Open Access
REVIEW
Recent Advances in Polymer-Based Photocatalysts for Environmental Remediation and Energy Conversion: A Review
1 School of Physics & Materials Studies, Faculty of Applied Sciences, Universiti Teknologi MARA (UiTM), Shah Alam, 40450, Malaysia
2 Smart Manufacturing Research Institute & School of Mechanical Engineering, Universiti Teknologi MARA (UiTM), Shah Alam, 40450, Malaysia
3 Department of Materials & Metallurgical Engineering, University of Ilorin, Ilorin, 240102, Nigeria
4 Department of Geosciences, Universiti Teknologi PETRONAS, Bandar Seri Iskandar, 32610, Malaysia
5 Department of Pure and Applied Physics, Ladoke Akintola University of Technology, Ogbomoso, 210214, Nigeria
* Corresponding Author: Surajudeen Sikiru. Email:
Journal of Polymer Materials 2025, 42(1), 1-31. https://doi.org/10.32604/jpm.2025.058936
Received 24 September 2024; Accepted 10 December 2024; Issue published 27 March 2025
Abstract
Photocatalysis is a crucial technique for environmental cleanup and renewable energy generation. Polymer-based photocatalysts have attracted interest due to their adaptability, adjustable chemical characteristics, and enhanced light absorption efficiency. Unlike traditional inorganic photocatalysts, we can optimize polymeric systems to enhance photocatalytic efficiency and yield significant advantages in environmental remediation and energy conversion applications. This study talks about the latest developments in polymer-based photocatalysts and how important they are for cleaning water, breaking down pollutants, and making renewable energy through processes like hydrogen production and CO2 reduction. These materials are proficient in degrading harmful pollutants such as organic colours, insecticides, and medications, transforming them into innocuous byproducts. Moreover, its use in solar-driven water splitting for hydrogen production and CO2 reduction provides a sustainable solution to global energy and environmental issues. These photocatalysts are much more effective and last longer thanks to progress in polymer chemistry, nano-structuring, and hybridization with materials like semiconductors and metal nanoparticles. The research underscores the promise of polymer photocatalysts for extensive environmental applications due to their cost-efficiency, ease of separation, and reusability. Future research endeavors seek to optimize polymeric photocatalyst systems for improved stability and performance, hence advancing sustainable solutions for critical environmental and energy challenges.Keywords
Photocatalysis is critical in the advancement of innovative environmental and energy conversion and storage technologies, such as water treatment and the synthesis of solar fuels via artificial photosynthesis, specifically hydrogen generation and carbon dioxide reduction [1]. Recently, the advancement of polymer-based photocatalysts has attracted considerable interest due to their potentials in environmental remediation and energy conversion [2]. Photocatalysis is facilitated by light absorption to produce reactive species, provides an eco-friendly and sustainable remedy for persistent challenges like industrial waste pollution and energy deficiency [3]. Polymer-based photocatalysts have become a versatile group of materials because their chemical structures can be easily altered and are better light receptors [4]. In contrast to traditional inorganic photocatalysts, the design of polymers with customized functionalities enhances the efficiency of photocatalytic processes, rendering them exceptionally appropriate for many applications. Researchers have examined polymer-based photocatalysts in environmental remediation for their capacity to degrade harmful pollutants, including organic dyes, heavy metals, and other toxic chemicals present in water and air [5,6]. These materials may effectively produce reactive oxygen species when exposed to visible light, decomposing contaminants into less hazardous byproducts. In the realm of energy conversion, polymer-based photocatalysts show promise in applications including water splitting for hydrogen production and CO2 reduction, aiding in the development of clean and renewable energy [3]. Recent progress in polymer chemistry, nano-structuring, and hybridization with materials such as semiconductors and metal nanoparticles has made these photocatalysts much more effective [7].
Over the years, particles have been segregated from several wastewaters, which pass through membrane filtration and produce concentrated sludge. Optimized ribbon-based materials for effective contaminant removal, comparing them to traditional purification techniques, However, the process is usually unsuitable for dye removal and other industrial wastewater. Nano filtration and ultrafiltration have tried to improve among other inefficiencies with this process. Nevertheless, the short life span, the requirement of high pressure, costly and high energy consumption persist [8,9]. Wastewater such as dye effluents and pharmaceutical waste is treated with oxidizing agents to break down complex molecules into CO2 and H2O in short reaction time. However, it is pH dependent, requires catalyst for efficient removal and expensive [10,11]. Furthermore, the photochemical reaction and UV radiation for waster waste remediation ensure no sludge and foul odours production, but the it has a limited treatment time, costly, energy depletion [12]. Recent advancements in oxidation processes have been used to eliminate organic contaminants from wastewater, integrating them into various sectors like wastewater treatment, pharmaceuticals, textiles, and the circular economy. However, Ribbon-based water purification methods is more greener micro extraction [13,14], but still face significant challenges in scalability and technological limitations. These methods, while promising, struggle to transition from laboratory settings to practical applications due to various factors. Firstly, Plasma-based purification methods encounter difficulties in scaling up from bench-top demonstrations to larger systems, primarily due to the need for precise plasma dose delivery and the complexity of design considerations [15]. Especially heterogeneous photo catalysis, that has long treatment times due to its thermodynamic and kinetic limitations resulting in significantly longer than conventional methods hindering scalability [16,17]. Materials used in purification processes often generate high oxidative species quickly, which is crucial for efficient water treatment [18]. Advanced purification systems require complex operational setups, making them difficult to adapt for large-scale use. Despite these challenges, ongoing research could lead to breakthroughs that improve the scalability, reusability and efficiency of ribbon-based water purification methods [19]. Furthermore, multistep strategy for the synthesis of Polymer-based photo catalysis with ribbon networks and bimetallic phosphide nanoarrays which exhibited outstanding long-term stability in hydrogen energy is achievable [20]. Blueprint for future development and integration of ribbon-based technology into commercial or humanitarian water purification systems still needs to be explored. The increase in need for green energy and environmental degradation has led to the development of clean and green photo catalysis technology. Photocatalysis was first discovered by Honda and Fujishima in the photo-electrochemical water-splitting process using titanium dioxide (TiO2) as the photocatalyst. it has been used in various areas such as environmental cleanup, pollution reduction, energy production and chemical production [21]. Researchers have focused on the optimization of the physicochemical properties of photocatalysts to enhance efficiency through multiple improvement techniques [22]. Scientists are enhancing photo catalysis by engineering semiconductor interfaces within composite systems, including heterojunctions, p-n junctions, and Z-scheme systems. Solar energy can be utilized to remove organic compounds, produce sustainable and clean energy via water splitting, degrade pollutants, convert and store energy, and facilitate a sustainable method for the green synthesis of essential chemicals (Fig. 1) [2].
Figure 1: (A) single components, (B) two components (C) heterojunction with two semiconductors [2]
Environmental scientists are tasked with alleviating health problems caused by air, water, and soil pollution through effective techniques, particularly through green technologies. Research efforts aimed at eliminating or managing harmful contaminants, both organic and inorganic, mostly originating from industrial and human activities, are of paramount significance [23,24]. Water contaminants mainly enter the Earth’s ecology via industrial sources, such as textiles, tanneries, pharmaceuticals, and agriculture. Waste disposal from pharmaceutical enterprises and hospitals can pollute soil and water through complex interactions with other contaminants, posing a significant hazard to biological organisms. Air pollutants include gaseous substances such as particulate matter, carbon monoxide, carbon dioxide, and volatile organic compounds (VOCs) emitted by vehicles, industrial facilities, and pesticide applications [25]. These main pollutants may also produce secondary pollutants, exacerbating their detrimental impacts. Traditional methods have reduced environmental contaminants and toxic pollutants, but new and better ways to protect the environment are needed. This study offers a thorough examination of advancements in polymer-based photocatalysts, emphasizing their design methodologies, operational mechanisms, and novel applications in environmental and energy sectors. This study examines the problem and future approaches for enhancing the efficiency and stability of polymer-based photocatalytic systems, highlighting the potential of these materials to tackle significant environmental and energy issues.
First, the low effectiveness of many current polymer photocatalysts is caused by quick recombination of photogenerated electron-hole pairs, weak mobility of charge carriers, and restricted light absorption. Second, their long-term efficacy is diminished due to their instability under challenging environmental circumstances. Third, although a significant amount of research has been done on individual polymer-based photocatalysts, not enough attention has been paid to creating materials that can effectively carry out several tasks at once, such energy conversion and pollutant degradation, inside a single system. The development of multifunctional polymer-based photocatalysts that can aid in both energy production (such as hydrogen generation from water splitting) and environmental remediation (such as pollutant degradation) will be made possible by the integration of environmental and energy applications. This will have two benefits for sustainable development. By concentrating on these goals, our work aims to close important knowledge gaps regarding polymer-based photocatalysts and provide viable answers for expanding their use in energy generation and environmental remediation.
1.1 Importance of Polymer-Based Photocatalysts on Materials Science Innovation
Materials Science is an interdisciplinary field that investigates the composition, structure, and practical applications of various materials. It includes both classic and cutting-edge materials, such as metals, ceramics, polymers, composites, semiconductors, and biological materials. Scientists and engineers can control and build materials with specialized qualities for different applications by altering their structure at the atomic and molecular levels. Developing practical solutions for global issues in energy, sustainability, and healthcare is fundamental to research, economic growth, and societal progress [26,27]. Energy scarcity and environmental degradation often pose significant threats to human civilization, prompting a critical need for sustainable solutions. Traditional industrial practices are characterized by high energy consumption and pollution, combined with the growing demand for synthetic chemicals exacerbating these challenges. Addressing these issues requires innovative energy sources and advanced manufacturing processes. Photocatalysis offers a viable method for sustainably transforming energy derived from solar into chemical energy by using both light and catalyst [28,29]. A catalyst remains unchanged and is not consumed throughout the overall chemical process. The photochemical alteration occurs in one molecular entity because of initial absorption of radiation by another molecular entity which is photosensitized [30]. Producing clean hydrogen fuel through photocatalytic water splitting offers a viable remedy for the world’s energy dilemma, providing a clean, renewable, and environmentally friendly energy source. Photocatalytic technology converts CO2 into valuable compounds like CO, methane, and methanol, addressing the energy crisis and mitigating environmental issues caused by excessive CO2 emissions [31,32]. The majority of existing photocatalysts are constructed from transition metal-based semiconductors. These materials can be categorized as sulfides (such as NiS and CdS), oxides (like Ni (OH)2 and NiO), nitrides (like Ta3N5), or oxynitrides (like BaNbO2N). However, these materials face several challenges, such as large energy gaps and conduction band positions that impede proton reduction, toxicity, instability, challenges in modifying and adjusting inorganic photocatalysts, and high production costs [33].
Photocatalysis’s widespread application in industry and everyday life relies on the development of photocatalysts that are metal and non-metal and are highly active, affordable, selective, coherent across a wide range of solar wavelengths, and stable under various conditions [34]. This has made photo catalysis significantly benefit from advancements in materials science such as polymers. Polymeric materials (Fig. 2) such as natural polymers, Covalent Organic Frameworks, Graphitic Carbon Nitride, synthetic polymers, natural polymers, and conjugated polymers which are examples of innovation in material science have recently attracted a lot of interest because of their capacity to address the limitations of photocatalysts, such poor light, adsorption, and rapid charge carrier recombination [31,35–37]. These elements affect how well photocatalysts work in various applications, such as nitrogen fixation, organic synthesis, pollutant degradation, hydrogen generation, and CO2 reduction. Under these circumstances, polymer-based photocatalysts’ stability and reusability are essential for their widespread use [2,35].
Figure 2: An illustration showcasing polymer-based materials with photocatalytic properties as a new class for promising photocatalytic applications
Polymer-based photocatalysts absorb sunlight and convert energy into chemical reactions, enabling processes like pollutant breakdown and water splitting. That offers the advantages of homogeneous and heterogeneous photo catalysis, versatility, and affordability, making it less harmful than inorganic semiconductors [2,38]. While possessing a molecular structure, polymeric photocatalysts exhibit the robustness, stability, recoverability and scalability of inorganic semiconductors, unlike molecular homogeneous photocatalysts, which are highly customizable but often less durable [30,39]. Polymer-based photocatalysts have become a significant area of research and innovation in materials science due to their potential to address critical environmental and energy challenges. The maps in Fig. 3 highlight the global distribution of research activity in the field of polymer-based photocatalysts, showing that China leads in this area, followed by the United States and other countries. China dominates in the number of publications in Asia, followed by other Asian countries like India and Iran. The United States and Canada show significant contributions in North America. In Europe and Africa, Countries like Russia and Nigeria are mentioned but with fewer publications. While in Oceania, Australia is prominently contributing with 69 publications.
Figure 3: Country-wise distribution of polymer-based photocatalysts published in Materials Science Innovation
The chart in Fig. 4 demonstrates that polymer-based photo catalysis has evolved from a niche topic to a major research area, especially in the last decade. Research publications on the variety of polymeric-based materials as photocatalysts are becoming more and more common because of the tremendous attention these materials have received in the photocatalytic process.
Figure 4: Polymer-based photo catalysis articles published annually
1.2 Synthesis Methods of Polymer-Based Photocatalysts
The major processes used to create polymer-based photocatalysts include chemisorption, solution evaporation, oxidative polymerization, and in-situ deposition. The chosen method and synthesis steps significantly influence the final properties of the catalyst. The size, shape and morphology of nanocomposites can vary depending on the precursors used or the synthesis method used which might impact the nanocomposites photocatalytic activity. For instance, under UV and visible light, N-doped TiO2, which was produced from TiCl4 and TiOSO4 using NH3 and NH4Cl as nitrogen sources, exhibited distinct photocatalytic activity. Samples made from TiCl4 were more active when exposed to UV light, but samples made from TiOSO4 and calcined at 400°C were more active when exposed to visible light [40–42]. Synthetic materials derived from petroleum oil are created by linking together individual monomers to form long chains of basic hydrocarbons like ethylene and propylene. These synthetic polymers have become popular support for photocatalyst materials, leading to more efficient and durable photocatalyst composites. Incorporating synthetic polymers into photocatalysts can improve their ease of cleaning and recovery, extending their overall photocatalytic lifespan [35].
Polymer-based photocatalysts combine the properties of polymers with photocatalytic materials to create versatile catalysts for uses like solar energy conversion, chemical synthesis, and environmental cleanup. There are several methods to synthesize polymer-based photocatalysts listed in Table 1.
1.3 Enhancing Photocatalytic Efficiency through Hybrid Composites
The combination of functional polymers with inorganic nanostructures has been highlighted as a promising area for environmental remediation. These hybrid materials exhibit multifunctional properties that enhance their catalytic performance. The design and synthesis of this composite involves precise methodologies that allow for the alteration of their structure and composition, leading to improved catalytic activity for pollutant degradation and renewable energy. Ranging from plasma and vacuum ultra-violet radiation treatments, photochemical and polymer coating approaches, and formation of self-assembled monolayers on surfaces using designer molecules, which provides a durable and recyclable photocatalytic surface [57–59]. Photoactivatable reagents to form covalent bonds with polymer surfaces allow for the addition of functional groups that can enhance properties like wettability and hydrophobicity. Polyaniline-based Composites, particularly those combined with metal oxides and non-metallic semiconductors, have shown improved photocatalytic performance. The morphology and specific surface area of these materials are crucial factors influencing their efficiency. Ternary composites, in particular, have demonstrated superior performance compared to binary systems, making them effective in degrading dyes and phenolic compounds [60,61]. Another approach that offers a robust and reversible way to adapt surface properties for various high-end applications in nanoscale materials is covalent modification. This method allows for precise tuning of interface structure and properties, essential for optimizing the performance of graphene-like materials [62].
Graphene’s exceptional chemical and physical properties make it an ideal component in hybrid polymer nanocomposites. These materials have been used in biosensors for environmental remediation, showcasing their potential for photocatalytic degradation of organic pollutants. The ability to chemically modify these composites further enhances their reactivity and biocompatibility [63]. The unique physicochemical characteristics of graphene oxide membranes have made them very interesting candidates for water purification applications. Nevertheless, the compromise between permeability and selectivity, together with their susceptibility to membrane fouling, presents substantial obstacles to their extensive commercial implementation. A novel in-situ growth and layer-by-layer assembly method for producing multilayer graphene oxide membranes reinforced with bismuth oxybromide (BiOBr) on nylon substrates, enabling photocatalytic self-cleaning systems can be adjusted in nanochannel size [64].
Advancements in materials science and nanotechnology improve ribbon-based water purification by optimizing nanoribbon configurations, enhancing adsorption capabilities, and increasing photocatalytic degradation efficiency for contaminants. The construction of various nanoporous graphene quantum dots from nanoribbon super-lattices with tunable pore size and abundant active sites. The investigations were performed with density functional theory calculations that depends on the type of super-lattice, the pore size can be tuned from 3 to 11 Å and the pore morphologies are also controllable to decrease the band gap and act as active sites [65]. Also, multi-walled carbon nanotubes (MWCNTs) was used with different unzipping level complex chemical interactions between uranium (VI) and graphene oxide nanoribbons for uranium capture [66]. The excellent performance of fibrous MXene nanoribbons (MNRs) membrane with efficient water purification methods which allow water to pass through freely but block oil droplets. In addition, the mixed cellulose-MNRs membrane possessed the photocatalytic self-cleaning ability which can efficiently raise the flux recovery rate (FRR) by ultraviolet light irradiation [67].
Furthermore, the generation of high-energy compounds by solar-to-chemical energy conversion is a very feasible approach in the pursuit of sustainable energy resources. The incorporation of metal dopants into TiO2 can reduce the bandgap, improving light absorption and photocatalytic activity [68,69]. This process allows for uniform dispersion and strong interactions between the nanoparticles and the support [70]. Despite being traditionally controlled by inorganic semiconductors, organic polymeric photocatalysts have the benefit of a wide range of selectable optoelectronic and surface catalytic characteristics at the molecular level, attributable to their very precise molecular structure. The amorphous exsolution of metal nanoparticles from oxide supports can create stable interfaces that enhance catalytic activity. The creation of a substrate-coating system based on zinc and epoxy resin incorporated into modified graphene oxide, which possesses two key characteristics: effective resistance against corrosion and the ability to harness photocatalytic properties. The impact of photocatalytic activity in anticorrosion performance cannot be overemphasized in the environmental remediation effort ranging from modified graphene oxide deposited on a zinc substrate [71], molybdenum zinc oxide modified by carbon quantum dots pigments [72], electrospun fibers doped with metallic oxides [73], and enhanced single-walled carbon nanotube (SWCNT) dispersion within polymeric resins that improve surface mechanical characteristics for flexible composite system [74,75], Although further research is needed to determine the optimal surface polarity for bonding and other factors critical for enhancing photocatalytic efficiency to achieve consistent performance across different environmental conditions and composite formulations.
Photocatalytic reactions
Photocatalysis involves chemical reactions with light and a semiconductor substrate. Photocatalysts absorb light and act as catalysts, generating electron-hole pairs upon exposure to light. These reactions can be categorized into homogeneous photo catalysis, where both semiconductors and reactants are in the same phase, and heterogeneous photo catalysis, where both semiconductors and reactants are in different phases. The efficient separation of photo-generated electron-hole pairs is essential. However, rapid recombination of these charge carriers can reduce the efficiency of the system. This affects both the activity and durability of the photocatalyst because the energy intended for the reaction is lost as heat. The need for polymer-based photocatalyst which are heterogeneous catalysis have ability to transfer organic pollutants into carbon dioxide and other non-hazardous products in water without photoreduction [76,77]. The hybrid of various metal oxides and carbonaceous nanomaterials like GO, rGO and CNTs can reduce the recombination of photogenerated electrons and holes by promoting electrons towards the high energy level [70,78–81].
Recent advancements in heterogeneous photo catalysis techniques have significantly enhanced their potential applications in environmental remediation, particularly in addressing water and air pollution. Also, providing high surface areas and enabling easy recovery and reuse of catalysts [82]. These innovations include the development of defect-engineered metal oxides, novel semiconductor systems, and the integration of piezo-catalysis with photo catalysis. Defects in metal oxides like ZnO and TiO2 enhance photocatalytic activity, enhancing degradation rates of pollutants like organic dyes and pharmaceuticals. This defect-rich materials, including binary and perovskite oxides, are effective for environmental remediation due to their superior physicochemical properties [83,84]. Furthermore, the advanced semiconductor systems through heterojunction design systems have facilitate electron migration and enhance photocatalytic efficiency. Techniques such as S-scheme and p-n heterojunctions have been particularly effective in improving pollutant degradation mechanisms [85–87]. Other synergistic approaches are evolving with the combination of piezo-catalysis and photo catalysis has emerged as a promising strategy, where mechanical stress enhances charge carrier separation, leading to more efficient pollutant degradation [88]. While these advancements present exciting opportunities for environmental remediation, challenges such as scalability and the need for further optimization of photocatalytic materials remain critical for practical applications. This multifaceted approach will leverage the strengths of different materials and techniques to optimize photocatalytic processes such as electric and magnetic field modulation, improved spectral absorption and rapid carrier mobility through composite development and up-conversion photonic that allows for the harvesting of near-infrared solar energy. Therefore, electron utilization efficiency in photocatalytic hydrogen evolution is crucial for solar energy conversion and storage, with prolonged lifetime and effective electron use regulating efficiency [89].
In photo catalysis, efficient separation of photo-generated electron-hole pairs is essential. However, rapid recombination of these charge carriers can reduce the efficiency of the system. This affects both the activity and durability of the photocatalyst because the energy intended for the reaction is lost as heat. Creating a heterojunction between two semiconductors with different bandgaps can promote charge separation and reduce recombination. For example, coupling TiO2 with graphene or g-C3N4 enhances charge carrier separation. The application of more resilient long-term light exposure, such as carbon nitride (g-C3N4) or modified metal oxides, can enhance the stability and durability of the system [81,90–92] Co-catalysts are widely used to promote the separation of photocarriers and to create active sites to promote the photocatalytic reduction reaction. The addition of co-catalysts such as platinum, palladium or electron scavengers such as sacrificial agents like methanol can help to efficiently separate the photogenerated electron-hole pairs and reduce the chances of photo-corrosion [93,94]. Also, improved photocatalyst designs with better charge separation properties, such as heterojunction structures, can prevent the recombination of charge carriers and minimize self-degradation [95]. While significant advancements have been made in understanding and mitigating photo-corrosion, the challenge remains to develop polymers that can withstand long-term light exposure without significant degradation. Further research into stabilizing mechanisms and environmental impacts is essential for enhancing the durability of these materials.
2 Tailoring Material Properties through Surface Functionalization
The optimization and improvement of the characteristics of materials by changing their surface chemistry, which entails the attachment of specific functional groups, molecules, or nanostructures to the surface of a material, which customizes its physical, chemical, or biological properties. The ability to fine-tune material surfaces allows for targeted modifications without changing the bulk properties, making surface functionalization widely applicable in fields like biotechnology, energy storage, catalysis, and nanotechnology. The surface interaction control can create advanced materials with optimized performance for specific industrial or scientific applications. Each method offers unique advantages in terms of efficiency, durability, and the ability to impact specific surface characteristics. This process involves modifying the surface characteristics of materials to improve their functionality, such as increasing selectivity, bioavailability, and catalytic performance. Firstly, controlled plasma treatments involve the interaction of solid materials with reactive plasma species, leading to the formation of functional groups on the surface of polymers. By adjusting plasma parameters, such as the type of polymers and fluxes of reactive species, the surface wettability can be tailored almost arbitrarily. This method allows for precise modification of surface functionalities, making it a powerful technique for enhancing the properties of polymer-based photocatalysts [96]. The Oxygen plasma treatment of polypropylene (PP) creates surface functional groups like -OH and -C=O, transforming the surface from hydrophobic to hydrophilic. This change facilitates the efficient deposition of TiO2 nanoparticles, enhancing the photocatalytic degradation of pollutants such as methyl orange by up to 80% under UVA irradiation [97]. titanium dioxide (TiO2) and gold (Au)-doped TiO2 (TiO2–Au) composite nanofibers (NFs) for Photocatalytic reactions were performed with methyl blue (MB) aqueous solution under UV–Vis and different irradiation wavelengths [98]. Techniques such as the use of biodegradable polymers like chitosan and polydopamine have been explored for their potential to improve the selectivity and productivity of catalytic reactions [58], Based on the research conducted by Arya et al., which explained the coating catalysts with polymers, such as poly(methyl-methacrylate) (PMMA) or Nafion, the stability, activity, and selectivity of the catalysts are improved [58].
Secondly, surface engineering through polymer coating can enhance the mechanical and thermal stability of catalysts, as well as their photocatalytic activity. For instance, polymer coatings on photocatalysts like Hydroxyapatite/titania nanocomposites (TiHAp) or zinc oxide nanoparticles have shown increased efficiency in degrading organic pollutants. Additionally, the use of polymers like polyether sulfone (PES) as a stable support for TiO2-based photocatalysts further enhances their performance. These methods result in a photocatalytic system capable of degrading pollutants efficiently, with the potential for improving reusability due to its robust structure. Immobilizing enzymes on porous polymers, such as High Internal Phase Emulsion (polyHIPE) can significantly enhance their reusability. The optimized conditions for enzyme immobilization lead to high retention of activity over multiple cycles, demonstrating the potential for repeated use in various applications [99]. Thirdly, the formation of hydrogen bonds in polymers can induce dipole-dipole interactions, creating an inherent electric field that enhances charge transfer. The functionalization of donor monomers in porous polymer networks (PPNs) can significantly enhance photocatalytic activity for hydrogen production. Two types of tri-azatruxene (TAT)-based PPNs were designed and synthesized with and without hexyl (R) group to understand the relationship between the presence of functional groups and charge transfer efficiency [100]. Also, there has been significant improvement in the photocatalytic oxidation rates and selectivity of organic semiconductor materials, such as the conversion of benzylamine to N-benzylidene-benzylamine [101]. Therefore, surface functionalization techniques significantly influence the photocatalytic activity of polymer-based materials by promoting efficient deposition and enhancing overall performance. This underscores the potential of functionalized PPNs in renewable energy applications, particularly in the field of photocatalytic hydrogen production. The study provides a foundation for future research aimed at optimizing the design of PPNs for enhanced performance.
Carbon dioxide (CO2) is a greenhouse gas is released by human and industrial processes causing significant environmental impacts like global warming, ocean acidification, ecosystem disruption, and human health risks [102]. Carbon capture and storage (CCS) technology can help reduce emissions, but its widespread implementation faces challenges related to cost, infrastructure, and long-term storage security but must be balanced with biodiversity and local ecosystem protection [103]. However, chemical oxidation points towards the complete mineralization of pollutants to CO2, H2O, and inorganic ions, or at least conversion of pollutant molecules into harmless products is achievable with photo catalysis. The conversion of CO2 into solar fuels via photo-driven catalytic reduction presents a promising avenue toward achieving carbon neutrality using copper-based catalysts are prepared and explored for photo-driven photo-thermal CO2 reduction reaction [104]. The photocatalytic reduction of CO2 presents significant challenges that hinder its efficiency and practicality. Key issues include low CO2 uptake, poor light absorption, and complex reaction mechanisms. Addressing these challenges requires innovative strategies in catalyst design and optimization. Many photocatalysts, such as ceria (CeO2), exhibit low sunlight absorption and uncontrollable selectivity, necessitating modifications like doping and heterostructure development [105]. The recombination of charge carriers significantly impacts photocatalytic efficiency. Understanding and engineering heterojunctions can enhance charge separation and improve performance and the Ambiguities in reaction pathways and unclear charge transfer mechanisms complicate the optimization of photocatalytic processes [2,106–108].
The potential solution could involve developing advanced materials, such as carbon nitrides and metal oxide heterojunctions, can enhance CO2 reduction efficiency by improving light absorption and increasing active sites by hybrids for efficient photocatalytic reduction of CO2 to methanol [109–111]. A thorough understanding of the underlying mechanisms and characteristics of photocatalysts is essential for future advancements like the regulation of the d-band centre of nanoparticles for efficient photo-driven catalytic CO2 reduction [112,113]. Despite significant advancements, the intricate nature of photocatalytic systems and the demand for scalable solutions continue to pose essential challenges to achieving efficient CO2 reduction.
2.1 Properties of Polymers Relevant to Photocatalysis
Semiconductor-based photo catalysis has gained attention as a promising, eco-friendly solution for growing pollution issues. However, its commercialization is challenged by the low activity of pure semiconductors, quick charge recombination, and dependence on ultraviolet light. Recent advances in materials science have introduced polymers as effective support for semiconductor photocatalysts, helping to overcome these obstacles. Polymers can reduce charge carrier recombination, extending their lifetimes, and their narrower bandgap allows for a broader response to visible light. Moreover, polymers can efficiently scavenge photo-generated holes [114,115].
Photocatalysis is an environmentally friendly technology, and polymers can be used independently as photocatalysts for wastewater treatment without relying on semiconductor support. Polymers provide several benefits over conventional metal oxides, semiconductors, and inorganic nanocrystals. These advantages include flexible structures, adjustable porosity, high chemical durability, precisely defined micro-pores, robust binding sites for transition metal centers, and customizable conjugation. Organic polymers are especially well-suited for biomedical and environmental cleanup applications because they offer a safer alternative to semiconductors that contain hazardous and bio-accumulative heavy metals [114,116,117]. The versatility of polymers stems from their ability to combine various compounds, mold them into desired shapes, and modify their properties to create value-added materials. Polymers are used in a variety of forms, including foam, surface coating, powder, and membranes. Polymer powders are spherical plastics that have been finely split and created via processing and bulk or solution polymerization. The diameter of these spherical particles might vary from a few micrometers to more than 200 μm. Powdered polymers are essential for appearance, affordability, aesthetics, and chemical makeup. Polymer powders need to have a certain elemental composition, appropriate physical attributes, and uniform qualities across the sample for practical applications [118,119]. Polymers in photo catalysis have several properties critical to their performance in such applications.
Photocatalytic techniques have evolved, with the first effective methods using TiO2 as the photocatalyst. However, this catalyst absorbs only 4% of the sunlight spectrum, prompting ongoing efforts to design other catalysts that exhibit more activity under visible light irradiation [120]. Photocatalytic efficacy depends on several criteria, including the photocatalyst’s capacity to create electron-hole pairs with minimal recombination rates, its absorption and adsorption characteristics, and its physical states. Various photocatalysts, including polyoxometalates, metal-organic frameworks (MOFs), perovskites, and oxides (Fig. 5), have been used in composites for the photodegradation of many organic refractory contaminants, such as medicines, textile dyes, herbicides, and organic pollutants [121,122]. Polyoxometalates are anionic inorganic metal-oxygen clusters characterized by metallic elements in an octahedral oxygen environment, often exhibiting a high oxidation state (commonly d0 configuration). These materials have excellent thermal stability, significant light absorption in the near UV spectrum, and favorable redox properties. Their attributes have facilitated their use in photo catalysis for the degradation of organic pollutants, the reduction of heavy metals, and the reduction of CO2 [120,123].
Figure 5: Efficiency of organic pollutants using different Photocatalyst/Polymer composites [120]. Elsevier and Copyright clearance, License number (5875271060427), 24 September 2024, European Polymer Journal
Metal-organic frameworks (MOFs) are crystalline materials characterized by a periodic arrangement of metals or metal clusters and organic ligands linked by robust coordination linkages. Various varieties of MOFs with distinct characteristics may be produced based on the intended uses, owing to the myriad combinations of organic ligands and metals [124,125]. These benefits have facilitated the use of these materials across several domains, including biomedicine for drug delivery, gas storage, uranium extraction, and photo catalysis [126]. Perovskites are intriguing candidates for photo catalysis due to their excellent chemical and thermal stabilities, cheap synthesis costs, and notable diffusion lengths that provide improved separation of produced electron-hole pairs. Perovskites may be used in various domains, including X-ray imaging, photovoltaic cells, and light-emitting diode (LED) devices [127]. The predominant photocatalysts are metal oxides, with TiO2 and ZnO serving as notable examples. Manganese oxide, which is naturally plentiful, non-toxic, and has remarkable structural and chemical characteristics, acid resistance, cheap cost, and a narrower band gap energy than TiO2, was selected as the final photocatalyst for investigation in this work [128]. The intended photocatalysts are environmentally friendly and stable substances, but they are often produced in powder form, complicating and increasing the cost of their separation and regeneration during the photocatalytic process [129,130]. A solution to this problem is to attach it to solid matrices, which allows for more straightforward implementation. Recent research has concentrated on the encapsulation of photocatalytic powders inside diverse matrices, with a polymer generated by photopolymerization under LED irradiation at 405 nm recently used for this purpose [131]. Comparing photocatalytic efficiencies of different photocatalyst families is challenging due to their chemical and physical properties, operating conditions, reactor design, irradiation setup, film fixation methods, and substrate properties. The chosen photocatalysts, including several structural families such as polyoxometalates, MOFs, perovskites, and metal oxides, were analyzed under comparable circumstances.
Polymers with good electrical conductivity assist in the separation and transport of charge carriers (electrons and holes), reducing recombination and increasing photocatalytic efficiency. The polymer utilised in photocatalysts is evaluated for its electrical properties based on its dielectric constant, photocurrent responsiveness, electrical conductivity, and dielectric loss. Polymers can often be doped with conductive materials or modified to enhance their charge transport properties in a photocatalyst reaction [2]. The polymer’s structure, high solvation ability, high electroactive conductivity, ease of complex formation with alkali salts, and direct cation migration pathway contribute to its excellent dielectric and ion-related properties [114]. To drive photocatalytic reactions, charge carriers (electrons and holes) must be generated, separated, and transported. For this reason, the electrical characteristics of polymers play a crucial role in photo catalysis [132,133].
The dielectric constant of a polymer affects its ability to store and transmit electric charge. A higher dielectric constant can help stabilize charge separation, which is beneficial for photocatalytic efficiency. Polymer-based photocatalysts often have a high exciton binding energy due to their low dielectric constant [134]. Polymers that exhibit photoconductivity increase their electrical conductivity when exposed to light. This property is crucial in photocatalysis, where the polymer needs to efficiently conduct charge carriers generated by light absorption. Photocurrent measures the charge transfer on the composite surface during photocatalytic reactions. Upon exposure to visible light, various samples show an initial rise in current, signifying the separation of electron-hole pairs. After reaching a peak, the current gradually decreases until it stabilizes. When the light source is removed, the current decreases, indicating that the charges on the material’s surface are recombining [135]. The electrical properties of polymers, such as conductivity, dielectric constant, band gap, photoconductivity, and interface conductivity, play crucial roles in determining their effectiveness in photocatalysis.
Reactive oxygen species are frequently used in conventional photo catalysis to prioritize the breakdown of less hazardous contaminants, leaving trace levels of extremely toxic compounds behind. This limitation can be addressed by the selective recognition capabilities of polymers owing to its magnetic properties [136]. Polymers with good mechanical strength and flexibility to withstand physical stress during processing and application. Durability ensures long-term stability and functionality. Paramagnetic properties provide valuable insights into the presence of unpaired electrons and charge carriers within polymers. The paramagnetic properties of polymers are less commonly emphasized in photo catalysis compared to other properties like electrical conductivity or optical behavior. However, when polymers exhibit paramagnetic characteristics, these properties can influence the photocatalytic process. Polymers’ paramagnetic properties or those modified with paramagnetic elements (e.g., transition metals like iron or manganese) can create localized magnetic fields. These fields can influence the spin states of electrons and holes, potentially reducing their recombination rate and enhancing charge separation, which is beneficial for photocatalytic efficiency. By stabilizing the separated charge carriers, paramagnetic interactions prolong the lifetime of the charges, increasing the likelihood of their participation in redox reactions on the photocatalyst’s surface [137].
Polymers’ paramagnetic properties are responsive to external magnetic fields, allowing for the control and orientation of the photocatalytic material during the reaction. This control can optimize the exposure of active sites to light and reactants, potentially improving photocatalytic performance. This also serves as matrices for embedding magnetic nanoparticles, creating a composite material that benefits from both magnetic and photocatalytic properties. The magnetic nature can aid in charge separation, while the polymer matrix provides structural support and stability [138]. Photocatalysts frequently struggle with limited selectivity in pollutant degradation and reuse challenges due to their small size. Polymers with magnetic properties offer a promising solution, as they can enhance the recovery of photocatalyst composites.
The bandgap of the photocatalyst determines how much energy is required to start photo catalysis. Visible light (400–800 nm) makes up around 53% of solar energy, but UV light only makes up 5% of sunshine. It is crucial to create photocatalysts with bandgaps less than 3.0 eV so they can better absorb solar light and use visible light [31]. Common photocatalysts like TiO2 are limited by their inability to absorb visible light. Its absorption is limited to the ultraviolet (UV) area, which makes up just 3%–5% of sunlight, due to its large bandgap. To overcome this, several studies have concentrated on altering TiO2 to increase its visible light sensitivity and boost the efficiency of solar energy utilization. Polymers have emerged as promising alternatives due to their efficient visible light absorption, narrow band gap, easily produced under mild conditions, and exhibit excellent resistance to fading when exposed to light and tunable properties, they can also capture photo-generated holes. These metal-free catalysts have the potential to improve photo catalysis and support green chemistry, sustainable environments, and renewable energy sources. To start photocatalytic reactions, a polymer must be able to absorb light, especially in the UV and visible wavelengths. Some polymers can be engineered or doped to enhance their light absorption capabilities. In some cases, polymers need to be transparent or semi-transparent to allow light to reach embedded photocatalytic materials, such as nanoparticles or other active components [114,139].
2.5 Recent Advancements in Environmental Remediation Technologies
In recent years, environmental remediation technologies have advanced significantly, tackling a wide range of pollution problems, from improving air quality to contaminating soil and water [140]. The pressing need to lessen the negative impacts of urbanization, agriculture, and industry on ecosystems and human health is what is driving these developments. Some of the most noteworthy recent developments in a range of environmental restoration methods are examined in this article. Utilizing biological processes, bioremediation breaks down environmental contaminants [141]. Enhancing the efficacy and efficiency of microorganisms utilized in bioremediation has been the focus of recent developments. Scientists have been able to alter bacteria and fungi through genetic engineering to improve their capacity to degrade pollutants. For instance, Pseudomonas putida strains that have undergone genetic modification have been created to more effectively break down petroleum compounds at oil spill locations. Furthermore, to accelerate degradation processes, researchers are increasingly using bio-augmentation, which involves introducing certain strains to polluted locations. Phytoremediation, or the exploitation of plant-microbe interactions, is another potential approach [142]. Finding hyper accumulator plants that can draw heavy metals out of the soil and using mycorrhizal fungus to improve nutrient absorption and pollutant degradation are examples of advancements in this field. According to recent research, combining these strategies can result in polluted sites being cleaned up more quickly and efficiently. One innovative technique for environmental remediation is nanotechnology [143]. Nanomaterials are being developed for uses including soil cleanup and water treatment because of their large surface area and reactivity [143]. For example, groundwater tainted with chlorinated solvents is being treated using zero-valent iron nanoparticles. By reacting with pollutants, these nanoparticles successfully lessen their toxicity. Furthermore, using nanoscale photocatalysts, such titanium dioxide (TiO2), has demonstrated promise in the degradation of organic contaminants in water when exposed to ultraviolet light [142].
To make these materials suitable for large-scale applications, recent developments have concentrated on increasing their photocatalytic efficiency. To provide more effective and economical remediation options, hybrid systems that combine nanomaterials with conventional filtering techniques are also being explored. Advanced Oxidation Processes (AOPs) are chemical treatment techniques that break down organic contaminants by producing hydroxyl radicals [144]. More effective catalysts and creative ways to produce these radicals are examples of recent developments in AOPs. Electrokinetic remediation is a method that applies an electric field to mobilize contaminants in soils and sediments [145]. Recent advancements have focused on optimizing electrode materials and configurations to enhance the efficiency of contaminant extraction [146,147]. Innovative approaches, such as the use of conductive polymers and nanomaterials as electrodes, have been shown to improve the transport of ions and enhance the removal of heavy metals and organic pollutants [148]. Moreover, the integration of real-time monitoring systems with electrokinetic setups allows for better control over the remediation process, leading to more efficient and effective outcomes. This approach has proven particularly useful in addressing contaminated sites that are challenging to remediate using traditional methods.
Recent advancements in monitoring and modeling have enhanced our understanding of natural attenuation processes, where natural biological, chemical, and physical processes reduce contaminant concentrations [149]. Enhanced natural attenuation (ENA) techniques involve adding nutrients or electron donors to stimulate indigenous microbial populations, promoting faster degradation of contaminants [150]. In situ remediation techniques, which treat contaminants without removing them from the site, are gaining traction due to their cost-effectiveness and minimal disruption [151]. Technologies such as in situ chemical oxidation (ISCO) and in situ bioremediation are being refined to target specific contaminants and optimize treatment times [152]. The success of environmental remediation technologies also depends on policy frameworks and community involvement. Recent efforts have emphasized the importance of stakeholder engagement in remediation projects. Community-driven initiatives ensure that remediation efforts align with local needs and priorities, fostering greater acceptance and support for projects. Furthermore, regulatory frameworks are evolving to incorporate emerging technologies, promoting their adoption while ensuring environmental safety. Policymakers are increasingly recognizing the need for sustainable practices that prioritize both remediation effectiveness and ecological health. The field of environmental remediation is witnessing rapid advancements that are enhancing our ability to address contamination challenges. From bioremediation and nanotechnology to innovative chemical processes and community engagement, these developments are paving the way for more effective and sustainable remediation strategies. As we continue to confront environmental challenges, these technologies will play a crucial role in restoring ecosystems and protecting public health, underscoring the importance of ongoing research and collaboration across disciplines.
3 Sustainable Energy Technologies
Materials science innovations are being used to address climate change and energy supply issues, including efficient solar panels, high-capacity batteries, and heat-to-electricity conversion materials. These innovations primarily involve alloys, polymers, and porous materials, aim to reduce carbon footprint, fossil fuel reliance, and transition to sustainable energy production. Polymer-based nanomaterials application potential applications across various fields of materials science have sparked growing interest [2,153]. Polymer-based Photocatalysts can provide a protective matrix for inorganic semiconductors, improving their stability against factors such as corrosion, leaching, and mechanical stress.
Given the abundant and inexpensive nature of water, electrolysis has emerged as a leading method for sustainably producing hydrogen energy. Polymer-based photocatalysts play a crucial role in producing clean fuels like hydrogen through water splitting under sunlight powered by solar energy. This process is an environmentally friendly way to generate clean energy, contributing to the promising alternative to create an affordable, sustainable hydrogen economy, addressing both the global energy crisis and environmental concerns [153,154]. The primary factor contributing to the success of photocatalytic, electrocatalytic, and photoelectrocatalytic approaches in green fuel and chemical production is their reliance on renewable energy sources. While electrochemical technologies are closer to industrialization, their development hinges on the cost of electricity. Moreover, these technologies involve two separate steps: electricity generation and subsequent conversion to chemical energy, leading to efficiency losses. In contrast, photo (electro) catalytic processes directly convert solar energy into chemical energy, emulating photosynthesis. This method has the benefit of mobility because it produces fuels only from solar radiation and doesn’t need external electricity sources [3,155]. Recent advancements in materials science have focused on employing inorganic semiconductors to address the limitations of current photocatalysts. These drawbacks include a limited range of light absorption from the sun, quick charge carrier recombination, ineffective electron transport, and inadequate photostability [156].
These materials are crucial for the development of alternative energy sources, as they can harness abundant and renewable solar energy to drive chemical reactions. Semiconductor polymers have attracted attention for their potential use in photovoltaic solar cells. Their ability to absorb and emit light across the entire solar spectrum, combined with their photostability and higher efficiency, has led to their initial exploration as photocatalysts [157,158]. Substituting fossil fuel sources with non-fossil fuel alternatives is a significant challenge. Achieving a balance between essential factors such as non-polluting or minimally polluting energy sources and energy efficiency is more challenging than shown in research conducted at both laboratory and industrial scales. So, the main goal in looking for alternatives was to lower carbon dioxide emissions and other air pollutants caused by transportation, such as nitrogen oxides, sulfur dioxide, PM10, and PM2.5 [159]. These pollutants make the air quality worse and hurt people’s health and the health of other living things around the world. Numerous laws are already in place globally to substitute polluting fuels with renewable energy sources in varying quantities throughout the next few years. However, the structure of renewable energy growth considers its installation, generating, and storage capacities. The advancement of these renewable technologies is evolving swiftly in the present context [159].
Studios frequently switch between technologies and deciding to use any current technology for commercial scaling is a significant consideration. The increased financial cost is another factor to consider when deploying these renewable technologies. Nonetheless, governments around the world are offering incentives to encourage the use of these renewable energy sources. Like startups or corporations, solar, photovoltaic, and wind energy sectors have garnered substantial support in several aspects in recent years. Another critical consideration in evaluating these alternative energy options is the feasibility and accessibility of renewable energy sources, such as solar and wind, depending on geographic locations and climatic conditions. Wind energy is progressing consistently across Latin America and Africa [159,160]. We precisely evaluate the full cycle effect (from raw material extraction to ultimate energy consumption) of energy systems to determine its influence on health. Health hazards often lead to the establishment of three distinct categories: The first category comprises fuels such as biomass and coal, oil, wood, natural gas, etc., whose use produces significant solid waste and air pollution. Secondly, renewable energy includes energy sources with a low energy density, such as wind, hydro, and solar power systems that exhibit low emissions, primarily restricted to low-probability incidents (such as dam failures, equipment malfunctions, and fires). However, certain disadvantages include the engagement of larger areas that will necessitate costly and advanced infrastructure. High energy densities in the processed fuel, which result in minimal fuel requirements and minimal waste production for transformation, distinguish the nuclear sector, primarily consisting of nuclear fission technologies [161]. Consequently, the effective analysis of e their effects and determine their toxicity levels based on the varying emission levels produced by each energy type. Renewable energies are defined as energy supplies that remain inexhaustible despite being used. To put it another way, renewable energy sources, like hydroelectric power from water resources, can replenish themselves. There are primarily five categories of renewable energy. These include biomass derived from plants and other waste, wind energy, solar energy from the sun, hydroelectric energy from flowing water, and geothermal energy from the Earth’s internal heat. Fig. 6 depicts the conversions of renewable and sustainable clean energy, as well as their uses. Renewable energy, sometimes referred to as clean energy, does not generate further pollution or waste, unlike fossil fuel energy sources. These have a minimal carbon footprint and generate reduced greenhouse gas emissions. In recent years, clean energy has gained popularity as many governments and economies seek to reduce their reliance on heavily polluting fossil fuels. Renewable energy sources have fulfilled 28% of global electricity demands, with 96% derived from hydroelectric, solar, and wind technologies. The five foremost renewable energy technologies are as follows:
Figure 6: Transformations and utilization of renewable and sustainable clean energy [159]. Elsevier and Copyright clearance, License number (5875201387581), 24 September 2024, Energy Nexus
Water is an essential element for all organisms on Earth’s surface; nevertheless, it is usually contaminated with pollutants such as arsenic, sodium chloride, fluorides, and toxic compounds caused by human activities, including pesticides, fertilisers, and industrial waste. Sustainable water purification is crucial for improving public health and supporting the UN’s Sustainable Development Goals (SDGs), particularly SDG 6 (Clean Water and Sanitation). The World Health Organization (WHO) states that 844 million people lack access to safe drinking water, making water source purification a critical global concern. A considerable segment of the global populace encounters difficulties associated with substandard water quality [162–164].
Polymer photocatalysts have recently gained interest in water treatment as they are effective in breaking down organic contaminants including dyes and pesticides, in water, soil and atmosphere. These photocatalysts can break down organic pollutants like dyes, pesticides, and pharmaceuticals into simple, biodegradable, or harmless substances. They can even convert these pollutants into carbon dioxide and water, leaving no harmful byproducts. This makes them valuable in wastewater treatment and air purification, helping to reduce environmental pollution [165]. The economic advantages of polymer-based photocatalysts, such as their broad availability, affordability, effectiveness, ease of separation and reuse, and flexible designs, are what propel their application in wastewater remediation. These photocatalysts are composed of conventional photoactive materials such as metal complexes, transition metal oxides, and plasmonic metals mixed with photo-inactive polymers. Some organic polymers have built-in photoactivity, exhibiting excellent semiconducting properties and the ability to degrade organic dyes in water. When combined with photoactive polymers, the performance of traditional photoactive species can be significantly enhanced [32,36,166]. They can be utilized to develop self-cleaning surfaces that break down contaminants upon exposure to light, contributing to cleaner environments and reducing the need for chemical cleaners.
3.2 Photocatalysts for Environmental Remediation
Industrialization has led to a significant water pollution crisis, with dye contamination posing serious health risks. Water pollution can harm human health, aquatic life, and land fertility. A variety of water treatment methods are being explored to find environmentally friendly solutions. There is a pressing environmental concern that is escalating health risks associated with air, water, and soil pollution. Conventional techniques for treating water include sedimentation, adsorption, membrane filtration, biological and chemical treatments, and filtration. However, these methods often struggle to effectively remove a variety of pollutants, including pharmaceutical waste and household chemicals. Removing 70%–95% of organic impurities and solely focusing on a narrow range of pollutants is the limitation of biological therapy. There are drawbacks to ozone-based therapy, including its poor solubility and quick disintegration at high pH and temperatures [167]. Scientists seek innovative, sustainable solutions, particularly green technologies, to address these challenges. The removal or control of toxic pollutants, primarily from industrial and human activities, is a critical research priority. Water pollution often originates from industries like textiles, tanneries, pharmaceuticals, and agriculture. Industrial effluents can contaminate both surface and groundwater. Antibiotics, along with other pharmaceuticals and hospital waste, can be a major source of pollution in soil and water due to their interactions with organics and heavy metals. Gaseous pollutants released by factories, automobiles, and the use of pesticides include particulate matter, carbon monoxide, carbon dioxide, and VOCs. Pollution issues can be made worse by the reaction between these primary pollutants and secondary pollutants such ozone, sulphuric acid, nitric acid, and hydrogen peroxide. To reduce environmental contaminants such as waterborne pesticides, textile and tannery effluents, organics, inorganics, gaseous oxides, VOCs, and solid wastes, better and more efficient procedures must be developed.
However, various air and water pollution control methods exist, photocatalysis has emerged as a promising approach. Numerous photocatalytic materials have been created by researchers, such as carbon nanostructures, tungstate, bismuthates, vanadate’s, complicated oxyhalides, and metal oxides like TiO2, zinc oxide, iron oxide, copper oxide, and tungsten (VI) oxide. These substances have shown promise in the treatment of various organic and inorganic contaminants in various settings [2]. Photocatalysis using semiconductor nanostructures is a sustainable approach for environmental remediation. Under visible light, photocatalysts generate electron-hole pairs, which drive redox reactions and produce reactive oxygen species (ROS) like superoxide and hydroxyl radicals. These radicals can decompose harmful pollutants into less toxic substances. The photocatalytic process involves several steps, including electron-hole pair formation, oxygen reduction, and hydroxyl radical generation. Reaction selectivity in semiconductor photocatalysts is dependent on their band locations and redox potential [2,167].
Photocatalysis is considered one of the most appropriate technologies. It can mineralize refractory substances. Photocatalysis has garnered significant global attention compared to conventional techniques because of its notable advantages, including the use of abundant and renewable solar energy, effective pollutant degradation, straightforward and safe operational methods, and the absence of secondary pollution. Researchers have published a substantial volume of studies on various types and applications of photocatalysts, including research papers, reviews, and book chapters [168–170]. Environmental remediation applications extensively use photo catalysis, particularly in the breakdown of pollutants, which produces numerous free radicals. These photogenerated free radicals have sufficient oxidative capacity to oxidize organic and inorganic contaminants, as well as inactivate microorganisms in the environment. Photographic materials have some good qualities compared to other technologies. Still, they are expensive, not very efficient, and charges can mix, which makes photo catalysis under solar illumination not very commercially viable [171]. Consequently, significant efforts have focused on improving current photocatalytic materials to boost their photocatalytic efficacy, with a primary focus on environmental remediation, which includes pollutant degradation [172]. This text discusses the principles of heterogeneous photo catalysis in the environmental remediation of various pollutants, including water contaminants, pathogenic bacteria, gaseous pollutants, and solid waste. The text also extensively examines potential semiconductors and their modification methods. Fig. 7 also anticipates future advancements.
Figure 7: The principles of heterogeneous photo catalysis in environmental remediation [172]. Elsevier and Copyright Clearance License Number (5875190375890), 24 September 2024, Chinese Journal of Catalysis
The studies provide an overview of the accomplishments in photocatalysis; however, its concentration on particular materials or contaminants, such as organic pollutants, necessitates more thorough insights. Consequently, it is necessary to comprehensively analyse the recent developments and challenges in contemporary photocatalytic materials and their application in environmental remediation without limitation to any specific substance, pollutant or technique. A study conducted by Djurišić et al. examined visible-light photocatalysts and their potential applications in environmental contexts [173]. However, their work primarily focused on photocatalyst materials, and their information is outdated, given the rapid advancements and significant breakthroughs in this field in recent years. Recent years have shown significant progress that necessitates additional evaluation. Therefore, prompt examination is essential to explore recent advancements in the breakdown of environmental pollutants, a significant risk to human and societal advancement through photocatalysis, and the associated challenges and strategies for mitigation.
4 Conclusion and Recommendation
This review highlights the increasing significance of polymer-based photocatalysts in tackling essential environmental and energy issues. These materials have adjustable chemical characteristics, superior light absorption, and increased stability, making them an intriguing alternative to traditional inorganic photocatalysts. Polymer-based photocatalysts have considerable promise in environmental remediation, especially in the degradation of hazardous contaminants like organic dyes, insecticides, and medicines. Their capacity to effectively generate clean energy via methods such as water splitting for hydrogen production and CO2 reduction further emphasizes their flexibility and influence in renewable energy applications. The photocatalytic activity of these materials has been greatly improved thanks to progress in polymer chemistry, nano-structuring, and hybridization with semiconductors and metal nanoparticles. Moreover, their cost-efficiency, simplicity of separation, and reusability make them suitable contenders for extensive applications in water purification and air quality enhancement. Notwithstanding these developments, hurdles persist in enhancing the stability, efficiency, and scalability of polymer-based photocatalytic systems. Future research should focus on developing more robust materials that have improved long-term stability and perform well in various environmental and energy applications. Polymer-based photocatalysts offer a long-term solution to minimize environmental degradation and encourage renewable energy sources to pave the way for a cleaner and greener future.
Acknowledgement: The authors acknowledge the effort of Sikiru Surajudeen Olalekan for the excellent formulation of the research ideal and coordination. The authors all appreciate the School of Physics & Materials Sciences, Faculty of Applied Sciences, Universiti Teknologi Mara Shah Alam Malaysia.
Funding Statement: The authors received no specific funding for this study.
Author Contributions: Formulated the research ideal, and wrote the main manuscript: Surajudeen Sikiru; Wrote the literature review: Yusuf Olanrewaju Busari, Mohd Muzamir Mahat, John Oluwadamilola Olutoki, Sanusi Yekinni Kolawole. All authors reviewed the results and approved the final version of the manuscript.
Availability of Data and Materials: Not applicable.
Ethics Approval: Not applicable.
Conflicts of Interest: The authors declare no conflicts of interest to report regarding the present study.
References
1. Mohtaram S, Mohtaram MS, Sabbaghi S, You X, Wu W, Golsanami N. Enhancement strategies in CO2 conversion and management of biochar supported photocatalyst for effective generation of renewable and sustainable solar energy. Energy Convers Manage. 2024;300:117987. doi:10.1016/j.enconman.2023.117987. [Google Scholar] [CrossRef]
2. Saianand G, Gopalan A-I, Wang L, Venkatramanan K, Roy VA, Sonar, et al. Conducting polymer based visible light photocatalytic composites for pollutant removal: progress and prospects. Environ Technol Innov. 2022;28:102698. doi:10.1016/j.eti.2022.102698. [Google Scholar] [CrossRef]
3. Liras M, Barawi M. Hybrid materials based on conjugated polymers and inorganic semiconductors as photocatalysts: from environmental to energy applications. Chem Soc Rev. 2019;48(22):5454–87. doi:10.1039/C9CS00377K. [Google Scholar] [PubMed] [CrossRef]
4. Singh S, Mahalingam H, Singh PK. Polymer-supported titanium dioxide photocatalysts for environmental remediation: a review. Appl Catal A: Gen. 2013;462:178–95. [Google Scholar]
5. Pandey B, Singh P, Kumar V. Photocatalytic-sorption processes for the removal of pollutants from wastewater using polymer metal oxide nanocomposites and associated environmental risks. Environ Nanotechnol, Monit Manage. 2021;16(1):100596. [Google Scholar]
6. Sikiru S, Abiodun OA, Sanusi YK, Sikiru YA, Soleimani H, Yekeen N, et al. A comprehensive review on nanotechnology application in wastewater treatment a case study of metal-based using green synthesis. J Environ Chem Eng. 2022;10(4):108065. doi:10.1016/j.jece.2022.108065. [Google Scholar] [CrossRef]
7. Zhang L, Du W, Nautiyal A, Liu Z, Zhang X. Recent progress on nanostructured conducting polymers and composites: synthesis, application and future aspects. Sci China Mater. 2018;61(3):303–52. doi:10.1007/s40843-017-9206-4. [Google Scholar] [CrossRef]
8. Holkar CR, Jadhav AJ, Pinjari DV, Mahamuni NM, Pandit AB. A critical review on textile wastewater treatments: possible approaches. J Environ Manage. 2016;182:351–66. doi:10.1016/j.jenvman.2016.07.090. [Google Scholar] [PubMed] [CrossRef]
9. Hethnawi A, Nassar NN, Manasrah AD, Vitale G. Polyethylenimine-functionalized pyroxene nanoparticles embedded on Diatomite for adsorptive removal of dye from textile wastewater in a fixed-bed column. Chem Eng J. 2017;320:389–404. doi:10.1016/j.cej.2017.03.057. [Google Scholar] [CrossRef]
10. Katheresan V, Kansedo J, Lau SY. Efficiency of various recent wastewater dye removal methods: a review. J Environ Chem Eng. 2018;6(4):4676–97. doi:10.1016/j.jece.2018.06.060. [Google Scholar] [CrossRef]
11. Ganiyu SA, Ajumobi OO, Lateef SA, Sulaiman KO, Bakare IA, Qamaruddin M, et al. Boron-doped activated carbon as efficient and selective adsorbent for ultra-deep desulfurization of 4,6-dimethyldibenzothiophene. Chem Eng J. 2017;321:651–61. doi:10.1016/j.cej.2017.03.132. [Google Scholar] [CrossRef]
12. Kumari H, Sonia, Ranga R, Chahal S, Devi S, et al. A review on photocatalysis used for wastewater treatment: dye degradation. Water Air Soil Pollut. 2023;234(6):349. [Google Scholar] [PubMed]
13. Algar L, Sicilia MD, Rubio S. Ribbon-shaped supramolecular solvents: synthesis, characterization and potential for making greener the microextraction of water organic pollutants. Talanta. 2023;255:124227. doi:10.1016/j.talanta.2022.124227. [Google Scholar] [PubMed] [CrossRef]
14. Huang L, Yang Z-H, Yan L-J, Alhassan SI, Gang H-Y, Ting W, et al. Preparation of 2D carbon ribbon/Al2O3 and nitrogen-doped carbon ribbon/Al2O3 by using MOFs as precursors for removing high-fluoride water. Trans Nonferrous Met Soc China. 2021;31(7):2174–88. doi:10.1016/S1003-6326(21)65647-9. [Google Scholar] [CrossRef]
15. Foster JE, Mujovic S, Groele J, Blankson IM. Towards high throughput plasma based water purifiers: design considerations and the pathway towards practical application. J Phys D: Appl Phys. 2018;51(29):293001. doi:10.1088/1361-6463/aac816. [Google Scholar] [CrossRef]
16. Sohail M, Rauf S, Irfan M, Hayat A, Alghamdi MM, El-Zahhar AA, et al. Recent developments, advances and strategies in heterogeneous photocatalysts for water splitting. Nanoscale Adv. 2024;6(5):1286–330. doi:10.1039/D3NA00442B. [Google Scholar] [PubMed] [CrossRef]
17. Ye Y, Jin J, Chen F, Dionysiou DD, Feng Y, Liang B, et al. Removal and recovery of aqueous U(VI) by heterogeneous photocatalysis: progress and challenges. Chem Eng J. 2022;450:138317. doi:10.1016/j.cej.2022.138317. [Google Scholar] [CrossRef]
18. Hübner U, Spahr S, Lutze H, Wieland A, Rüting S, Gernjak W, et al. Advanced oxidation processes for water and wastewater treatment—guidance for systematic future research. Heliyon. 2024;10(9):e30402. doi:10.1016/j.heliyon.2024.e30402. [Google Scholar] [PubMed] [CrossRef]
19. Li Y, Liu Y, Zhan Y, Zhang Y, Zhao X, Yang M, et al. Peracetic acid-induced nanoengineering of Fe-based metallic glass ribbon in application of efficient drinking water treatment. Appl Catal B Environ Energy. 2024;355:124161. doi:10.1016/j.apcatb.2024.124161. [Google Scholar] [CrossRef]
20. Jin C, Peng H, Zeng X, Liu Z, Ding D. Hierarchical assembly of NiFe-PB-derived bimetallic phosphides on 3D Ti3C2 MXene ribbon networks for efficient oxygen evolution. Chem Phys Mater. 2024;3(1):118–24. [Google Scholar]
21. Fujishima A, Honda K. Mechanism of anodic dissolution reaction of ZnO single crystal electrode under irradiation. Denki Kagaku Oyobi Kogyo Butsuri Kagaku. 1972;40(1):33–8. doi:10.5796/kogyobutsurikagaku.40.33. [Google Scholar] [CrossRef]
22. Wu H, Kong XY, Wen X, Chai SP, Lovell EC, Tang J, et al. Metal-organic framework decorated cuprous oxide nanowires for long-lived charges applied in selective photocatalytic CO2 reduction to CH4. Angew Chem Int Ed. 2021;60(15):8455–9. doi:10.1002/anie.202015735. [Google Scholar] [PubMed] [CrossRef]
23. Ayodhya D, Veerabhadram G. Stable and efficient graphitic carbon nitride nanosheet-supported ZnS composite catalysts toward competent catalytic performance for the reduction of 4-nitrophenol using NaBH4. Mater Today Sustain. 2019;5:100015. [Google Scholar]
24. Abdelnasser S, Al Sakkaf R, Palmisano G. Environmental and energy applications of TiO2 photoanodes modified with alkali metals and polymers. J Environ Chem Eng. 2021;9(1):104873. doi:10.1016/j.jece.2020.104873. [Google Scholar] [CrossRef]
25. Biswas B, Warr LN, Hilder EF, Goswami N, Rahman MM, Churchman JG, et al. Biocompatible functionalisation of nanoclays for improved environmental remediation. Chem Soc Rev. 2019;48(14):3740–70. doi:10.1039/C8CS01019F. [Google Scholar] [PubMed] [CrossRef]
26. Ago H, Okada S, Miyata Y, Matsuda K, Koshino M, Ueno K, et al. Science of 2.5 dimensional materials: paradigm shift of materials science toward future social innovation. Sci Technol Adv Mater. 2022;23(1):275–99. doi:10.1080/14686996.2022.2062576. [Google Scholar] [PubMed] [CrossRef]
27. Abolhasani M, Kumacheva E. The rise of self-driving labs in chemical and materials sciences. Nat Synth. 2023;2(6):483–92. doi:10.1038/s44160-022-00231-0. [Google Scholar] [CrossRef]
28. Nosaka Y, Nosaka A. Introduction to photocatalysis: from basic science to applications. Cambridge: The Royal Society of Chemistry; 2016. [Google Scholar]
29. Lopez EIG, Palmisano L. Materials science in photocatalysis. Amsterdam: Elsevier; 2021. [Google Scholar]
30. Banerjee T, Podjaski F, Kröger J, Biswal BP, Lotsch BV. Polymer photocatalysts for solar-to-chemical energy conversion. Nat Rev Mater. 2021;6(2):168–90. doi:10.1038/s41578-020-00254-z. [Google Scholar] [CrossRef]
31. Dai C, Liu B. Conjugated polymers for visible-light-driven photocatalysis. Energy Environ Sci. 2020;13(1):24–52. doi:10.1039/C9EE01935A. [Google Scholar] [CrossRef]
32. EL-Mekkawi DM. Polymer-based photocatalysis for remediation of wastewater contaminated with organic dyes. In: Polymer technology in dye-containing wastewater. Singapore: Springer Nature Singapore; 2022. vol. 1, p. 57–100. [Google Scholar]
33. Jayakumar J, Chou HH. Recent advances in visible-light-driven hydrogen evolution from water using polymer photocatalysts. ChemCatChem. 2020;12(3):689–704. doi:10.1002/cctc.201901725. [Google Scholar] [CrossRef]
34. Bai S, Wang L, Li Z, Xiong Y. Facet-engineered surface and interface design of photocatalytic materials. Adv Sci. 2017;4(1):1600216. doi:10.1002/advs.v4.1. [Google Scholar] [CrossRef]
35. Hasnan NSN, Mohamed MA, Anuar NA, Sukur MFA, Yusoff SFM, Mokhtar WNAW, et al. Emerging polymeric-based material with photocatalytic functionality for sustainable technologies. J Ind Eng Chem. 2022;113:32–71. doi:10.1016/j.jiec.2022.06.009. [Google Scholar] [CrossRef]
36. Kumar R, Travas-Sejdic J, Padhye LP. Conducting polymers-based photocatalysis for treatment of organic contaminants in water. Chem Eng J Adv. 2020;4:100047. doi:10.1016/j.ceja.2020.100047. [Google Scholar] [CrossRef]
37. Gong Y, Li M, Li H, Wang Y. Graphitic carbon nitride polymers: promising catalysts or catalyst supports for heterogeneous oxidation and hydrogenation. Green Chem. 2015;17(2):715–36. doi:10.1039/C4GC01847H. [Google Scholar] [CrossRef]
38. Zhou H, Wang H, Yue C, He L, Li H, Zhang H, et al. Photocatalytic degradation by TiO2-conjugated/coordination polymer heterojunction: preparation, mechanisms, and prospects. Appl Catal B Environ Energy. 2023;344:123605. [Google Scholar]
39. Huang C, Wen J, Shen Y, He F, Mi L, Gan Z, et al. Dissolution and homogeneous photocatalysis of polymeric carbon nitride. Chem Sci. 2018;9(41):7912–5. doi:10.1039/C8SC03855D. [Google Scholar] [PubMed] [CrossRef]
40. Dey A, Gogate PR. Nanocomposite photocatalysts-based wastewater treatment. In: Handbook of nanomaterials for wastewater treatment. Elsevier; 2021. p. 779–809. [Google Scholar]
41. Olatunji O. Classification of natural polymers. In: Natural polymers: industry techniques and applications. Cham: Springer; 2016. p. 1–17. [Google Scholar]
42. Melinte V, Stroea L, Chibac-Scutaru AL. Polymer nanocomposites for photocatalytic applications. Catalysts. 2019;9(12):986. doi:10.3390/catal9120986. [Google Scholar] [CrossRef]
43. Zhang Y, Zhang G-L, Wang Y-T, Ma Z, Yang T-Y, Zhang T, et al. In-situ synthesized porphyrin polymer/TiO2 composites as high-performance Z-scheme photocatalysts for CO2 conversion. J Colloid Interface Sci. 2021;596:342–51. doi:10.1016/j.jcis.2021.03.104. [Google Scholar] [PubMed] [CrossRef]
44. Xu F, Yu J. TiO2-based S-scheme photocatalyst. In: Interface science and technology. Elsevier; 2023. vol. 35, p. 133–74. [Google Scholar]
45. Anegbe B, Ifijen IH, Maliki M, Uwidia IE, Aigbodion AI. Graphene oxide synthesis and applications in emerging contaminant removal: a comprehensive review. Environ Sci Eur. 2024;36(1):15. doi:10.1186/s12302-023-00814-4. [Google Scholar] [CrossRef]
46. Yang C, Wang P, Li J, Wang Q, Xu P, You S, et al. Photocatalytic PVDF ultrafiltration membrane blended with visible-light responsive Fe(III)-TiO2 catalyst: degradation kinetics, catalytic performance and reusability. Chem Eng J. 2021;417:129340. doi:10.1016/j.cej.2021.129340. [Google Scholar] [CrossRef]
47. Mohd Hir ZA, Abdullah AH, Zainal Z, Lim HN. Visible light-active hybrid film photocatalyst of polyethersulfone-reduced TiO2: photocatalytic response and radical trapping investigation. J Mater Sci. 2018;53:13264–79. doi:10.1007/s10853-018-2570-3. [Google Scholar] [CrossRef]
48. Umaru D, Hafeez HY, Mohammed J, Suleiman AB, Ndikilar CE, Zakariyya Y. A review of recent progress in solar fuel (Hydrogen) generation via photocatalytic water-splitting of cadmium sulfide (CdS) based photocatalyst. Appl Surf Sci Adv. 2023;18:100520. doi:10.1016/j.apsadv.2023.100520. [Google Scholar] [CrossRef]
49. Gao P, Liu J, Sun DD, Ng W. Graphene oxide-CdS composite with high photocatalytic degradation and disinfection activities under visible light irradiation. J Hazard Mater. 2013;250:412–20. [Google Scholar] [PubMed]
50. Jiang X, Wang J. Enhanced photocatalytic activity of three-dimensional TiO2/reduced graphene oxide aerogel by efficient interfacial charge transfer. Appl Surf Sci. 2023;612:155849. doi:10.1016/j.apsusc.2022.155849. [Google Scholar] [CrossRef]
51. Tibi F, Park S-J, Kim J. Improvement of membrane distillation using PVDF membrane incorporated with TiO2 modified by silane and optimization of fabricating conditions. Membranes. 2021;11(2):95. doi:10.3390/membranes11020095. [Google Scholar] [PubMed] [CrossRef]
52. Ngang H, Ooi B, Ahmad A, Lai S. Preparation of PVDF-TiO2 mixed-matrix membrane and its evaluation on dye adsorption and UV-cleaning properties. Chem Eng J. 2012;197:359–67. doi:10.1016/j.cej.2012.05.050. [Google Scholar] [CrossRef]
53. Guo Z, Dai F, Yin H, Zhang M, Xing J, Wang L. The dual role of Au nanoparticles in the surface plasmon resonance enhanced photocatalyst Au/g-C3N4. Coll Interface Sci Commun. 2022;48:100615. doi:10.1016/j.colcom.2022.100615. [Google Scholar] [CrossRef]
54. Sun Y, Zhang W, Li Q, Liu H, Wang X. Preparations and applications of zinc oxide based photocatalytic materials. Adv Sens Energy Mat. 2023;2(3):100069. [Google Scholar]
55. Gahlot S, Dappozze F, Mishra S, Guillard C. High surface area g-C3N4 and g-C3N4-TiO2 photocatalytic activity under UV and Visible light: impact of individual component. J Environ Chem Eng. 2021;9(4):105587. doi:10.1016/j.jece.2021.105587. [Google Scholar] [CrossRef]
56. Li F, Tang M, Li T, Zhang L, Hu C. Two-dimensional graphene/g-C3N4 in-plane hybrid heterostructure for enhanced photocatalytic activity with surface-adsorbed pollutants assistant. Appl Catal B: Environ. 2020;268:118397. doi:10.1016/j.apcatb.2019.118397. [Google Scholar] [CrossRef]
57. Ramar P, Raghavendra V, Murugan P, Samanta D. Immobilization of polymers to surfaces by click reaction for photocatalysis with recyclability. Langmuir. 2022;38(44):13344–57. doi:10.1021/acs.langmuir.2c00809. [Google Scholar] [PubMed] [CrossRef]
58. Arya RK, Thapliyal D, Pandit A, Gora S, Banerjee C, Verros GD, et al. Polymer coated functional catalysts for industrial applications. Polymers. 2023;15(9):2009. doi:10.3390/polym15092009. [Google Scholar] [PubMed] [CrossRef]
59. Lin X, Fang H, Wang L, Sun D, Zhao G, Xu J. Photocatalytic degradation of sulfamethoxazole and enrofloxacin in water using electrospun composite photocatalytic membrane. Water. 2024;16(2):218. doi:10.3390/w16020218. [Google Scholar] [CrossRef]
60. Cui Z, Yuan R, Chen H, Zhou B, Zhu B, Zhang C. Application of polyaniline-based photocatalyst in photocatalytic degradation of micropollutants in water: a review. J Water Proc Eng. 2024;59:104900. doi:10.1016/j.jwpe.2024.104900. [Google Scholar] [CrossRef]
61. Ruziwa DT, Oluwalana AE, Mupa M, Meili L, Selvasembian R, Nindi MM, et al. Pharmaceuticals in wastewater and their photocatalytic degradation using nano-enabled photocatalysts. J Water Proc Eng. 2023;54:103880. doi:10.1016/j.jwpe.2023.103880. [Google Scholar] [CrossRef]
62. Huynh TMT, Nguyen DD, Hoang NH, Phan TH. Reversible tuning of surface properties of graphene-like material via covalently functionalized hydrophobic layer. Crystals. 2023;13(4):635. doi:10.3390/cryst13040635. [Google Scholar] [CrossRef]
63. Asthana N, Kumar D, Kyzas GZ. Graphene-reinforced hybrid polymer nanocomposites-based biosensor implementation for environmental remediation. Macromolecular Symposia. 2024;413(2):2300074. doi:10.1002/masy.202300074. [Google Scholar] [CrossRef]
64. Long Q, Chen L, Zong Y, Wan X, Liu F, Luo H, et al. Photocatalytically self-cleaning graphene oxide nanofiltration membranes reinforced with bismuth oxybromide for high-performance water purification. J Coll Interface Sci. 2024;675:958–69. doi:10.1016/j.jcis.2024.07.027. [Google Scholar] [PubMed] [CrossRef]
65. Abdelsalam H, Sakr MA, Saroka VA, Abd-Elkader OH, Zhang Q. Nanoporous graphene quantum dots constructed from nanoribbon superlattices with controllable pore morphology and size for wastewater treatment. Surf Inter. 2023;40:103109. doi:10.1016/j.surfin.2023.103109. [Google Scholar] [CrossRef]
66. Wang Y, Liu Y, Hu X, Li Y, Tu H, Wang C, et al. Rational structure design for enhanced uranium(VI) capture and beyond: from carbon nanotubes to graphene oxide nanoribbons. J Mol Liq. 2021;323:114639. doi:10.1016/j.molliq.2020.114639. [Google Scholar] [CrossRef]
67. Huang Z, Shen L, Lin H, Li B, Chen C, Xu Y, et al. Fabrication of fibrous MXene nanoribbons (MNRs) membrane with efficient performance for oil-water separation. J Membr Sci. 2022;661:120949. doi:10.1016/j.memsci.2022.120949. [Google Scholar] [CrossRef]
68. Kanoun MB, Ahmed F, Awada C, Jonin C, Brevet P-F. Band gap engineering of Au doping and Au-N codoping into anatase TiO2 for enhancing the visible light photocatalytic performance. Int J Hydrog Energy. 2024;51:907–13. doi:10.1016/j.ijhydene.2023.10.244. [Google Scholar] [CrossRef]
69. Mikolajczyk A, Wyrzykowska E, Mazierski, Grzyb T, Wei Z, Kowalska E, et al. Visible-light photocatalytic activity of rare-earth-metal-doped TiO2: experimental analysis and machine learning for virtual design. Appl Catal B: Environ Energy. 2024;346:123744. doi:10.1016/j.apcatb.2024.123744. [Google Scholar] [CrossRef]
70. Kim MJ, Hassan MA, Lee C, Jung WG, Bae H, Jeon S, et al. Maximizing photoelectrochemical performance in metal-oxide hybrid composites via amorphous exsolution—a new exsolution mechanism for heterogeneous catalysis. Small. 2024;20(18):2308934. doi:10.1002/smll.v20.18. [Google Scholar] [CrossRef]
71. Ovari T-R, Trufán B, Katona G, Szabó G, Muresan LM. Correlations between the anti-corrosion properties and the photocatalytic behavior of epoxy coatings incorporating modified graphene oxide deposited on a zinc substrate. RSC Adv. 2024;14(16):10826–41. doi:10.1039/D4RA00413B. [Google Scholar] [PubMed] [CrossRef]
72. Liu X-R, Sheng X-X, Yuan X-Y, Liu J-K, Sun X-W, Yang X-H. Research on correlation between corrosion resistance and photocatalytic activity of molybdenum zinc oxide modified by carbon quantum dots pigments. Dyes Pigm. 2020;175:108148. doi:10.1016/j.dyepig.2019.108148. [Google Scholar] [CrossRef]
73. Albistur A, Rivero PJ, Esparza J, Rodríguez R. Evaluation of the photocatalytic activity and anticorrosion performance of electrospun fibers doped with metallic oxides. Polymers. 2021;13(12):2011. doi:10.3390/polym13122011. [Google Scholar] [PubMed] [CrossRef]
74. Ananthasubramanian P, Sahay R, Raghavan N. Investigation of the surface mechanical properties of functionalized single-walled carbon nanotube (SWCNT) reinforced PDMS nanocomposites using nanoindentation analysis. RSC Adv. 2024;14(22):15249–60. doi:10.1039/D4RA02717E. [Google Scholar] [PubMed] [CrossRef]
75. Buhari AS, Abdulrahman AS, Lawal SA, Abdulkareem AS, Muriana RA, Jimoh OT, et al. Mechanical and corrosion protection characteristics of CNTs/epoxy resin nanocomposite coating on buried API 5L X65 steel storage tank. J Phys Sci. 2023;34(1):87–108. doi:10.21315/jps2023.34.1.8. [Google Scholar] [CrossRef]
76. Fatima R, Warsi MF, Zulfiqar S, Ragab SA, Shakir I, Sarwar MI. Nanocrystalline transition metal oxides and their composites with reduced graphene oxide and carbon nanotubes for photocatalytic applications. Ceram Int. 2020;46(10):16480–92. doi:10.1016/j.ceramint.2020.03.213. [Google Scholar] [CrossRef]
77. Dai W, Xu H, Yu J, Hu X, Luo X, Tu X, et al. Photocatalytic reduction of CO2 into methanol and ethanol over conducting polymers modified Bi2WO6 microspheres under visible light. Appl Surf Sci. 2015;356:173–80. doi:10.1016/j.apsusc.2015.08.059. [Google Scholar] [CrossRef]
78. Zhu H, Chen X, Zheng Z, Ke X, Jaatinen E, Zhao J, et al. Mechanism of supported gold nanoparticles as photocatalysts under ultraviolet and visible light irradiation. ChemComm. 2009;48:7524–6. [Google Scholar]
79. Sweetman MJ, May S, Mebberson N, Pendleton P, Vasilev K, Plush SE, et al. Activated carbon, carbon nanotubes and graphene: materials and composites for advanced water purification. C–J Carbon Res. 2017;3(2):18. doi:10.3390/c3020018. [Google Scholar] [CrossRef]
80. Lin X, Sun M, Yao Y, Yuan X. In situ construction of N/Ti3+ codoped triphasic TiO2 layer on TiO2 nanotube arrays to improve photoelectrochemical performance. Electrochim Acta. 2018;291:319–27. doi:10.1016/j.electacta.2018.09.099. [Google Scholar] [CrossRef]
81. Farooq A, Anwar M, Somaily H, Zulfiqar S, Warsi MF, Din MI, et al. Fabrication of Ag-doped magnesium aluminate/rGO composite: a highly efficient photocatalyst for visible light-driven photodegradation of crystal violet and phenol. Physica B Condens Matter. 2023;650:414508. doi:10.1016/j.physb.2022.414508. [Google Scholar] [CrossRef]
82. Zhu YY, He YY, Li YX, Liu CH, Lin W. Heterogeneous porous synergistic photocatalysts for organic transformations. Chem Eur J. 2024;30(37):e202400842. doi:10.1002/chem.v30.37. [Google Scholar] [CrossRef]
83. Sharma M, Sajwan D, Gouda A, Sharma A, Krishnan V. Recent progress in defect-engineered metal oxides for photocatalytic environmental remediation. Photochem Photobiol. 2024;100:830–96. doi:10.1111/php.13959. [Google Scholar] [PubMed] [CrossRef]
84. Chatterjee A, Jana AK, Basu JK. Silica supported binary metal organic framework for removing organic dye involving combined effect of adsorption followed by photocatalytic degradation. Mater Res Bull. 2021;138:111227. doi:10.1016/j.materresbull.2021.111227. [Google Scholar] [CrossRef]
85. Riaz U, Ashraf S, Kashyap J. Enhancement of photocatalytic properties of transitional metal oxides using conducting polymers: a mini review. Polymer. 2015;71:75–90. [Google Scholar]
86. Theerthagiri J, Chandrasekaran S, Salla S, Elakkiya V, Senthil R, Nithyadharseni P, et al. Recent developments of metal oxide based heterostructures for photocatalytic applications towards environmental remediation. J Solid State Chem. 2018;267:35–52. doi:10.1016/j.jssc.2018.08.006. [Google Scholar] [CrossRef]
87. Kumar SG, Rao KSRK. Comparison of modification strategies towards enhanced charge carrier separation and photocatalytic degradation activity of metal oxide semiconductors (TiO2, WO3 and ZnO). Appl Surf Sci. 2017;391:124–48. doi:10.1016/j.apsusc.2016.07.081. [Google Scholar] [CrossRef]
88. Alshammari KF. Recent advances of piezo-catalysis and photocatalysis for efficient environmental remediation. Luminescence. 2024;39(6):e4808. doi:10.1002/bio.v39.6. [Google Scholar] [CrossRef]
89. Xi Q, Liu J, Xie F, Jian A, Sun Z, Zhou A, et al. Electron-parking engineering assisted ZnIn2S4/Mo2TiC2-Ru photocatalytic hydrogen evolution for efficient solar energy conversion and storage. Appl Catal B Environ Energy. 2024;355:124184. doi:10.1016/j.apcatb.2024.124184. [Google Scholar] [CrossRef]
90. Kuang C, Tan P, Javed M, Khushi HH, Nadeem S, Iqbal S, et al. Boosting photocatalytic interaction of sulphur doped reduced graphene oxide-based S@rGO/NiS2 nanocomposite for destruction of pathogens and organic pollutant degradation caused by visible light. Inorg Chem Commun. 2022;141:109575. doi:10.1016/j.inoche.2022.109575. [Google Scholar] [CrossRef]
91. Meng S, Jiang X, Nan Z. Amine functionalized Fe doped g-C3N4 for rapidly and thoroughly selective degradation of anionic organic pollutants. Surf and Interf. 2023;42:103390. [Google Scholar]
92. Nawaz R, Aziz MH, Asif M, Noor F, Aligayev A, Ali SM, et al. Evaluation of tetracycline photocatalytic degradation using NiFe2O4/CeO2/GO nanocomposite for environmental remediation: in silico molecular docking, antibacterial performance, degradation pathways, and DFT calculations. Sep Purif Technol. 2024;23:128074. [Google Scholar]
93. Arzate-Salgado SY, Morales-Pérez AA, Solís-López M, and Ramírez-Zamora RM. Evaluation of metallurgical slag as a Fenton-type photocatalyst for the degradation of an emerging pollutant: Diclofenac. Catalysis Today. 2016;266:126–35. doi:10.1016/j.cattod.2015.09.026. [Google Scholar] [CrossRef]
94. Toledo-Camacho SY, Rey A, Maldonado M, Llorca J, Contreras S, and Medina F. Photocatalytic hydrogen production from water-methanol and-glycerol mixtures using Pd/TiO2(-WO3) catalysts and validation in a solar pilot plant. International Journal of Hydrogen Energy. 2021;46(73):36152–66. doi:10.1016/j.ijhydene.2021.08.141. [Google Scholar] [CrossRef]
95. Zou X, et al. Enhanced visible-light photocatalytic degradation of tetracycline antibiotic by 0D/2D TiO2(B)/BiOCl Z-scheme heterojunction: performance, reaction pathways, and mechanism investigation. Applied Surface Science. 2023;630:157532. doi:10.1016/j.apsusc.2023.157532. [Google Scholar] [CrossRef]
96. Vesel A, Mozetic M. New developments in surface functionalization of polymers using controlled plasma treatments. J Phy D: App Phy. 2017;50(29):293001. doi:10.1088/1361-6463/aa748a. [Google Scholar] [CrossRef]
97. Zajac K, Macyk J, Szajna K, Krok F, Macyk W, Kotarba A. Functionalization of polypropylene by TiO2 photocatalytic nanoparticles: on the importance of the surface oxygen plasma treatment. Nanomaterials. 2024;14(16):1372. doi:10.3390/nano14161372. [Google Scholar] [PubMed] [CrossRef]
98. Yang X, Salles V, Maillard M, Kaneti YV, Liu M, Journet C, et al. Fabrication of Au functionalized TiO2 nanofibers for photocatalytic application. J Nan Res. 2019;21:1–13. [Google Scholar]
99. Sahin B, Ozbey-Unal B, Dizge N, Keskinler B, Balcik C. Optimization of immobilized urease enzyme on porous polymer for enhancing the stability, reusability and enzymatic kinetics using response surface methodology. Coll Surf. B Biointerfaces. 2024;240:113986. doi:10.1016/j.colsurfb.2024.113986. [Google Scholar] [PubMed] [CrossRef]
100. Kim J, Jeon JP, Kim YH, Anh NTD, Chung K, Seo JM, et al. Simple functionalization of a donor monomer to enhance charge transfer in porous polymer networks for photocatalytic hydrogen evolution. Angew Chem. 2024;136(14):e202319395. doi:10.1002/ange.v136.14. [Google Scholar] [CrossRef]
101. Liu H, Wang Y, Xue X, Liu Y, Chen P, Wang P, et al. Local weak hydrogen bonds induced dipole-dipole interactions in polymer for enhancing photocatalytic oxidation. J Coll Interface Sci. 2024;669:393–401. doi:10.1016/j.jcis.2024.04.221. [Google Scholar] [PubMed] [CrossRef]
102. West J, Jones D, Annunziatellis A, Barlow T, Beaubien SE, Bond A, et al. Comparison of the impacts of elevated CO2 soil gas concentrations on selected European terrestrial environments. 2015;42:357–71. [Google Scholar]
103. Gao H, Liu K, Luo T. CO2 reduction reaction pathways on single-atom Co sites: impacts of local coordination environment. Chin J Catal. 2022;43(3):832–8. doi:10.1016/S1872-2067(21)63893-7. [Google Scholar] [CrossRef]
104. Wang L, Zhang S, Zhang L, Yu J. Regulating the d-band center of Cu nanoparticles for efficient photo-driven catalytic CO2 reduction. Appl Catal B Environ Energy. 2024;355:124167. doi:10.1016/j.apcatb.2024.124167. [Google Scholar] [CrossRef]
105. Hoque KA, Sathi SA, Akter F, Akter T, Ahmed T, Ullah W, et al. Recent advances on photocatalytic CO2 reduction using CeO2-based photocatalysts: a review. J Environ Chem Eng. 2024;12(5):113487. doi:10.1016/j.jece.2024.113487. [Google Scholar] [CrossRef]
106. Zia J, Riaz U. Photocatalytic degradation of water pollutants using conducting polymer-based nanohybrids: a review on recent trends and future prospects. J Mol Liq. 2021;340:117162. doi:10.1016/j.molliq.2021.117162. [Google Scholar] [CrossRef]
107. Alowakennu M, Omoniyi AO, Ejeromedoghene O, Alli YA, Akor E, Nnyia MO. Harnessing photo-induced processes for the fabrication and application of functional conjugated and conducting polymer-based materials. J Mol Struct. 2023;1292:136149. doi:10.1016/j.molstruc.2023.136149. [Google Scholar] [CrossRef]
108. Liao G, He Y, Wang H, Fang B, Tsubaki N, Li C. Carbon neutrality enabled by structure-tailored zeolite-based nanomaterials. Device. 2023;1(5):100173. doi:10.1016/j.device.2023.100173. [Google Scholar] [CrossRef]
109. Aggarwal M, Basu S, Shetti NP, Nadagouda MN, Kwon EE, Park Y-K, et al. Photocatalytic carbon dioxide reduction: exploring the role of ultrathin 2D graphitic carbon nitride (g-C3N4). Chem Eng J. 2021;425:131402. doi:10.1016/j.cej.2021.131402. [Google Scholar] [CrossRef]
110. Li F, Zhang L, Tong J, Liu Y, Xu S, Cao Y, et al. Photocatalytic CO2 conversion to methanol by Cu2O/graphene/TNA heterostructure catalyst in a visible-light-driven dual-chamber reactor. Nano Energy. 2016;27:320–9. doi:10.1016/j.nanoen.2016.06.056. [Google Scholar] [CrossRef]
111. Liu H, Zhang Z, Meng J, Zhang J. Novel visible-light-driven CdIn2S4/mesoporous g-C3N4 hybrids for efficient photocatalytic reduction of CO2 to methanol. Mol Catal. 2017;430:9–19. doi:10.1016/j.molcata.2016.12.006. [Google Scholar] [CrossRef]
112. Yan S, Wang L, Wu Y, Hou T, Li Y, Shen K, et al. Photothermal-enabled metal-free polyfuran photocatalysts for efficient solar-to-hydrogen peroxide energy conversion from seawater. Appl Catal B Environ Energy. 2024;357:124337. doi:10.1016/j.apcatb.2024.124337. [Google Scholar] [CrossRef]
113. Yang J, Yin H, Du A, Tebyetekerwa M, Bie C, Wang Z, et al. Unveiling O2 adsorption on non-metallic active site for selective photocatalytic H2O2 production. Appl Catal B Environ Energy. 2025;361:124586. doi:10.1016/j.apcatb.2024.124586. [Google Scholar] [CrossRef]
114. Armaković SJ, Armaković S, Savanović MM. Photocatalytic application of polymers in removing pharmaceuticals from water: a comprehensive review. Catalysts. 2024;14(7):447. doi:10.3390/catal14070447. [Google Scholar] [CrossRef]
115. Ma L, Gao Y, Wei B, Huang L, Zhang N, Weng Q, et al. Visible-light photocatalytic H2O2 production boosted by frustrated lewis pairs in defected polymeric carbon nitride nanosheets. ACS Catal. 2024;14(4):2775–86. doi:10.1021/acscatal.3c05360. [Google Scholar] [CrossRef]
116. Khizer MR, Saddique Z, Imran M, Javaid A, Latif S, Mantzavinos D, et al. Polymer and graphitic carbon nitride based nanohybrids for the photocatalytic degradation of pharmaceuticals in wastewater treatment—a review. Sep Purif Technol. 2024;350:127768. doi:10.1016/j.seppur.2024.127768. [Google Scholar] [CrossRef]
117. Helali S, Polo-López MI, Fernández-Ibáñez B, Ohtani FA, Malato S, et al. Solar photocatalysis: a green technology for E. coli contaminated water disinfection. Effect of concentration and different types of suspended catalyst. J Photoch Photobio A. 2014;276:31–40. [Google Scholar]
118. Huang Z, Liu J, Liu Y, Xu Y, Li R, Hong H, et al. Enhanced permeability and antifouling performance of polyether sulfone (PES) membrane via elevating magnetic Ni@MXene nanoparticles to upper layer in phase inversion process. J Membr Sci. 2021;623:119080. doi:10.1016/j.memsci.2021.119080. [Google Scholar] [CrossRef]
119. Ghali M, Brahmi C, Benltifa M, Vaulot C, Airoudj A, Fioux P, et al. Characterization of polyoxometalate/polymer photo-composites: a toolbox for the photodegradation of organic pollutants. J Polym Sci. 2021;59(2):153–69. doi:10.1002/pola.v59.2. [Google Scholar] [CrossRef]
120. Benltifa M, Brahmi C, Dumur F, Limousy L, Bousselmi L, Lalevée J. A comparison study of the photocatalytic efficiency of different developed photocatalysts/polymer composites. Eur Polym J. 2022;181:111660. doi:10.1016/j.eurpolymj.2022.111660. [Google Scholar] [CrossRef]
121. Chaturvedi G, Kaur A, Umar A, Khan MA, Algarni H, Kansal SK. Removal of fluoroquinolone drug, levofloxacin, from aqueous phase over iron based MOFs, MIL-100(Fe). J Solid State Chem. 2020;281:121029. doi:10.1016/j.jssc.2019.121029. [Google Scholar] [CrossRef]
122. Das N, Kandimalla S. Application of perovskites towards remediation of environmental pollutants: an overview: a review on remediation of environmental pollutants using perovskites. Int J Environ Sci Technol. 2017;14:1559–72. doi:10.1007/s13762-016-1233-7. [Google Scholar] [CrossRef]
123. Huang H, Pradhan B, Hofkens J, Roeffaers MB, Steele JA. Solar-driven metal halide perovskite photocatalysis: design, stability, and performance. ACS Energy Lett. 2020;5(4):1107–23. doi:10.1021/acsenergylett.0c00058. [Google Scholar] [CrossRef]
124. Bon V, Senkovska I, Kaskel S. Metal-organic frameworks. Singaapore: Springer; 2019. [Google Scholar]
125. Öhrström L, Noa FMA. Metal-organic frameworks. Washington, DC, USA: ACS; 2021. [Google Scholar]
126. Kaskel S. The chemistry of metal-organic frameworks: synthesis, characterization, and applications. New York: John Wiley & Sons; 2016. [Google Scholar]
127. Nisticò R. A comprehensive study on the applications of clays into advanced technologies, with a particular attention on biomedicine and environmental remediation. Inorganics. 2022;10(3):40. doi:10.3390/inorganics10030040. [Google Scholar] [CrossRef]
128. Batzill M. Fundamental aspects of surface engineering of transition metal oxide photocatalysts. Energy Environ Sci. 2011;4(9):3275–86. doi:10.1039/c1ee01577j. [Google Scholar] [CrossRef]
129. Fresno F, Portela R, Suárez S, Coronado JM. Photocatalytic materials: recent achievements and near future trends. J Mater Chem A. 2014;2(9):2863–84. doi:10.1039/C3TA13793G. [Google Scholar] [CrossRef]
130. Hernández-Alonso MD, Fresno F, Suárez S, Coronado JM. Development of alternative photocatalysts to TiO2: challenges and opportunities. Energy Environ Sci. 2009;2(12):1231–57. doi:10.1039/b907933e. [Google Scholar] [CrossRef]
131. Shukla S, Pandey PC, Narayan RJ. Tunable quantum photoinitiators for radical photopolymerization. Polymers. 2021;13(16):2694. doi:10.3390/polym13162694. [Google Scholar] [PubMed] [CrossRef]
132. Shu A, Qin C, Li M, Zhao L, Shangguan Z, Shu Z, et al. Electric effects reinforce charge carriers behaviour for photocatalysis. Energy Environ Sci. 2024;17:4907–28. doi:10.1039/D4EE01379D. [Google Scholar] [CrossRef]
133. Zha J-W, Zheng M-S, Fan B-H, Dang Z-M. Polymer-based dielectrics with high permittivity for electric energy storage: a review. Nano Energy. 2021;89:106438. doi:10.1016/j.nanoen.2021.106438. [Google Scholar] [CrossRef]
134. Li G, Fu P, Yue Q, Ma F, Zhao X, Dong S, et al. Boosting exciton dissociation by regulating dielectric constant in covalent organic framework for photocatalysis. Chem Catal. 2022;2(7):1734–47. doi:10.1016/j.checat.2022.05.002. [Google Scholar] [CrossRef]
135. Amorim SM, Steffen G, de S Junior JM, Brusamarello CZ, Romio AP, Domenico MD. Synthesis, characterization, and application of polypyrrole/TiO2 composites in photocatalytic processes: a review. Polym Compos. 2021;29(7):1055–74. doi:10.1177/0967391120949489. [Google Scholar] [CrossRef]
136. Poonia K, Raizada P, Singh A, Verma N, Ahamad T, Alshehri SM, et al. Magnetic molecularly imprinted polymer photocatalysts: synthesis, applications and future perspective. J Ind Eng Chem. 2022;113:1–14. doi:10.1016/j.jiec.2022.05.029. [Google Scholar] [CrossRef]
137. Wang L, Yu H, Ullah RS, Haroon M, Fahad S, Li J, et al. Recent progress in the electron paramagnetic resonance study of polymers. Polym Chem. 2018;9(24):3306–35. doi:10.1039/C8PY00689J. [Google Scholar] [CrossRef]
138. Li X, Wang W, Dong F, Zhang Z, Han L, Luo X, et al. Recent advances in noncontact external-field-assisted photocatalysis: from fundamentals to applications. ACS Catal. 2021;11(8):4739–69. doi:10.1021/acscatal.0c05354. [Google Scholar] [CrossRef]
139. Zulkifli FZA, Ito M, Uno T, Kubo M. Synthesis and photocatalytic activity of novel polycyclopentadithiophene. Polymers. 2023;15(20):4091. doi:10.3390/polym15204091. [Google Scholar] [PubMed] [CrossRef]
140. Hussain A, Rehman F, Rafeeq H, Waqas M, Asghar A, Afsheen N, et al. In-situ, ex-situ, and nano-remediation strategies to treat polluted soil, water, and air—a review. Chemosphere. 2022;289:133252. doi:10.1016/j.chemosphere.2021.133252. [Google Scholar] [PubMed] [CrossRef]
141. Bhatnagar S, Kumari R. Bioremediation: a sustainable tool for environmental management—a review. Annu Res Rev Biol. 2013;3(4):974–93. [Google Scholar]
142. Linley S, Thomson N. Environmental applications of nanotechnology: nano-enabled remediation processes in water, soil and air treatment. Water Air Soil Pollut. 2021;232(2):59. doi:10.1007/s11270-021-04985-9. [Google Scholar] [CrossRef]
143. Guerra FD, Attia MF, Whitehead DC, Alexis F. Nanotechnology for environmental remediation: materials and applications. Molecules. 2018;23(7):1760. doi:10.3390/molecules23071760. [Google Scholar] [PubMed] [CrossRef]
144. Deng Y, Zhao R. Advanced oxidation processes (AOPs) in wastewater treatment. Curr Pollut Rep. 2015;1:167–76. doi:10.1007/s40726-015-0015-z. [Google Scholar] [CrossRef]
145. Cameselle C, Gouveia S. Electrokinetic remediation for the removal of organic contaminants in soils. Curr Opin Electrochem. 2018;11:41–7. doi:10.1016/j.coelec.2018.07.005. [Google Scholar] [CrossRef]
146. Wang Y, Li A, Cui C. Remediation of heavy metal-contaminated soils by electrokinetic technology: mechanisms and applicability. Chemosphere. 2021;265:129071. doi:10.1016/j.chemosphere.2020.129071. [Google Scholar] [PubMed] [CrossRef]
147. Dahiya S, Mishra B. Enhancing understandability and performance of flow electrode capacitive deionisation by optimizing configurational and operational parameters: a review on recent progress. Sep Purif Technol. 2020;240:116660. doi:10.1016/j.seppur.2020.116660. [Google Scholar] [CrossRef]
148. Lo M, Ktari N, Gningue-Sall D, Madani A, Aaron SE, Aaron J-J, et al. Polypyrrole: a reactive and functional conductive polymer for the selective electrochemical detection of heavy metals in water. Emergent Mater. 2020;3:815–39. doi:10.1007/s42247-020-00119-9. [Google Scholar] [CrossRef]
149. Denham ME, Amidon MB, Wainwright HM, Dafflon B, Ajo-Franklin J, Eddy-Dilek CA. Improving long-term monitoring of contaminated groundwater at sites where attenuation-based remedies are deployed. Environ Manage. 2020;66(6):1142–61. doi:10.1007/s00267-020-01376-4. [Google Scholar] [PubMed] [CrossRef]
150. Gruiz K. Natural attenuation in contaminated soil remediation. Engin Tools Environ Risk Mgt. 2019;4:95–201. [Google Scholar]
151. Liu L, Liu C, Fu R, Nie F, Zuo W, Tian Y, et al. Full-chain analysis on emerging contaminants in soil: source, migration and remediation. Chemosphere. 2024;363:142854. doi:10.1016/j.chemosphere.2024.142854. [Google Scholar] [PubMed] [CrossRef]
152. Pac TJ, Baldock J, Brodie B, Byrd J, Gil B, Morris KA, et al. In situ chemical oxidation: lessons learned at multiple sites. Remediation. 2019;29(2):75–91. doi:10.1002/rem.2019.29.issue-2. [Google Scholar] [CrossRef]
153. Li W, Sohail M, Anwar U, Taha T, Al-Sehemi AG, Muhammad S, et al. Recent progress in g-C3N4–based materials for remarkable photocatalytic sustainable energy. Int J Hydrogen Energy. 2022;47(49):21067–118. doi:10.1016/j.ijhydene.2022.04.247. [Google Scholar] [CrossRef]
154. Partho AT, Tahir M, Tahir B. Recent advances in covalent organic framework (COF) nanotextures with band engineering for stimulating solar hydrogen production: a comprehensive review. Int J Hydrogen Energy. 2022;47(81):34323–75. doi:10.1016/j.ijhydene.2022.08.060. [Google Scholar] [CrossRef]
155. Bushuyev OS, De Luna P, Dinh CT, Tao L, Saur G, Van de Lagemaat J, et al. What should we make with CO2 and how can we make it? Joule. 2018;2(5):825–32. doi:10.1016/j.joule.2017.09.003. [Google Scholar] [CrossRef]
156. Coronado JM, Fresno F, Hernández-Alonso MD, Portela R. Design of advanced photocatalytic materials for energy and environmental applications. London: Springer; 2013. [Google Scholar]
157. Liu C, Wang K, Gong X, Heeger AJ. Low bandgap semiconducting polymers for polymeric photovoltaics. Chem Soc Rev. 2016;45(17):4825–46. doi:10.1039/C5CS00650C. [Google Scholar] [PubMed] [CrossRef]
158. Hassaan MA, El-Nemr MA, Elkatory MR, Ragab S, Niculescu V-C, El Nemr A. Principles of photocatalysts and their different applications: a review. Top Curr Chem. 2023;381(6):31. doi:10.1007/s41061-023-00444-7. [Google Scholar] [PubMed] [CrossRef]
159. Jaiswal KK, Chowdhury CR, Yadav D, Verma R, Dutta S, Jaiswal KS, et al. Renewable and sustainable clean energy development and impact on social, economic, and environmental health. Energy Nexus. 2022;7:100118. doi:10.1016/j.nexus.2022.100118. [Google Scholar] [CrossRef]
160. Lange J-P. Towards circular carbo-chemicals-the metamorphosis of petrochemicals. Energy Environ Sci. 2021;14(8):4358–76. doi:10.1039/D1EE00532D. [Google Scholar] [CrossRef]
161. Harrison RM, Allan J, Carruthers D, Heal MR, Lewis AC, Marner B, et al. Non-exhaust vehicle emissions of particulate matter and VOC from road traffic: a review. Atmos Environ. 2021;262:118592. doi:10.1016/j.atmosenv.2021.118592. [Google Scholar] [CrossRef]
162. Al-Nuaim MA, Alwasiti AA, Shnain ZY. The photocatalytic process in the treatment of polluted water. Chem Pap. 2023;77(2):677–701. doi:10.1007/s11696-022-02468-7. [Google Scholar] [PubMed] [CrossRef]
163. Al-Gamal AQ, Falath WS, Saleh TA. Enhanced efficiency of polyamide membranes by incorporating TiO2-Graphene oxide for water purification. J Mol Liq. 2021;323:114922. doi:10.1016/j.molliq.2020.114922. [Google Scholar] [CrossRef]
164. Akhil D, Lakshmi D, Kartik A, Vo D-VN, Arun J, Gopinath KP. Production, characterization, activation and environmental applications of engineered biochar: a review. Environ Chem Lett. 2021;19:2261–97. doi:10.1007/s10311-020-01167-7. [Google Scholar] [CrossRef]
165. Sasikala V, Karthik P, Ravichandran S, Prakash N, Rajesh J, Mukkannan A. Effective removal of organic dyes using novel MnWO4 incorporated CA/PCL nanocomposite membranes. Surf Interfaces. 2023;40:103008. doi:10.1016/j.surfin.2023.103008. [Google Scholar] [CrossRef]
166. Tran VV, Nu TTV, Jung H-R, Chang M. Advanced photocatalysts based on conducting polymer/metal oxide composites for environmental applications. Polymers. 2021;13(18):3031. doi:10.3390/polym13183031. [Google Scholar] [PubMed] [CrossRef]
167. Dutta V, Sharma S, Raizada P, Thakur VK, Khan AAP, Saini V, et al. An overview on WO3 based photocatalyst for environmental remediation. J Environ Chem Eng. 2021;9(1):105018. doi:10.1016/j.jece.2020.105018. [Google Scholar] [CrossRef]
168. Noureen L, Wang Q, Humayun M, Shah WA, Xu Q, Wang X. Recent advances in structural engineering of photocatalysts for environmental remediation. Environ Res. 2023;219:115084. doi:10.1016/j.envres.2022.115084. [Google Scholar] [PubMed] [CrossRef]
169. Zhang M, Yang Y, An X, Hou L. A critical review of g-C3N4-based photocatalytic membrane for water purification. Chem Eng J. 2021;412:128663. doi:10.1016/j.cej.2021.128663. [Google Scholar] [CrossRef]
170. Ali S, Humayun M, Pi W, Yuan Y, Wang M, Khan A, et al. Fabrication of BiFeO3-g-C3N4-WO3 Z-scheme heterojunction as highly efficient visible-light photocatalyst for water reduction and 2, 4-dichlorophenol degradation: insight mechanism. J Hazard Mater. 2020;397:122708. doi:10.1016/j.jhazmat.2020.122708. [Google Scholar] [PubMed] [CrossRef]
171. Liang Z, Yan C-F, Rtimi S, Bandara J. Piezoelectric materials for catalytic/photocatalytic removal of pollutants: recent advances and outlook. Appl Catal B: Environ. 2019;241:256–69. doi:10.1016/j.apcatb.2018.09.028. [Google Scholar] [CrossRef]
172. Wang H, Li X, Zhao X, Li C, Song X, Zhang P, et al. A review on heterogeneous photocatalysis for environmental remediation: from semiconductors to modification strategies. Chin J Catal. 2022;43(2):178–214. doi:10.1016/S1872-2067(21)63910-4. [Google Scholar] [CrossRef]
173. Djurišić AB, He Y, Ng A. Visible-light photocatalysts: prospects and challenges. APL Mater. 2020;8(3):030903. doi:10.1063/1.5140497. [Google Scholar] [CrossRef]
Cite This Article
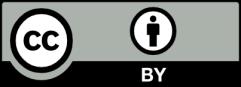
This work is licensed under a Creative Commons Attribution 4.0 International License , which permits unrestricted use, distribution, and reproduction in any medium, provided the original work is properly cited.