spectrometer (Thermo Fisher Scientific IS10, USA).
4.1 Analysis of Single-Factor Experimental Results
The removal rates of Cu2+, Zn2+ and Pb2+ by the prepared Fe3O4-L gradually rise with the increase of the particle size mesh (Figs. 1a–1c). The growth in particle size leads to an increase in the specific surface area and porosity of Fe3O4-L particles, which leads to an increase in the adsorption activity sites and facilitates the adsorption reaction.
Figure 1: Effect of preparation conditions of Fe3O4-L on the removal of Cu2+, Zn2+ and Pb2+. (a–c) Particle size (Fe2+:Fe3+=1:2, total iron concentration = 0.7 mol/L, water bath temperature = 60°C) (d–f) Molar ratio of Fe2+ to Fe3+ (Particle size = 250 mesh, total iron concentration = 0.7 mol/L, water bath temperature = 60°C) (g–i) Total Fe concentration (Particle size = 250 mesh, Fe2+:Fe3+ = 1:2, water bath temperature = 60°C) (k–l) Water bath temperature (Particle size = 250 mesh, Fe2+:Fe3+ = 1:2, total iron concentration = 0.7 mol/L)
The removal rates of Cu2+, Zn2+ and Pb2+ by Fe3O4-L gradually increase as the ratio of Fe2+ to Fe3+ substance decreases (Figs. 1d–1f). The Fe3+ content affects the purity and porosity of Fe3O4-L. When the Fe3+ content in the solution is low, the prepared Fe3O4-L has more impurities and low purity. In the process of loading Fe3O4, the dissolution of Fe3+ will lead to the increase of H+ content in the solution, resulting in the decomposition of some components of lignite that can produce new pore channels and increase the surface area and porosity [15,23,30]. The content of Fe2+ affects the Fe3O4 nanocrystal properties and yields. The increase of Fe2+ facilitates the reaction to produce Fe3O4 nanocrystals with larger particle size and better crystallinity, but the low yield leads to the reduction of Fe3O4 particles loaded on the surface of lignite, which affects the adsorption performance [23,24,30].
The removal rates of Cu2+, Zn2+ and Pb2+ by Fe3O4-L show an increasing and then decreasing trend with the increase of total Fe concentration (Figs. 1g–1i). The total concentration of iron salts affects the crystallization of Fe3O4 nanoparticles. The process of preparing nanoparticles by chemical co-precipitation is divided into two stages: the crystal nucleation stage and the grain growth stage [31]. The iron salt concentration is low mainly in the nucleation stage, generating a large number of nuclei. However, the particle size of the product at this time is small, and the adsorption effect of Fe3O4-L is minimal. With the increase of iron salt concentration, the grain growth stage is entered. The grain growth rate is accelerated, the product particle size, surface area and porosity increase, and the adsorption capacity is strong. The total concentration of iron salts is getting higher and higher, and the iron salts react rapidly to generate nuclei after adding precipitant and grow continuously, with a large specific surface area and more adsorption sites. However, when the concentration is already too high, the generated Fe3O4 agglomerates seriously, and the pores of Fe3O4-L are gradually blocked, which affects the adsorption effect [32].
The removal of all three metal ions by Fe3O4-L increases with increasing reaction temperature, but then decreases when the temperature is too high (Figs. 1j–1l). The higher the reaction temperature, the greater the crystal growth rate and the larger the product particle size, which provides more adsorption sites and facilitates adsorption. However, the higher temperature tends to lead to crystal aggregation and blockage of Fe3O4-L pores, which is not conducive to adsorption [31]. Temperature affects the purity of Fe3O4-L. When the temperature increases, Fe2+ is easily oxidized to Fe3+, and it is difficult to control the molar ratio of Fe2+ to Fe3+, which leads to the increase of impurities in the product and reduces the adsorption capacity. Temperature affects the stability of Fe3O4 particles and thus the rate of their production. At lower temperatures, the solute energy is weak and the crystal formation rate is slow. As the temperature increases, the rate of crystal formation gradually reaches its maximum. The continued increase in temperature will cause the kinetic energy of molecules in solution to increase too fast, which is not conducive to the formation of stable particles and reduces the rate of crystal formation [33].
4.2 Analysis of Response Surface Experimental Results
ANOVA was used to assess the adequacy of the second-order regression model developed by BBD, and the results were shown in Tables 2–4. The F value and P-value of the model (Prob > F) determined the model significance. The model F values for Cu2+, Zn2+, and Pb2+ were 48.90, 137.74, and 27.31, respectively. The P-values (Prob > F) for the Cu2+ and Zn2+ models were less than 0.001, indicating that the Cu2+ and Zn2+ models were highly significant. The P-value for the Pb2+ model was 0.001, which was less than 0.05, indicating that the model was significant. The P values (Prob > F) for the model items A, B, C, AB, AC in the Cu2+ model, A, B, C, A2, B2, C2 in the Zn2+ model, and A, B, AB, AC, BC, A2 in the Pb2+ model were less than 0.05, indicating that the above model terms were significant in each model [1,29]. The closer the R2 value is to 1, the better the fit between the calculated results and the observed results of the model. The R2 values of the three models in this experiment were 0.9670, 0.9944, 0.9723, all very close to 1. R2Adj were 0.9473, 0.9872, 0.9367, indicating that the models could explain 94.73%, 98.72%, 93.67% of the changes in response values, respectively, and the regression models could better predict the experimental results and optimize the process parameters [31]. The AP values of the models in this experiment were 24.562, 34.144, 18.622, all greater than 4, while the coefficients of variation CV were 0.63%, 0.52%, 1.06%, all less than 10%, indicating that the models are highly accurate and stable [34,35]. In summary, RSM can better simulate the removal patterns of magnetically modified lignite for Cu2+, Zn2+, and Pb2+, and is a meaningful model.
The removal rate of Cu2+ increases with increasing particle size, and the increased speed is faster when the total Fe concentration and the molar ratio of Fe2+ to Fe3+ are low. The removal rate of Cu2+ decreases with increasing total iron concentration and declines more rapidly at a larger particle sizes and a higher molar ratio of Fe2+ to Fe3+. The removal rate of Cu2+ increases with the decrease of the molar ratio of Fe2+ to Fe3+, and the change rate increases with the increase of total Fe concentration and particle size (Fig. 2). Combining the response surface plots, considering the response surface and ANOVA results, indicates that the AB and AC interactions are very significant and the BC is not.
Figure 2: Response surface plot of the interaction effect of different preparation conditions on the removal rate of Cu2+. (a) Particle size and total Fe concentration (b) Particle size and molar ratio of Fe2+ to Fe3+ (c) Total Fe concentration and molar ratio of Fe2+ to Fe3+
The Zn2+ removal rate increases with increasing particle size, and decreases and then increases with increasing total Fe concentration and decreasing molar ratio of Fe2+ to Fe3+ (Fig. 3). The curvature of the response surface plot of AB, AC, and BC interaction is larger and the interaction effect of the binary parameters is higher, and the variance results verify this conclusion.
Figure 3: Response surface plot of the interaction effect of different preparation conditions on the removal rate of Zn2+. (a) Particle size and total Fe concentration (b) Particle size and molar ratio of Fe2+ to Fe3+ (c) Total Fe concentration and molar ratio of Fe2+ to Fe3+
In a certain range, the Pb2+ removal rate increases with the increase of particle size. When the particle size is too large, the Pb2+ removal rate decreases and the decrease is most obvious at the molar ratio of Fe2+ to Fe3+ of 1:1. Pb2+ removal rate decreases with increasing total Fe concentration, and the decrease is more obvious when the molar ratio of Fe2+ to Fe3+ is higher. When the particle size is small and the total Fe concentration is low, the Pb2+ removal rate decreases with the molar ratio of Fe2+ to Fe3+, and vice versa (Fig. 4). Combined with the response surface diagram, contour diagram, and variance analysis results, the pairwise interaction of AB, AC and BC is highly significant.
Figure 4: Response surface plot of the interaction effect of different preparation conditions on the removal rate of Pb2+. (a) Particle size and total Fe concentration (b) Particle size and molar ratio of Fe2+ to Fe3+ (c) Total Fe concentration and molar ratio of Fe2+ to Fe3+
The response surface BBD experimental design and the analysis of the results showed that all three factors had an effect on the ability of the prepared Fe3O4-L to adsorb metal ions, and there was an interactive effect between the three factors. Using the optimization function of Design Expert, the optimal preparation conditions for the preparation of Fe3O4-L by chemical co-precipitation were optimized as follows: the particle size of 250 mesh, the total Fe concentration of 0.5 mol/L, and the molar ratio of Fe2+ to Fe3+ of 1:2. The removal rates of Cu2+, Zn2+, and Pb2+ in AMD by Fe3O4-L prepared under these conditions were 94.52%, 88.49% and 96.69%.
4.3 Analysis of Adsorption Behavior
The removal of all three ions by Fe3O4-L shows an increasing trend as the reaction progressed. In the first 60 min, the adsorption sites on the adsorbent surface are sufficient, the mass transfer driving force between the solution and the adsorbent surface is large, and the adsorption rate increases significantly. In the middle and late stages, the adsorption sites on the surface gradually reach adsorption saturation. The adsorption of metal ions is transferred from the surface to the adsorption sites in the internal pores and the adsorption rate is slowed down. The adsorption basically reaches the equilibrium state at 180 min. The removal rates of Fe3O4-L for Cu2+, Zn2+ and Pb2+ at equilibrium are 99.99%, 85.27% and 97.48%, respectively (Fig. 5a). Fe3O4-L is a porous adsorbent with a high adsorption rate loaded with Fe3O4 nanoparticles on the lignite matrix, which has a good prospect for the rapid removal of heavy metal cations from polluted wastewater [23,24].
Figure 5: The effect of reaction time on the adsorption of Cu2+, Zn2+, Pb2+ by Fe3O4-L and the fitting results of the kinetic model. (a) The effect of reaction time on the adsorption of Cu2+, Zn2+, Pb2+ by Fe3O4-L (b) Fitting results of quasi-first-order kinetic model (c) Fitting results of quasi-second-order kinetic model (d) Fitting results of intra-particle diffusion model
The solute adsorption rate controls the residence time of the adsorbate at the solid-solution interface, so understanding the adsorption kinetics is important for the study of pollutant removal. In order to study the adsorption mechanism and potential rate-controlling steps throughout the adsorption process, pseudo-first-order models (such as Eq. (2)), pseudo-second-order models (such as Eq. (3)), and intraparticle diffusion models (such as Eq. (4) were utilized) to fit and analyze the kinetic process of removing Cu2+, Zn2+, and Pb2+ from Fe3O4-L prepared by chemical co-precipitation method. The results are shown in Table 5 and Figs. 5b–5d.
where, qt and qe are the adsorption capacity at time t (min) and equilibrium, respectively (mg/g), K1 is the rate constant of quasi-first-order kinetic reaction (min−1), K2 is the rate constant of quasi-second-order kinetic reaction (mg/g⋅min), K3 is the reaction rate coefficient of intra-particle diffusion (mg/g⋅min0.5), and t is the adsorption time (min) [6,36,37].
From Table 5, Figs. 5b–5d, it can be seen that the R2 of Fe3O4-L adsorption process of Cu2+, Zn2+, Pb2+ fit pseudo-first-order, pseudo-second-order kinetics and intraparticle diffusion models are: 0.8155, 0.8316, 0.8249, 0.9981, 0.9939, 0.9947, 0.9046, 0.7195, 0.8904, 0.8878, 0.8778, 0.8974. The value of R2 can be judged that the pseudo-second-order kinetic equation can better describe the adsorption process of Fe3O4-L to three heavy metal ions. According to the mechanism established by the pseudo-second-order kinetic equation, it can be inferred that in the process of Fe3O4-L adsorption of three heavy metals, physical adsorption and chemical adsorption coexist, and chemical adsorption is the main one [38,39]. All the curves of Fe3O4-L adsorption of Cu2+, Zn2+, Pb2+ with time can be divided into two parts, and do not pass through the coordinate origin, which indicates that the adsorption process is determined by both surface adsorption and slow channel diffusion, but the effect of diffusion adsorption on chemisorption rate Impact can be ignored [36].
In each temperature regime, an increase in the initial concentration increases the concentration difference between the solution and the adsorbent surface, leading to an increase in the adsorption of Cu2+, Zn2+, and Pb2+ per unit mass of Fe3O4-L (Figs. 6a, 6c, 6e). However, Fe3O4-L has a limited adsorption capacity and cannot further reduce the concentration of the remaining metal ions in the solution after the adsorption reaches saturation. The adsorption capacity of Fe3O4-L for Cu2+, Zn2+ is larger and that for Pb2+ is smaller when the temperature increases, which proves that the adsorption reaction of Fe3O4-L for Cu2+ and Zn2+ is an absorbing reaction, and that for Pb2+ is an exothermic reaction. Under the same environmental conditions, the adsorption effect of Fe3O4-L on Cu2+ and Pb2+ was better than that of Zn2+. Differences in migration rates, ionic radii and hydration energy of Cu2+, Zn2+ and Pb2+ lead to higher adsorption affinity of Fe3O4-L for Cu2+ and Pb2+ than for Zn2+ [3].
Figure 6: Effect of initial concentration on adsorption of Cu2+, Zn2+, Pb2+ by Fe3O4-L at different temperature systems and isotherm model fitting. (a) Effect of initial concentration on adsorption of Cu2+ by Fe3O4-L at different temperature systems. (b) Isotherm fitting of Cu2+ adsorbed by Fe3O4-L. (c) Effect of initial concentration on adsorption of Zn2+ by Fe3O4-L at different temperature systems. (d) Isotherm fitting of Zn2+ adsorbed by Fe3O4-L. (e) Effect of initial concentration on adsorption of Pb2+ by Fe3O4-L at different temperature systems (f) Isotherm fitting of Pb2+ adsorbed by Fe3O4-L
Adsorption isotherms are often used to describe the interaction of an adsorbent with an adsorbate. The Langmuir and Freundlich adsorption model are important models for describing many adsorption isotherms. Using the Langmuir and Freundlich adsorption models, the adsorption forms of Fe3O4-L for Cu2+, Zn2+, and Pb2+ were explored and the maximum adsorption capacity was calculated [37].
The Langmuir temperature model describes monolayer adsorption: when an adsorbent chemisorbs on a fixed number of active centers on the surface of the adsorbent, a monolayer is formed, where each active center is large enough to accommodate one adsorbed cation. All active sites are equivalent, and there is no interaction between species adsorbed on adjacent active sites. Furthermore, adsorption energy and adsorption enthalpy are equivalent. Eq. (5) represents the nonlinear form of the Langmuir isotherm:
where, KL is the adsorption constant related to the adsorption activation energy, the larger the value, the stronger the adsorption capacity; Ce is the remaining adsorbate concentration in the solution at equilibrium (mg/L), and qe is the adsorption capacity at equilibrium (mg/g). Qm is the theoretical saturation capacity of complete monolayer adsorption (mg/g), which is also related to the maximum adsorption capacity.
The Freundlich isotherm describes adsorption occurring on amorphous surfaces. This isotherm involves reversible adsorption and is not limited to monolayer formation. The Freundlich isotherm assumes that adsorption occurs at multiple locations due to the inhomogeneity of the associated surface. Eq. (6) represents the nonlinear form of Freundlich isotherm:
where, KF is the Freundlich constant related to the adsorption equilibrium, n is the Freundlich constant related to the number of active centers in the adsorbent required for the adsorption of a metal cation, and Ce (mg/L) is the remaining adsorbate concentration in the solution at equilibrium, qe (mg/g) is the adsorption capacity at equilibrium [40].
Figs. 6b, 6d and 6f show the adsorption isotherms of Cu2+, Zn2+, Pb2+ three heavy metal ions adsorbed by Fe3O4-L at 25°C, 35°C and 45°C, respectively. The relevant parameters of data fitting are shown in Table 6. According to the fitting results, both Langmuir and Freundlich adsorption models can well fit the isotherm adsorption process of Fe3O4-L for three heavy metal ions. From the correlation coefficient R2, the Langmuir model can better fit the adsorption process. The Langmuir isotherm is related to monolayer adsorption and homogeneous adsorption, so the adsorption of Cu2+, Zn2+, Pb2+ three heavy metal ions by Fe3O4-L is more inclined to homogeneous monolayer adsorption [38]. According to the Langmuir model, the theoretical maximum adsorption capacity of Fe3O4-L for Cu2+, Zn2+, and Pb2+ at different temperatures can be calculated, which are 14.5273 (25°C), 14.7014 (35°C), 14.9039 (45°C), 11.3407 (25°C), 12.1250 (35°C), 12.6858 (45°C), 14.2771 (25°C), 13.3843 (35°C), 12.8604 (45°C), the theoretical values are similar to the experimental results [41–43].
The adsorption capacity of the adsorbent is usually represented by the maximum adsorption capacity calculated by the Langmuir model. Table 7 summarizes the ability of other adsorbents reported in the literature to adsorb Cu2+, Zn2+, and Pb2+. Obviously, Fe3O4-L is superior to Pellet material of iron tailings (IT) compounded with sodium alginate (SA) [44], GCM2 [45], KOH-RH [46], SiO2/PAA [47], Sawdust of Populus alba [48] and Magnetite nanospheres [49]. Although some adsorbents such as Nanoporous activated neem bark have a better adsorption effect than Fe3O4-L, these adsorbents have the problems of a complicated preparation process, high cost and difficulty in recycling [41–43].
The stability of magnetic materials is an important factor in their adsorption applications. The results of the cyclic adsorption experiment on Fe3O4-L are shown in Fig. 7. It can be seen from the figure that the first removal rates of Cu2+, Zn2+ and Pb2+ of Fe3O4-L are: 99.76%, 85.17% and 97.18%, respectively. After five cycles of adsorption-desorption experiments, the adsorption rates decreased by 6.92%, 5.84% and 4.79%, respectively. It shows that Fe3O4-L has a strong regeneration ability, and has the advantages of stable recycling when treating heavy metal pollution in water [50–52].
Figure 7: The experimental results of cyclic adsorption by Fe3O4-L
4.4 Characterization and Mechanistic Analysis
Lignite has a dense structure and uneven shape, with many microfractures and large pores distributed on the surface. The surface of Fe3O4-L is rougher and covered with a large number of tiny particles (Fig. 8). The elemental composition of lignite is dominated by C, O, Al, Si and Ca, while Fe3O4-L adds a large amount of Fe elements compared with lignite (Fig. 9). SEM and EDS results illustrate that the Fe3O4-L surface was successfully loaded with a large number of Fe3O4 particles and had a larger specific surface area and more pore structure than lignite, which was particularly favorable for the adsorption of heavy metal ions. A large number of precipitates accumulated on the surface and pores of Fe3O4-L with adsorbed metal ions, and Cu, Zn and Pb elements obviously appeared on Fe3O4-L after adsorption, indicating that Cu2+, Zn2+ and Pb2+ were successfully on Fe3O4-L and underwent precipitation reactions.
Figure 8: The SEM spectra of the materials. (a) Lignite (b) Fe3O4-L (c) Fe3O4-L after adsorption of Cu2+, Zn2+ and Pb2+
Figure 9: The EDS spectra of the materials. (a) Lignite (b) Fe3O4-L (c) Fe3O4-L after adsorption of Cu2+ (d) Fe3O4-L after adsorption of Zn2+ (e) Fe3O4-L after adsorption of Pb2+
The X-ray diffraction patterns (XRD) and Fourier transform infrared spectra (FTIR) of lignite and Fe3O4-L before and after adsorption are shown in Fig. 10. In the figure, a represents lignite, b represents Fe3O4-L, and c, d, and e represent Fe3O4-L after adsorption of Cu2+, Zn2+, and Pb2+, respectively.
Figure 10: The XRD patterns and FTIR spectras of the materials. (a) XRD patterns (b) FTIR spectras. a. Lignite b. Fe3O4-L c. Fe3O4-L after adsorption of Cu2+ d. Fe3O4-L after adsorption of Zn2+ e. Fe3O4-L after adsorption of Pb2+
Analysis of XRD results shows that lignite is mainly composed of conventional mineral components such as SiO2, and the Fe3O4 crystalline phase appears obviously after magnetic modification, which confirms the successful preparation of Fe3O4-L. After the adsorption reaction Cu2+, Zn2+ and Pb2+ existed as CuFe2O4, Zn(OH)2, ZnFe2O4 and PbS and PbS, respectively, as a result of the ion exchange and complexation reaction of Cu2+, Zn2+ and Pb2+ on the surface of Fe3O4-L. Cu2+, Zn2+ and Pb2+ are adsorbed onto the Fe3O4-L surface driven by the mass transfer forces generated by the concentration difference between the Fe3O4-L surface and the solution, and precipitation occurs to form Cu(OH)2, Zn(OH)2, PbS. Cu(OH)2, some of Zn(OH)2 and Fe(OH)3, the hydrolysis product of Fe3+, undergo a co-precipitation reaction to form CuFe2O4 and ZnFe2O4.
Analysis of the FTIR results shows that the typical absorption peak of Fe3O4 clearly appears near 582 cm−1 in b compared to a, which is attributed to the stretching vibration of the Fe-O bond, indicating a successful preparation. Before and after the adsorption of heavy metal ions by Fe3O4-L, the absorption peaks near 3400, 2922, 1690, 1593 cm−1 were shifted and the series of absorption peaks corresponding to complex C-H out-of-plane bending vibrations were changed in the range of 600–900 cm−1, which verified that the adsorption of Cu2+, Zn2+, and Pb2+ by Fe3O4-L was a combination of physical and chemical adsorption. Some collapse of the crystal structure of Fe3O4-L after adsorption of heavy metal ions occurs as a result of the reaction between metal ions and Fe-O [23,53,54].
(1) In the single-factor experiment for the preparation of Fe3O4-L by chemical co-precipitation, the particle size, the molar ratio of Fe2+ to Fe3+, total Fe concentration and water bath temperature were used as factors to analyze the effects of Fe3O4-L in removing Cu2+, Zn2+ and Pb2+ from AMD. It was determined that the Fe3O4-L prepared with a particle size of 250 mesh, a molar ratio of Fe2+ to Fe3+ of 1:2, a total Fe concentration of 0.7 mol/L, and a water bath temperature of 60°C was better. Among these reaction conditions, the particle size, the total Fe concentration, and the molar ratio of Fe2+ to Fe3+ were more influential.
(2) The response surface experiment was carried out to optimize the preparation of Fe3O4-L by taking the particle size, the Fe iron concentration and the molar ratio of Fe2+ to Fe3+ as factors. The results showed that the optimal reaction conditions for the preparation of Fe3O4-L by chemical co-precipitation were as follows: the particle size of 250 mesh, the total Fe concentration of 0.5 mol/L, and the molar ratio of Fe2+ to Fe3+ of 1:2. Under these conditions, the removal rates of Cu2+, Zn2+ and Pb2+ were 94.52%, 88.49% and 96.69%, respectively.
(3) Fe3O4-L was prepared as adsorbent under optimal preparation conditions, and static adsorption was performed at different reaction times and different initial concentrations under different temperature systems respectively. The results showed that the adsorption of Cu2+, Zn2+ and Pb2+ by Fe3O4-L basically reached equilibrium at 180 min, and the removal rates of Cu2+, Zn2+ and Pb2+ at equilibrium were 99.99%, 85.27% and 97.48%, respectively. At the same temperature regime, an increase in the initial concentration is beneficial to enhance the adsorption capacity and efficiency, but the removal rate decreases. The adsorption reactions of Fe3O4-L for Cu2+ and Zn2+ are heat-absorbing reactions, and those on Pb2+ are exothermic reactions. Fe3O4-L can still maintain a high adsorption capacity after five cycles of adsorption-desorption experiments.
(4) The lignite and Fe3O4-L before and after adsorption were characterized by SEM-EDS, XRD and FTIR. The appearance of a large number of fine particles on the surface of lignite, a significant increase in Fe content, the appearance of the Fe3O4 crystalline phase and Fe-O bonding proved the success of Fe3O4-L preparation. Cu2+, Zn2+ and Pb2+ were adsorbed by Fe3O4-L and mainly existed in the form of CuFe2O4, Zn(OH)2, ZnFe2O4 and PbS respectively, as a result of the combined effect of physical diffusion, ion exchange and surface complexation reactions.
Acknowledgement: The authors would like to thank all editors and anonymous reviewers for their comments and suggestions.
Funding Statement: This work was supported by the National Natural Science Foundation of China (41672247), Liaoning Province’s “Program for Promoting Liaoning Talents” (XLYC1807159), the Discipline Innovation Team of Liaoning Technical University (LNTU20TD-21) and the Liaoning Provincial Department of Education (LJKZ0324).
Conflicts of Interest: The authors declare that they have no conflicts of interest to report regarding the present study.
References
1. Mousavi, S. J., Parvini, M., Ghorbani, M. (2018). Adsorption of heavy metals (Cu2+ and Zn2+ ) on novel bifunctional ordered mesoporous silica: Optimization by response surface methodology. Journal of the Taiwan Institute of Chemical Engineers, 84, 123–141. DOI 10.1016/j.jtice.2018.01.010. [Google Scholar] [CrossRef]
2. Luptakova, A., Ubaldini, S., Macingova, E., Fornari, P., Giuliano, V. (2012). Application of physical–chemical and biological–chemical methods for heavy metals removal from acid mine drainage. Process Biochemistry, 47(11), 1633–1639. DOI 10.1016/j.procbio.2012.02.025. [Google Scholar] [CrossRef]
3. Lu, X., Wang, F., Li, X. Y., Shih, K., Zeng, E. Y. (2016). Adsorption and thermal stabilization of Pb2+ and Cu2+ by zeolite. Industrial & Engineering Chemistry Research, 55(32), 8767–8773, 1–27. DOI 10.1021/acs.iecr.6b00896. [Google Scholar] [CrossRef]
4. Rozumová, L., Životský, O., Seidlerová, J., Motyka, O., Šafarík, I. et al. (2016). Magnetically modified peanut husks as an effective sorbent of heavy metals. Journal of Environmental Chemical Engineering, 4(1), 549–555. DOI 10.1016/j.jece.2015.10.039. [Google Scholar] [CrossRef]
5. Yin, W., Zhao, C., Xu, J., Zhang, J. (2019). The Cd(II) adsorption capacities of activated carbons optimized by RSM: Preparation and adsorption optimization. Desalination and Water Treatment, 159, 377–389. DOI 10.5004/dwt.2019.24185. [Google Scholar] [CrossRef]
6. Karapinar, N., Donat, R. (2009). Adsorption behaviour of Cu2+ and Cd2+ onto natural bentonite. Desalination, 249(1), 123–129. DOI 10.1016/j.desal.2008.12.046. [Google Scholar] [CrossRef]
7. Olu-Owolabi, B. I., Unuabonah, E. I. (2011). Adsorption of Zn2+ and Cu2+ onto sulphate and phosphate-modified bentonite. Applied Clay Science, 51(1–2), 170–173. DOI 10.1016/j.clay.2010.10.022. [Google Scholar] [CrossRef]
8. Zhao, Y., Wang, Z., Zhao, G., Sun, R. (2019). Effects of upgrading treatment on physicochemical structure, moisture re-adsorption ability and NOx emission characteristic of lignite particles. Energy & Fuels, 33, 4070–4078. DOI 10.1021/acs.energyfuels.9b00306. [Google Scholar] [CrossRef]
9. Arslan, G., Pehlivan, E. (2008). Uptake of Cr3+ from aqueous solution by lignite-based humic acids. Bioresource Technology, 99(16), 7597–7605. DOI 10.1016/j.biortech.2008.02.007. [Google Scholar] [CrossRef]
10. Chu, H., Xue, Z., Gao, Y. (2021). Study on the adsorption behavior of mercury and arsenic by lignite. Multipurpose Utilization of Mineral Resources, 2, 91–96. DOI 10.3969/j.issn.1000-6532.2021.02.017. [Google Scholar] [CrossRef]
11. Di, J., Zhang, S., Yang, Y., Liang, B., Wang, X. et al. (2020). Study on adsorption characteristics of modified lignite for Fe2+, Mn2+ in acid mine drainage. Coal Science and Technology. https://kns.cnki.net/kcms/detail/11.2402.TD.20200731.1639.002.html. [Google Scholar]
12. Mohan, D., Chander, S. (2006). Removal and recovery of metal ions from acid mine drainage using lignite—A low cost sorbent. Journal of Hazardous Materials, 137(3), 1545–1553. DOI 10.1016/j.jhazmat.2006.04.053. [Google Scholar] [CrossRef]
13. Peiravi, M., Mote, S. R., Mohanty, M. K., Liu, J. (2017). Bioelectrochemical treatment of acid mine drainage (AMD) from an abandoned coal mine under aerobic condition. Journal of Hazardous Materials, 333, 329–338. DOI 10.1016/j.jhazmat.2017.03.045. [Google Scholar] [CrossRef]
14. Zhang, W., Zhang, B., Yuan, Z. (2012). Advances in the preparation of nanomagnetite by chemical coprecipitation. Journal of Inner Mongolia University of Science and Technology, 31(4), 348–350. DOI 10.16559/j.cnki.2095-2295.2012.04.015. [Google Scholar] [CrossRef]
15. Linh, P. H., Manh, D. H., Phong, P. T., Hong, L. V., Phuc, N. X. (2014). Magnetic properties of Fe3O4 nanoparticles synthesized by coprecipitation method. Journal of Superconductivity & Novel Magnetism, 27(9), 2111–2115. DOI 10.1007/s10948-014-2561-9. [Google Scholar] [CrossRef]
16. Wu, S., Sun, A., Zhai, F., Wang, J., Xu, W. et al. (2011). Fe3O4 magnetic nanoparticles synthesis from tailings by ultrasonic chemical co-precipitation. Materials Letters, 65(12), 1882–1884. DOI 10.1016/j.matlet.2011.03.065. [Google Scholar] [CrossRef]
17. Mahmud, N., Nasser, M. S., El-Naas, M. H., Ba-Abbad, M. M., Mohammad, A. W. et al. (2020). Synthesis and characterization of Fe3O4 nanoparticles using different experimental methods. IOP Conference Series: Materials Science and Engineering, 778, 012028. DOI 10.1088/1757-899X/778/1/012028. [Google Scholar] [CrossRef]
18. Safarik, I., Baldikova, E., Prochazkova, J., Safarikova, M., Pospiskova, K. (2018). Magnetically modified agricultural and food waste: Preparation and application. Journal of Agricultural & Food Chemistry, 66(11), 2538–2552. DOI 10.1021/acs.jafc.7b06105. [Google Scholar] [CrossRef]
19. Lim, Y. S., Lai, C. W., Hamid, S. B. A., Julkapli, N. M., Yehya, W. A. et al. (2014). A study on growth formation of nano-sized magnetite Fe3O4 via co-precipitation method. Materials Research Innovations, 18(sup6), S6–457–S6–461. DOI 10.1179/1432891714Z.0000000001028. [Google Scholar] [CrossRef]
20. Sebayang, P., Kurniawan, C., Aryanto, D., Setiadi, E. A., Tamba, K. et al. (2018). Preparation of Fe3O4/Bentonite nanocomposite from natural iron sand by co-precipitation method for adsorbents materials. IOP Conference Series: Materials Science and Engineering, 316, 012053. DOI 10.1088/1757-899X/316/1/012053. [Google Scholar] [CrossRef]
21. Hashemian, S., Saffari, H., Ragabion, S. (2014). Adsorption of cobalt(II) from aqueous solutions by Fe3O4/Bentonite nanocomposite. Water, Air, & Soil Pollution, 226(1), 2212.1–2212.10. DOI 10.1007/s11270-014-2212-6. [Google Scholar] [CrossRef]
22. Vikram, S., Dhakshnamoorthy, M., Vasanthakumari, R., Rajamani, A. R., Rangarajan, M. et al. (2015). Tuning the magnetic properties of iron oxide nanoparticles by a room-temperature air-atmosphere (RTAA) co-precipitation method. Journal of Nanoscience & Nanotechnology, 15(5), 3870–3878. DOI 10.1166/jnn.2015.9544. [Google Scholar] [CrossRef]
23. Petcharoen, K., Sirivat, A. (2012). Synthesis and characterization of magnetite nanoparticles via the chemical co-precipitation method. Materials Science & Engineering B, 177(5), 421–427. DOI 10.1016/j.mseb.2012.01.003. [Google Scholar] [CrossRef]
24. Meng, H., Zhang, Z., Zhao, F., Qiu, T., Yang, J. (2013). Orthogonal optimization design for preparation of Fe3O4 nanoparticles via chemical coprecipitation. Applied Surface Science, 280, 679–685. DOI 10.1016/j.apsusc.2013.05.041. [Google Scholar] [CrossRef]
25. Dehghanpour, H. R. (2020). pH, molar ratio of ferrous to ferric ions and surfactant presence effects on physical properties of iron oxide nanoparticles generated by co-precipitation method. Journal of Coordination Chemistry, 73(24), 3452–3464. DOI 10.1080/00958972.2020.1849639. [Google Scholar] [CrossRef]
26. Zhang, S., Zhang, Z., Wen, S. (2011). Nanometer ferric oxide powder was prepared by chemical coprecipitation method. Liaoning Chemical Industry, 40(4), 325–327 + 351. DOI 10.3969/j.issn.1004-0935.2011.04.001. [Google Scholar] [CrossRef]
27. Wang, Z., Fu, P., Wang, R., Lui, P., Yu, Y. (2022). Study on the adsorption of Cr (VI) in water by lignite residue after humic acid extraction. Industrial Water Treatment, 43(1), DOI 10.19965/j.cnki.iwt.2022-0221. [Google Scholar] [CrossRef]
28. Chou, P. I., Ng, D. Q., Li, I. C., Lin, Y. P. (2018). Effects of dissolved oxygen, pH, salinity and humic acid on the release of metal ions from PbS, CuS and ZnS during a simulated storm event. Science of the Total Environment, 624, 1401–1410. DOI 10.1016/j.scitotenv.2017.12.221. [Google Scholar] [CrossRef]
29. Kumar, S. S., Bishnoi, N. R. (2017). Coagulation of landfill leachate by FeCl3: Process optimization using Box–Behnken design (RSM). Applied Water Science, 7(4), 1943–1953. DOI 10.1007/s13201-015-0372-1. [Google Scholar] [CrossRef]
30. Su, C., Li, W., Liu, X., Li, X. (2013). Optimal preparation conditions of sepiolite-supported nanoscale iron using response surface methodology. Acta Scientiae Circumstantiae, 33(4), 985–990. DOI 10.13671/j.hjkxxb.2013.04.025. [Google Scholar] [CrossRef]
31. An, S., Li, H. (2021). Optimization of NaHSO3 enhanced Fe2+/persulfate system for treatment of eriochrome black T wastewater by response surface methods. Industrial Water Treatment, 41(7), 112–116. DOI 10.19965/j.cnki.iwt.2020-0937. [Google Scholar] [CrossRef]
32. Huang, J., Xu, Z., Yi, C. (2007). Fe3O4 nanoparticles prepared by chemical co-precipitation method. Journal of Hubei University (Natural Science), 29(1), 50–52. [Google Scholar]
33. Zhang, S., Zhang, Z., Wen, S. (2011). Nanotriferroteoxide powder was prepared by chemical co-precipitation. Liaoning Chemical Industry, 40(4), 325–327. [Google Scholar]
34. Bagheri, S., Aghaei, H., Ghaedi, M., Asfaram, A., Monajemi, M. et al. (2017). Synthesis of nanocomposites of iron oxide/gold (Fe3O4/Au) loaded on activated carbon and their application in water treatment by using sonochemistry: Optimization study. Ultrasonics Sonochemistry, 41, 279–287. DOI 10.1016/j.ultsonch.2017.09.031. [Google Scholar] [CrossRef]
35. Luo, L., Wang, G., Wang, Z., Ma, J., He, Y. et al. (2021). Optimization of fenton process on removing antibiotic resistance genes from excess sludge by single-factor experiment and response surface methodology. Science of the Total Environment, 788, 147889. DOI 10.1016/J.SCITOTENV.2021.147889. [Google Scholar] [CrossRef]
36. Huang, X., Yang, J., Wang, J., Bi, J., Xie, C. et al. (2018). Design and synthesis of core–shell Fe3O4 @PTMT composite magnetic microspheres for adsorption of heavy metals from high salinity wastewater. Chemosphere, 206, 513–521. DOI 10.1016/j.chemosphere.2018.04.184. [Google Scholar] [CrossRef]
37. Wang, S., Yu, Y., He, L., Zhang, D., Ye, M. (2019). Design of magnetic nanoparticles with high magnetic separation efficiencies and durability for Cu2+ adsorption. Nanotechnology, 31(8), 085710. DOI 10.1088/1361-6528/ab55c2. [Google Scholar] [CrossRef]
38. Zhang, Z., Kuang, Q., Jia, X. (2010). Study on the kinetics and thermodynamics of Pb2+, Cu2+, Cr3+, Cd2+, Ni2+ adsorption onto peanut hull. Ecology and Environmental Sciences, 19(12), 2973–2977. DOI 10.16258/j.cnki.1674-5906.2010.12.018. [Google Scholar] [CrossRef]
39. Jia, N., Wang, H., Huo, J. (2006). The study on adsorption of Zn2+ by modified meerschaum. China Mining Magazine, 15(4), 70–72. [Google Scholar]
40. Paulino, A. T., Belfiore, L. A., Kubota, L. T., Muniz, E. C., Almeida, V. C. et al. (2011). Effect of magnetite on the adsorption behavior of Pb(IICd(IIand Cu(II) in chitosan-based hydrogels. Desalination, 275(1–3), 187–196. DOI 10.1016/j.desal.2011.02.056. [Google Scholar] [CrossRef]
41. Li, Q., Yu, J., Zhou, F., Jiang, X. (2015). Synthesis and characterization of dithiocarbamate carbon nanotubes for the removal of heavy metal ions from aqueous solutions. Colloids and Surfaces A: Physicochemical and Engineering Aspects, 482, 306–314. DOI 10.1016/j.colsurfa.2015.06.034. [Google Scholar] [CrossRef]
42. Olegario, E., Pelicano, C. M., Felizco, J. C., Mendoza, H. (2019). Thermal stability and heavy metal (As5+, Cu2+, Ni2+, Pb2+ and Zn2+) ions uptake of the natural zeolites from the Philippines. Materials Research Express, 6(8), 085204. DOI 10.1088/2053-1591/ab1a73. [Google Scholar] [CrossRef]
43. Garg, R., Garg, R., Khan, M. A., Bansal, M., Garg, V. K. (2022). Utilization of biosynthesized silica-supported iron oxide nanocomposites for the adsorptive removal of heavy metal ions from aqueous solutions. Environmental Science and Pollution Research. DOI 10.1007/s11356-022-21111-2. [Google Scholar] [CrossRef]
44. Xu, C., Feng, Y., Li, H., Wu, R., Ju, J. et al. (2022). Adsorption of heavy metal ions by iron tailings: Behavior, mechanism, evaluation and new perspectives. Journal of Cleaner Production, 344, 131065. DOI 10.1016/j.jclepro.2022.131065. [Google Scholar] [CrossRef]
45. Seyedein Ghannad, S. M. R., Lotfollahi, M. N. (2018). Preparation of granular composite materials as novel sorbents and their application for removal of heavy metals from solution. International Journal of Environmental Science and Technology, 16, 3697–3706. DOI 10.1007/s13762-018-1772-1. [Google Scholar] [CrossRef]
46. Hossain, N., Nizamuddin, S., Shah, K. (2022). Thermal-chemical modified rice husk-based porous adsorbents for Cu(IIPb(IIZn(IIMn(II) and Fe(III) adsorption. Journal of Water Process Engineering, 46, 102620. DOI 10.1016/j.jwpe.2022.102620. [Google Scholar] [CrossRef]
47. Qu, J., Liao, Z., Zong, M., Zhang, F., Zhang, H. et al. (2021). Preparation of SiO2/PAA and its adsorption behavior to Cu2+ and Pb2+. Industrial Water & Wastewater, 52(5), 42–46. [Google Scholar]
48. Najam, R., Andrabi, S. M. A. (2016). Adsorption capability of sawdust of populus alba for Pb(IIZn(II) and Cd(II) ions from aqueous solution. Desalination and Water Treatment, 57(59), 29019–29035. DOI 10.1080/19443994.2016.1157039. [Google Scholar] [CrossRef]
49. Kumari, M., Pittman, C. U., Mohan, D. (2015). Heavy metals [chromium (VI) and lead (II)] removal from water using mesoporous magnetite (Fe3O4) nanospheres. Journal of Colloid and Interface Science, 442, 120–132. DOI 10.1016/j.jcis.2014.09.012. [Google Scholar] [CrossRef]
50. Rajput, S., Pittman, C. U., Mohan, D. (2016). Magnetic magnetite (Fe3O4) nanoparticle synthesis and applications for lead (Pb2+) and chromium (Cr6+) removal from water. Journal of Colloid and Interface Science, 468, 334–346. DOI 10.1016/j.jcis.2015.12.008. [Google Scholar] [CrossRef]
51. Chao, L., Wang, Y., Chen, S., Li, Y. (2021). Preparation and adsorption properties of chitosan-modified magnetic nanoparticles for removal of Mo (VI) ions. Polish Journal of Environmental Studies, 30(3), 2489–2498. DOI 10.15244/pjoes/130039. [Google Scholar] [CrossRef]
52. Zhu, C., Dong, X., Chen, Z., Naidu, R. (2016). Adsorption of aqueous Pb(IICu(IIZn(II) ions by amorphous tin(VI) hydrogen phosphate: An excellent inorganic adsorbent. International Journal of Environmental Science and Technology, 13(5), 1257–1268. DOI 10.1007/s13762-016-0964-9. [Google Scholar] [CrossRef]
53. Ren, Y., Abbood, H. A., He, F., Peng, H., Huang, K. (2013). Magnetic EDTA-modified chitosan/SiO2/Fe3O4 adsorbent: Preparation, characterization, and application in heavy metal adsorption. Chemical Engineering Journal, 226, 300–311. DOI 10.1016/j.cej.2013.04.059. [Google Scholar] [CrossRef]
54. Di, J., Li, M., Wang, X., Yang, Y., Liang, B. et al. (2020). Experimental study on adsorption of Cu2+ and Zn2+ in acid mine drainage by modified lignite. Coal Science and Technology, 50(3), 301–307. [Google Scholar]
Cite This Article
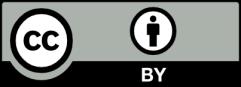
This work is licensed under a Creative Commons Attribution 4.0 International License , which permits unrestricted use, distribution, and reproduction in any medium, provided the original work is properly cited.