Open Access
ARTICLE
Constructing TiO2/g-C3N4/Single-Walled Carbon Nanotube Hydrogel for Synergistic Solar Evaporation and Photocatalytic Organic Pollutant
1 Jiangxi Provincial Key Laboratory of Flexible Electronics, Jiangxi Science and Technology Normal University/Nanchang Jiaotong Institute, Nanchang, 330013, China
2 School of Pharmacy, Jiangxi Science and Technology Normal University, Nanchang, 330013, China
3 School of Chemistry and Chemical Engineering, Jiangxi Science and Technology Normal University, Nanchang, 330013, China
4 Centre for Analysis and Testing, Jiangxi Science and Technology Normal University, Nanchang, 330013, China
* Corresponding Author: Sanmei Liu. Email:
(This article belongs to the Special Issue: Multifunctional Conductive Hydrogels and Their Applications)
Journal of Polymer Materials 2024, 41(4), 315-327. https://doi.org/10.32604/jpm.2024.057951
Received 31 August 2024; Accepted 28 October 2024; Issue published 16 December 2024
Abstract
Integration of solar-driven interfacial evaporation and photocatalysis is one of the most promising technologies for generating fresh water and removing pollutants. In this work, TiO2/g-C3N4 photocatalysis is loaded on a hydrogel containing single-walled carbon nanotube (SWCNT). Due to the excellent water evaporation channel of hydrogel and the excellent photothermal conversion performance of SWCNT, as well as the good visible light absorption ability of TiO2/g-C3N4, TiO2/g-C3N4/SWCNT hydrogel exhibits good hydrothermal evaporation and photocatalytic activity. The optimum water evaporation rate of 1.43 kg m−2 h−1. In particular, the optimized TiO2/g-C3N4/SWCNT hydrogel can also remove more than 90% methylene blue (MB) within 120 min. Moreover, the TiO2/g-C3N4/SWCNT hydrogel shows good photocatalytic stability after five cycles. Furthermore, the radical trapping experiment is also performed. The photogenerated ·O2− and ·OH− plays a critical role in MB degradation. This work will provide guidance for the rational design of multifunctional photothermal materials. TiO2/g-C3N4/SWCNT hydrogel presents a promising methodology to boost practical applications for seawater desalination and sewage purification.Graphic Abstract
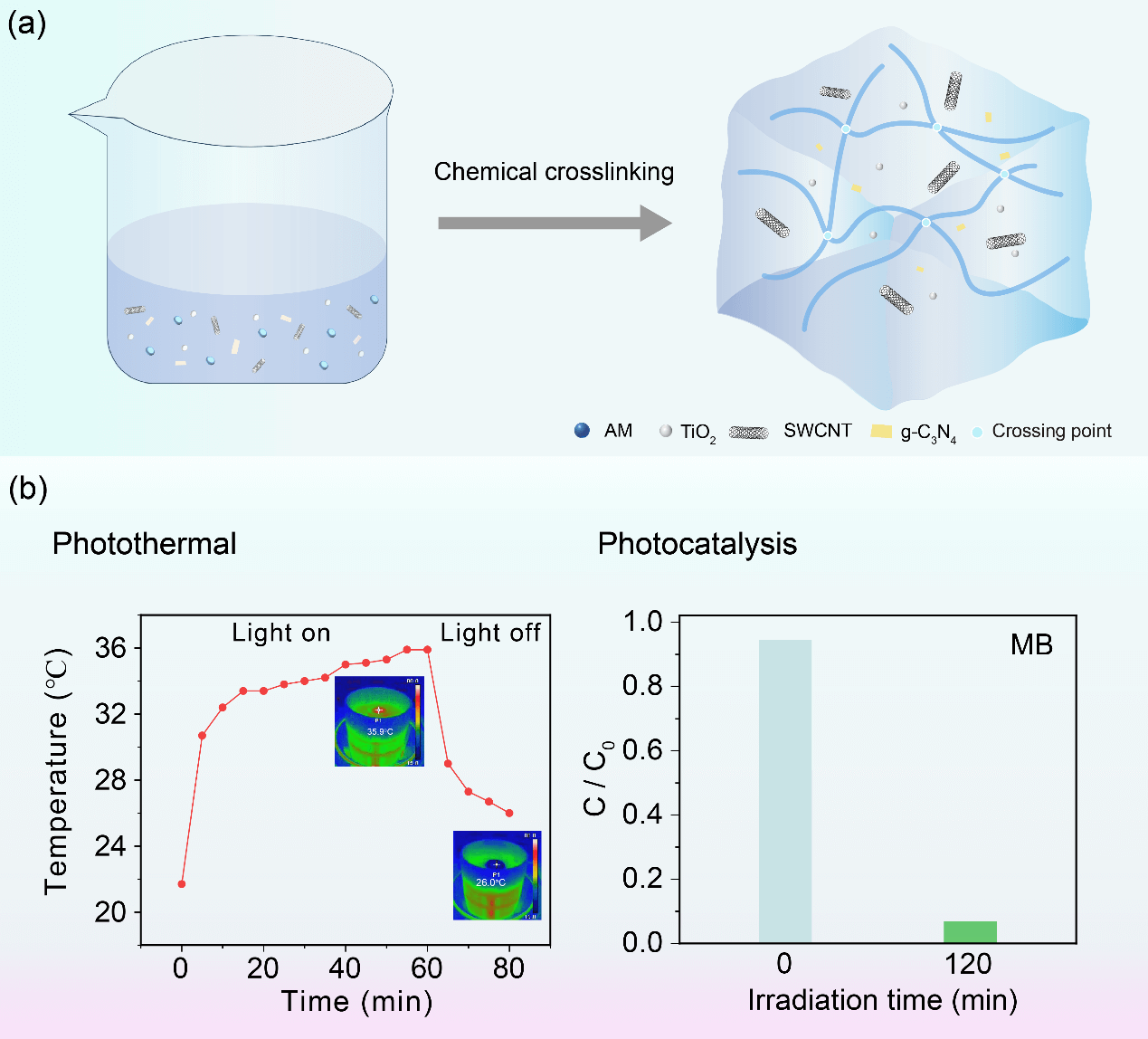
Keywords
Supplementary Material
Supplementary Material FileThe rapid population growth, worsening pollution, and increasingly severe weather have exacerbated the global water and energy crises. Currently, the shortage of freshwater resources worldwide poses a severe threat to the stability of ecosystems and human health [1–3]. Many people, especially those in remote and rural areas, lack the infrastructure needed to consistently access safe drinking water. Consequently, developing cost-effective wastewater treatment technologies has been a focus of research. Utilizing renewable solar energy through interfacial solar steam evaporation technology has shown great potential for seawater desalination [4–7] and wastewater treatment [8–11]. Although solar photothermal technology offers promising solutions for wastewater issues, traditional evaporators struggle to efficiently treat wastewater. To address this, researchers have combined photothermal evaporators with photocatalysts to achieve both efficient water evaporation and wastewater treatment. For example, Li et al. [12] developed an Ag/MXene membrane that leverages photothermal and photocatalytic synergy to degrade heavy metal ions and organic pollutants during wastewater treatment; Miao et al. [13] designed a metal-organic frameworks (MOFs)-derived C/TiO2 composite material that simultaneously performs photothermal and photocatalytic functions, enabling solar-driven freshwater production and degradation of organic pollutants in wastewater. As research progresses, photothermal-photocatalytic materials have been demonstrated to degrade volatile organic compounds (VOCs) in distilled water [14]. Additionally, solar-driven photocatalytic evaporators offer the potential for multifunctional applications, achieving dual [15] or even triple benefits [16].
Owing to their three-dimensional network structures with activated water states, excellent mechanical/optical/electronic properties, and multi-scale tunability, hydrogels have gained widespread applications in various fields such as bioelectronics [17–19], biomedical engineering [20], energy conversion [21], strain sensors [22], electrochromics [23], soft robotics [24,25], etc. Particularly, various hydrogel-based evaporators have also been developed for water purification in recent years [6,8,20,21]. For example, Wang et al. [14] developed an MXene/CdS photothermal-photocatalytic hydrogel for efficient solar water evaporation and VOC degradation. Zhao et al. [26] designed a galactomannan/graphene oxide/Fe3O4 hydrogel evaporator for solar-driven water evaporation and simultaneous photothermal power generation. Zheng et al. [27] created a magnetically controlled, wind-resistant Janus biomass composite hydrogel with desalination capabilities for the purification of polluted seawater. Lyu et al. [28] developed a simple, low-cost macroporous hydrogel for high-performance atmospheric water harvesting (AWH). Therefore, hydrogels integrating photothermal and photocatalytic effects hold significant potential in this field.
TiO2, due to its low cost and environmental friendliness, is a potential photocatalyst. However, it can only absorb ultraviolet light, and the rapid recombination of photogenerated carriers significantly reduces its photocatalytic activity [29–31]. To overcome these drawbacks, constructing heterojunctions is an ideal strategy to mitigate the rapid recombination of photogenerated carriers. Since g-C3N4 exhibits good visible light absorption, combining TiO2 with g-C3N4 can effectively reduce photogenerated carrier recombination [32–35]. There are relevant studies on this topic, such as the work by Yu et al. [36], who developed an advanced 2D/3D g-C3N4/TiO2@MnO2 multifunctional separation membrane for light-driven sustainable water purification. Yang et al. [37] designed a hollow octahedral MOF-derived TiO2 structure combined with ultrathin porous g-C3N4, enhancing the degradation efficiency of brewing wastewater.
Given the above analysis, this paper demonstrates that TiO2/g-C3N4 loaded on a hydrogel matrix can achieve efficient solar-driven evaporation and organic pollutant degradation. By incorporating SWCNTs with broad-spectrum absorption and TiO2/g-C3N4 photocatalytic heterojunction, a hydrogel with dual functionalities of photocatalytic degradation and interfacial evaporation is developed. The prepared TiO2/g-C3N4/SWCNT composite hydrogel exhibited excellent light absorption across the full spectrum (approximately 98.9%), efficient photothermal conversion (35.9°C), and a water evaporation rate of 1.43 kg m−2 h−1. Notably, the hydrogel exhibited a lower evaporation enthalpy than pure water (as low as 1144.7 J g−1). The photocatalytic performance of the hydrogel enabled over 90% degradation of pollutants within 120 min, with almost no decline in photocatalytic performance after five cycles. The primary active species for methylene blue (MB) photodegradation are ⋅O2− and ⋅OH−.
Materials: N,N’-Methylenebis (acrylamide) (MBAA, 99%), ammonium persulfate (APS, ≥98%), acrylamide (AM, 99.0%), melamine (99%), and titanium oxide (TiO2, 98%) were obtained from Aladdin (Shanghai, China). N,N,N’,N’-Tetramethylethylenediamine (TEMED, 99%) was obtained from J&K Scientific (Beijing, China). Single-walled carbon nanotube (SWCNT) aqueous dispersion (9–10 wt.%) was obtained from XFNano (Nanjing, China). All other reagents were analytical grade and directly employed without further purification.
Preparation of graphitic carbon nitride (g-C3N4): g-C3N4 was prepared by direct thermal polymerization. Specifically, 5 g of melamine was placed into a crucible, covered with a lid, and heated in a muffle furnace at a rate of 15°C/min to 550°C. The temperature was maintained for 4 h, and then the sample was cooled to room temperature. After cooling, the product was removed and ground into a powder in a mortar for future use.
Preparation of single catalyst hydrogel: First, 0.3 g of TiO2 nanoparticle/g-C3N4 powder was added to 10 mL of deionized water, stirred thoroughly, and then sonicated for 30 min to form a homogeneous aqueous solution. Subsequently, 0.027 g of a diluted aqueous dispersion of single-walled carbon nanotubes (4.5–5 wt.%) was added and stirred well to form dispersion A. Then, 1.1 g of AM monomer was added to dispersion A, stirred until dissolved, and 100 μL of APS (10 wt.%) initiator solution and 0.0125 g of MBAA crosslinker were added to form solution B. The stirred dispersion of solution B was then sonicated for 10 min to form a homogeneous dispersion. Afterward, 50 μL of TEMED catalyst was added while stirring, and after stirring for 1 min, the mixture was transferred to a mold for gelation. After the gelation was completed at room temperature, the hydrogel was demolded and soaked in deionized water for more than 48 h to remove residual substances. It is noteworthy that the TiO2/SWCNT and g-C3N4/SWCNT hydrogels corresponded to TSH and CSH, respectively.
Preparation of composite catalyst hydrogel: First, 0.3 g of TiO2 nanoparticle and 0.3 g of g-C3N4 powder were added to 10 mL of deionized water, stirred thoroughly, and then sonicated for 30 min to form a homogeneous aqueous solution. Subsequently, 0.027 g of a diluted aqueous dispersion of single-walled carbon nanotubes (4.5–5 wt.%) was added and stirred well to form dispersion A. Then, 1.1 g of AM monomer was added to dispersion A, stirred until dissolved, and 100 μL of APS (10 wt.%) initiator solution and 0.0125 g of MBAA crosslinker were added to form solution B. The stirred dispersion of solution B was then sonicated for 10 min to form a homogeneous dispersion. Afterward, 50 μL of TEMED catalyst was added while stirring, and after stirring for 1 min, the mixture was transferred to a mold for gelation. After the gelation was completed at room temperature, the hydrogel was demolded and soaked in deionized water for more than 48 h to remove residual substances. It is noteworthy that in the TiO2/g-C3N4/SWCNT composite hydrogel, the weight ratios of TiO2 to g-C3N4 were 1:1, 1:2, and 2:1, corresponding to TCSH-1, TCSH-2, and TCSH-3, respectively.
Characterizations: The surface morphology and chemical element distribution of TSH, CSH, and TCSH-1 hydrogels were characterized using using a scanning electron microscope (SEM, SU8600, Hitachi, Tokyo, Japan) equipped with an X-ray energy dispersive spectrometer (EDS, ELECT SUPER(C5), EDAX, Commonwealth of Pennsylvania, US). The TiO2 and g-C3N4 powders were analyzed by X-ray photoelectron spectroscopy using an X-ray diffractometer (XRD, Empyrean, MalvernPanalytical, Malvern, UK). The diffuse reflectance properties of the prepared samples were measured using an ultraviolet-visible-near-infrared spectrophotometer (TP720, Tianjin Toupu Instrument Co., Tianjin, China) with an integrating sphere unit and an automatic reflectance measurement unit, and the obtained spectra were corrected for baseline/blank and black. The absorbance (A) was obtained through the Eq. (1):
A=1−T−R(1)
where R and T are the reflectance and transmittance of the hydrogel, respectively.
Photocatalytic performance measurements: The photocatalytic performance of the hydrogels was evaluated using methylene blue (MB) at a concentration of 10 g L−1 as a model. The light intensity was maintained at one sun (1 kW m−2), with a solar simulator as the light source (CEL-HXUV300-T3, Education Au-light Co., Beijing, China). The prepared 3D columnar hydrogels were cut into small pieces and placed in the methylene blue solution before irradiation, kept in the dark for 60 min to achieve adsorption-desorption equilibrium. Subsequently, samples (3 mL) were taken every 15 min and measured using a UV-Vis-NIR spectrophotometer (TP720, Tianjin Toupu Instrument Co., Tianjin, China). Moreover, the degradation efficiency (De) was calculated based on the Eq. (2) [38,39]:
De=1−C/C0(2)
where C0 denotes the initial concentration, and C is the concentration of the solution in different periods. We further evaluate the photocatalytic efficiency based on the pseudo-first-order model according to the Eq. (3) [40]:
−ln(C/C0)=kt(3)
where k is related to the constant photodegradation rate.
Solar steam generation performance measurements: The solar steam generation performance of the hydrogels was tested using a solar simulator with an AM1.5 filter (CEL-HXUV300-T3, Education Au-light Co., Beijing, China). The solar flux was measured by an automatic optical power meter (CEL-NP2000-2A, Education Au-light Co., Beijing, China). The mass change during the evaporation process was monitored in real-time using an electronic analytical balance (Sartorius BAS223, accurate to 1 mg). The surface temperature and infrared thermograms of the hydrogels were monitored in real-time using an infrared thermal imager (HM-TPH21Pr0-3AQF, HIKMICRO, Hangzhou, China). During the tests, the ambient humidity and temperature were ~60% and ~25°C, respectively. Additionally, the evaporation rate (ER) and equivalent evaporation enthalpy (Eequ) were calculated based on the Eqs. (4) and (5) [41]:
ER=dm/(S∗dt)(4)
where dm denotes to the mass change by using hydrogel as the evaporator for 1 h, S is the evaporation area of hydrogel, and dt is evaporation time.
Eequ=E0m0mg(5)
where E0 and m0 are the evaporation enthalpy and mass change of bulk water; mg is the mass change of hydrogel.
Conductivity and salinity measurements: In the conductivity range of 0.001 to 0.01 wt.‰, the salinity of NaCl solutions exhibits a strong linear correlation with electrical conductivity. A standard curve was established by testing the conductivity of different brines with a conductivity controller (A10CD-AA1, Shanghai Kuosi Electronics Co., Shanghai, China) and used to calculate the salinity of pure water evaporated from simulated seawater by TCSH-1. The conductivity of at least three parallel samples is measured and averaged to accurately determine the salinity.
As shown in Fig. 1a, the hydrogel prepared in the experiment has a three-dimensional columnar structure, designed to increase the evaporation area and thereby enhance the evaporation rate of the hydrogel. TiO2 nanoparticles and g-C3N4 nanosheet dual catalysts are integrated into the hydrogel matrix. The characteristic macroporous structure of the hydrogel not only enables multi-level refraction and diffraction of light within the gel network, thereby enhancing light absorption, but also allows for rapid water absorption and transport (Fig. 1b). In addition, this design specifically addresses the drawback of TiO2, which can only absorb ultraviolet light, and its reduced photocatalytic efficiency due to rapid photogenerated carrier recombination. Since g-C3N4 has well visible light absorption, the heterojunction constructed by the dual catalysts can improve the efficiency of the photocatalyst (Fig. 1c). The incorporation of photocatalytic functionality not only enables efficient sewage purification but also prevents the contamination of bulk water during the evaporation process. Such hydrogels hold promise for multifunctional applications in solar-powered water purification systems.
Figure 1: Schematic diagram of hydrogel evaporation and photocatalysis. (a) Schematic diagram of TiO2/g-C3N4/SWCNT (TCSH-1) and its advantages in solar-driven water purification: photothermal evaporation and photocatalytic degradation. (b) Schematic diagram of the porous structure of the hydrogel, illustrating the multi-level refraction and diffraction of light within the gel network, as well as the rapid absorption of water from the bottom towards the top. (c) Schematic diagram of the mechanism of TCSH-1 photocatalytic degradation of MB
TiO2 and g-C3N4, as typical photocatalysts, are now widely applied. In this study, g-C3N4 powder is synthesized by a direct thermal polymerization method, resulting in a pale yellow color. The microstructure and crystal structure of the two solid catalysts are further explored through Scanning Electron Microscopy (SEM) and X-ray Diffraction (XRD). As shown in Fig. S1, the TiO2 and g-C3N4 solids exhibit different morphological structures at 2 μm. The purchased TiO2 appears as nanoparticles under microscopic observation, while the g-C3N4 obtained by thermal polymerization exhibits a sheet-like structure. Further crystal structure analysis is performed using XRD. As shown in Fig. S2, the diffraction peaks at 2θ = 13.5° and 27.4° correspond to the (100) and (002) crystal planes of the g-C3N4 standard sample card (JCPDS 87-1526), respectively. The diffraction peak at 13.5° is attributed to the tris-s-triazine rings, and the sharp diffraction peak at 27.4° is caused by the stacking of aromatic heterocycles. This indicates that g-C3N4 is successfully prepared by the direct thermal polymerization method and that the prepared g-C3N4 possesses good purity and crystallinity. Subsequently, the samples of TiO2/g-C3N4 loaded on the hydrogel matrix are analyzed using SEM and Energy Dispersive X-ray Spectroscopy (EDS). The results show that the photocatalyst is successfully loaded in the hydrogel, displaying the characteristic porous structure of the hydrogel (Fig. 2).
Figure 2: Microstructure and elemental distribution. SEM images of (a) TSH, (b) CSH, (c) TCSH-1, (d) elemental mapping images of C, (e) N, (f) O, and (g) Ti
Excellent light absorption across the full spectrum is essential for achieving efficient photothermal conversion and solar-driven evaporation performance in hydrogels. As shown in Fig. 3a, the prepared single and composite photocatalytic hydrogels exhibit superior light absorption across the entire spectrum (with an absorption rate greater than 98.8%), with negligible light loss. Notably, the light absorption rate of TSH reaches 99.9%, outperforming CSH and TCSH-1. The primary reason for this is that the reflectivity of the TCSH-1 hydrogel across the full spectrum is higher than that of the other two single-catalyst hydrogels (Fig. 3b). Furthermore, we investigate the wetting properties of TSH, CSH, and TCSH-1 through water contact angle measurements. The contact angle of the TCSH-1 hydrogel rapidly decreases to 12.87°, facilitating the dynamic migration of water molecules to the evaporator surface (Fig. S3). As depicted in Fig. 3d,e, the water evaporation performance of TSH, CSH, and TCSH-1 is evaluated by recording the mass change of water under 1 kW m−2 solar irradiation over 60 min. As the irradiation time increases, the mass change of water in each hydrogel exhibits a linear increase, with the CSH sample showing the highest evaporation rate, approximately 1.63 kg m−2 h−1. The water evaporation rate of the prepared TCSH-1 shows a decreasing trend with the increase in the number of photocatalysts in the hydrogel components. Furthermore, the different evaporation rates of the hydrogels are verified by testing the equivalent enthalpy of evaporation. Specifically, the evaporation rate is recorded by placing the hydrogels under dark conditions, and the corresponding equivalent enthalpy of evaporation is calculated using Eq. (5). The results indicate that the obtained enthalpy of evaporation correlates well with the hydrogel evaporation rate results, with CSH having the lowest equivalent enthalpy of evaporation, at 1144.7 J g−1 (Fig. 3c). Notably, the equivalent enthalpy of evaporation of the prepared hydrogels is significantly lower than that of pure water (2450 J g−1). The photothermal conversion efficiency of these hydrogels is further demonstrated by monitoring the interface temperature changes of the hydrogels under illumination using infrared imaging technology. Remarkably, the temperature of the hydrogels rapidly rises above 30°C within the first 10 min of light exposure, followed by a slow increase to 35.9°C. The rapid decrease in surface temperature in the absence of light further confirms the high photothermal conversion capability of the hydrogels (Fig. 3f,g).
Figure 3: The light absorption and photothermal conversion properties of hydrogels. The absorbance (a) and reflectance (b) of TSH, CSH, and TCSH-1 hydrogels across the full spectral range. (c) The relationship between the equivalent evaporation enthalpy and the evaporation rate of TSH, CSH, and TCSH-1 hydrogels. (d) The change in water mass over time for TSH, CSH, and TCSH-1 hydrogels under one sun illumination, with corresponding evaporation rate (e) and temperature response (f). (g) The evaporation experiment using CSH floating on bulk water, is conducted at irradiation times of 0, 10, 20, 40, 60, and 80 min, with corresponding photographs and infrared images
The long-term stability of materials is essential for ensuring the sustainable and efficient operation of water purification processes. To evaluate the stability of the TCSH-1 hydrogel, it is subjected to a cyclic test in 3.5 wt.% simulated seawater for seven consecutive cycles (Fig. 4a). After repeated swelling-evaporation processes, the evaporation performance of the hydrogel remains consistently at 2.03 kg m−2 h−1. Furthermore, the evaporation performance of the TCSH-1 hydrogel evaporator is tested under outdoor conditions to assess its practical performance in a real-world environment. As shown in Fig. S4, a fully automatic optical power meter records the solar power density in Nanchang from 9:00 a.m. to 16:00 p.m. on 23rd September. The weather on that day is cloudy to overcast, with a light breeze (wind speed 3–4 level), and the hourly evaporation rates are displayed in Fig. 4b.
Figure 4: The practical application performance of hydrogels. (a) The TCSH-1 hydrogel undergoes continuous evaporation testing in 3.5 wt.% simulated seawater. (b) The evaporation performance of the hydrogel evaporator is evaluated under outdoor conditions. (c) Salinity of the simulated seawater samples is measured before and after desalination. (d) The concentrations of typical salt cations (Na+, Mg2+, K+, and Ca2+) in the seawater samples are analyzed before and after purification
Next, the performance of the hydrogel in seawater desalination and wastewater purification is evaluated. Three different simulated seawater samples—World Sea (average salinity 35 wt.‰), Bohai Sea (moderate salinity, 70 wt.‰), and Dead Sea (highest salinity, 200 wt.‰)—are purified. After purification, the salinity of these samples decreases by 3 to 4 orders of magnitude, as shown in Figs. 4c and S5. Additionally, the purified water meets the drinking water standards of the World Health Organization (WHO, 1 wt.‰) and the Environmental Protection Agency (EPA, 0.5 wt.‰). Inductively coupled plasma mass spectrometry (ICP-MS) is used to measure the concentrations of salt ions, including Na+, Mg2+, K+, and Ca2+, in the evaporated water. As depicted in Fig. 4d, the concentrations of major salt ions decrease by more than three orders of magnitude after purification, demonstrating the high desalination efficiency of TCSH-1.
The photocatalytic efficiency of the prepared hydrogel is evaluated through the photodegradation test of a typical organic pollutant, methylene blue (MB). First, the hydrogel is placed under dark conditions for 60 min to achieve adsorption-desorption equilibrium, followed by a 120-min photocatalytic degradation test of MB. The results indicate that TCSH-1 exhibits the highest degradation efficiency (Fig. 5a). As shown in Fig. 5b, kinetic analysis reveals that the rate constants (k values) for TSH, CSH, TCSH-1, TCSH-2, and TCSH-3 hydrogels are 0.0104, 0.01293, 0.02039, 0.01664, and 0.01311 min−1, respectively. As shown in Table S1, the TCSH-1 hydrogel evaporator exhibits better photothermal evaporation efficiency [42–44]. More notably, TCSH-1 hydrogel is much better than or comparable to those of reported hydrogel based catalysts [45–47]. Therefore, TSH, CSH, and TCSH-1 are selected for subsequent experiments. As shown in Fig. 5c, the UV-visible absorption spectra indicate that the absorption peak of MB at 664 nm significantly decreases over time, suggesting the gradual degradation of MB. As can be seen from Fig. 5d, the catalytic performance of the photocatalyst slightly decreases after five cycles (10 h). Moreover, the SEM morphology of TCSH-1 remains nearly unchanged after five cycles (Fig. S6), indicating that the synthesized TCSH-1 exhibits excellent stability.
Figure 5: Photocatalytic degradation of MB experiment. (a) Degradation percent and (b) kinetic curves of TSH, CSH, TCSH-1, TCSH-2, TCSH-3. Photodegradation MB for (c) Temporal UV-vis adsorption spectra of TCSH-1. (d) Five cycles of the TCSH-1
The mechanism of photodegradation MB for TCSH-1 is further studied in Fig. 6. Radicals and holes trapping experiments are adopted by adding p-benzoquinone (BQ), disodium ethylenediaminetetraacetate (EDTA-2Na) and isopropyl alcohol (IPA), which using as ⋅h+, ⋅O2− and ⋅OH−, respectively (Fig. 6a). As presented in Fig. 6b, a significant effect is observed after adding BQ and IPA for photodegradation activity, implying ⋅O2− and ⋅OH− are dominant active species in photodegradation MB. The optical properties of TSH, CSH, and TCSH-1 are investigated. As seen in Fig. 6c, CSH and TCSH-1 display the absorption edge in visible light. In order to obtain Eg of TiO2 and g-C3N4, their UV-vis diffusion reflection spectra and Tacu plots are provided in Fig. 6d, and the bandgap of the semiconductor catalysts are obtained according to the Eq. (6) [48,49]:
(αhV)n=A(hV−Eg)(6)
Figure 6: Photocatalytic mechanism investigation. (a) Effect of different scavengers for photodegradation MB in the presence of TCSH-1. (b) Histogram of the conversion of TCSH-1. (c) UV-vis diffusion reflection spectra of TSH, CSH, TCSH-1, and (d) TiO2, g-C3N4 (the inset: is corresponding Tacu plots)
The Eg of TiO2 and g-C3N4 were 3.1 and 2.74 eV by the straight line to the X axis intercept, respectively.
To better understand the mechanism of this photodegradation MB process, the conduction and valence band positions of TiO2 and g-C3N4 are estimated according to reported literature [50]. The process of charge separation and transfer in TiO2/g-C3N4/SWCNT hydrogel can be illustrated in Scheme S1 according to the above-mentioned results. The electron-hole pairs are generated and the electrons transferred from the VB to the CB when the TiO2/g-C3N4/SWCNT hydrogel absorbed visible light. Because the ·O2− and ·OH− as the primary active species for MB degradation, a possible Z type heterojunction between TiO2 and g-C3N4 is proposed, the photogenerated electrons of TiO2 can be migrated to VB of g-C3N4, the electrons in CB of g-C3N4 is combined the O2 to produce ·O2− and the hole in VB of TiO2 is combined the H2O to produce ·OH− to degrade MB. Thus, effective separation of the photogenerated electron-hole pairs are achieved.
In summary, TiO2/g-C3N4/SWCNT hydrogel with solar water evaporation and photocatalytic activity is successfully synthesized. The obtained TiO2/g-C3N4/SWCNT hydrogel show well water evaporation channel, photothermal conversion, and charge separation efficiency. Due to these merits, the TiO2/g-C3N4/SWCNT hydrogel demonstrates good water evaporation efficiency and photodegradation performance for MB. Furthermore, the radical trapping experiment is conducted to further understand the mechanism of photodegradation, and the result confirms that the ⋅O2− and ⋅OH− are the main active species for MB degradation. This study is helpful for designing and developing efficient multi-functional photothermal materials.
Acknowledgement: The authors extend gratitude to all individuals who contributed to the completion of this study. The authors express their gratitude to the Analytical Testing Center of Jiangxi Normal University of Science and Technology for providing the equipment.
Funding Statement: This work was financially supported by the Jiangxi Provincal Key Laboratory of Flexible Electronics (20212BCD42004 & 20242BCC32010).
Author Contributions: The authors confirm contribution to the paper as follows: Writing—review & editing, Writing—original draft, Software, Formal analysis, Data curation, Experiment, Conceptualization: Junxiao Qiu; Writing—review & editing, Writing—original draft, Resources, Supervision, Data curation, Conceptualization, Funding acquisition: Sanmei Liu. All authors reviewed the results and approved the final version of the manuscript.
Availability of Data and Materials: The datasets generated during and analyzed during the current study are available from the corresponding author on reasonable request.
Ethics Approval: Not applicable.
Conflicts of Interest: The authors declare that they have no conflicts of interest to report regarding the present study.
Supplementary Materials: Supplementary material is available online at https://doi.org/10.32604/jpm.2024.057951.
References
1. Shannon MA, Bohn PW, Elimelech M, Georgiadis JG, Mariñas BJ, Mayes AM. Science and technology for water purification in the coming decades. Nature. 2008;452(7185):301–10. doi:10.1038/nature06599. [Google Scholar] [PubMed] [CrossRef]
2. Mekonnen MM, Hoekstra AY. Four billion people facing severe water scarcity. Sci Adv. 2016;2(2):e1500323. doi:10.1126/sciadv.1500323. [Google Scholar] [PubMed] [CrossRef]
3. Lorenzo R, Davide DC, Maria CR, Jampel DA, Paolo D. Global agricultural economic water scarcity. Sci Adv. 2020;6(18):eaaz6031. doi:10.1126/sciadv.aaz6031. [Google Scholar] [PubMed] [CrossRef]
4. Service RF. Desalination freshens up. Science. 2006;313(5790):1088–90. doi:10.1126/science.313.5790.1088. [Google Scholar] [PubMed] [CrossRef]
5. Qiu JX, Xu XY, Li Z, Hu YX, Liu GQ, Lv XM, et al. A solar-electric dual-driven microporous hydrogel evaporator for all-weather highly efficient water purification. Nano Energy. 2024;130:110057. doi:10.1016/j.nanoen.2024.110057. [Google Scholar] [CrossRef]
6. Xu XY, Zhao Q, Liu Q, Qiu JX, Li J, Zheng WQ, et al. Full-spectrum-responsive Ti4O7-PVA nanocomposite hydrogel with ultrahigh evaporation rate for efficient solar steam generation. Desalination. 2024;577:117400. doi:10.1016/j.desal.2024.117400. [Google Scholar] [CrossRef]
7. Zhao F, Guo YH, Zhou XG, Shi W, Yu GH. Materials for solar-powered water evaporation. Nat Rev Mater. 2020;5(5):388–401. doi:10.1038/s41578-020-0182-4. [Google Scholar] [CrossRef]
8. Li Z, Qiu JX, Xu XY, Wan RT, Yao MT, Wang HB, et al. Solar driven kaolin-based hydrogels for efficient interfacial evaporation and heavy metal ion adsorption from wastewater. Sep Purif Technol. 2025;354:129243. doi:10.1016/j.seppur.2024.129243. [Google Scholar] [CrossRef]
9. Zhou JH, Gu YF, Liu PF, Wang PF, Miao L, Liu J, et al. Development and evolution of the system structure for highly efficient solar steam generation from zero to three dimensions. Adv Funct Mater. 2019;29(50):1903255. doi:10.1002/adfm.201903255. [Google Scholar] [CrossRef]
10. Zhao Q, Wu ZX, Xu XY, Yang RP, Ma HD, Xu QL, et al. Design of poly(3,4-ethylenedioxythiophenepolystyrene sulfonate-polyacrylamide dual network hydrogel for long-term stable, highly efficient solar steam generation. Sep Purif Technol. 2022;300:121889. doi:10.1016/j.seppur.2022.121889. [Google Scholar] [CrossRef]
11. Zhao Q, Liu JY, Wu ZX, Xu XY, Ma HD, Hou JD, et al. Robust PEDOT: PSS-based hydrogel for highly efficient interfacial solar water purification. Chem Eng J. 2022;442:136284. doi:10.1016/j.cej.2022.136284. [Google Scholar] [CrossRef]
12. Zhang QX, Zhang ZP, Zhao DY, Wang L, Li H, Zhang F, et al. Synergistic photocatalytic-photothermal contribution enhanced by recovered Ag+ ions on MXene membrane for organic pollutant removal. Appl Catal B-Environ. 2023;320:122009. doi:10.1016/j.apcatb.2022.122009. [Google Scholar] [CrossRef]
13. Su LF, Liu X, Xia W, Wu B, Li C, Xu B, et al. Simultaneous photothermal and photocatalytic MOF-derived C/TiO2 composites for high-efficiency solar driven purification of sewage. J Coll Interf Sci. 2023;650:613–21. doi:10.1016/j.jcis.2023.07.014. [Google Scholar] [PubMed] [CrossRef]
14. Wang ZY, Xu L, Liu CH, Han SJ, Fu ML, Yuan BL. MXene/CdS photothermal-photocatalytic hydrogels for efficient solar water evaporation and synergistic degradation of VOC. J Mater Chem A. 2024;12(18):10991–1003. [Google Scholar]
15. Fan DQ, Tang YC, Liao YM, Mi Y, Lu Y, Yang XF. Two birds with one stone: functionalized wood composites for efficient photocatalytic hydrogen production and solar water evaporation. Chin Chem Lett. 2024;35(9):109441. doi:10.1016/j.cclet.2023.109441. [Google Scholar] [CrossRef]
16. Wang HW, Wang ZY, Wang T, Tang WQ, Gao SM, Niu H, et al. Shooting three birds with one stone: device construction and thermal management for simultaneous photothermal conversion water evaporation, thermoelectric generation and photocatalytic degradation. Adv Funct Mater. 2024;34(23):2315211. doi:10.1002/adfm.202315211. [Google Scholar] [CrossRef]
17. Yu JW, Wan RT, Tian FJ, Cao J, Wang W, Liu Q, et al. 3D printing of robust high-performance conducting polymer hydrogel-based electrical bioadhesive interface for soft bioelectronics. Small. 2024;20(19):2308778. doi:10.1002/smll.202308778. [Google Scholar] [PubMed] [CrossRef]
18. Yu JW, Tian FJ, Wang W, Wan RT, Cao J, Chen C, et al. Design of highly conductive, intrinsically stretchable, and 3D printable PEDOT: PSS hydrogels via PSS-chain engineering for bioelectronics. Chem Mater. 2023;35(15):5936–44. doi:10.1021/acs.chemmater.3c00844. [Google Scholar] [CrossRef]
19. Wan RT, Yu JW, Quan ZY, Ma HD, Li JH, Tian FJ, et al. A reusable, healable, and biocompatible PEDOT: PSS hydrogel-based electrical bioadhesive interface for high-resolution electromyography monitoring and time-frequency analysis. Chem Eng J. 2024;490:151454. doi:10.1016/j.cej.2024.151454. [Google Scholar] [CrossRef]
20. Hu Y. Biomedical DNA hydrogels. Soft Sci. 2022;2:3. doi:10.20517/ss.2021.20. [Google Scholar] [CrossRef]
21. Liu C, Wang SJ, Feng SP, Fang NX. Portable green energy out of the blue: hydrogel-based energy conversion devices. Soft Sci. 2023;3:10. [Google Scholar]
22. Zhang ZL, Chen GD, Xue Y, Duan QF, Liang XY, Lin T, et al. Fatigue-resistant conducting polymer hydrogels as strain sensor for underwater robotics. Adv Funct Mater. 2023;33(42):2305705. doi:10.1002/adfm.202305705. [Google Scholar] [CrossRef]
23. Luo XY, Wan RT, Zhang ZX, Song MT, Yan LX, Xu JK, et al. 3D-printed hydrogel-based flexible electrochromic device for wearable displays. Adv Sci. 2024;11:2404679. doi:10.1002/advs.202404679. [Google Scholar] [PubMed] [CrossRef]
24. Ke XL, Mu XJ, Chen SY, Zhang ZX, Zhou JH, Chen YL, et al. Reduced graphene oxide reinforced PDA-Gly-PVA composite hydrogel as strain sensors for monitoring human motion. Soft Sci. 2023;3:21. doi:10.20517/ss.2023.14. [Google Scholar] [CrossRef]
25. Li JH, Cao J, Lu BY, Gu GY. 3D-printed PEDOT: pSS for soft robotics. Nat Rev Mater. 2023;8(9):604–22. doi:10.1038/s41578-023-00587-5. [Google Scholar] [CrossRef]
26. Zhao Q, Wen HK, Wu JY, Wen XL, Xu ZY, Duan JF. Galactomannan/graphene oxide/Fe3O4 hydrogel evaporator for solar water evaporation for synergistic photothermal power generation. Desalination. 2024;570:117064. doi:10.1016/j.desal.2023.117064. [Google Scholar] [CrossRef]
27. Zheng DQ, Shi L, Zhang M, Huang WX, Li ZH, Long SF, et al. A self-floating and windproof Janus biomass composite hydrogel with magnetic controllability and salt rejection for polluted seawater desalination. Chem Eng J. 2024;497:154943. doi:10.1016/j.cej.2024.154943. [Google Scholar] [CrossRef]
28. Lyu T, Wang ZY, Liu RN, Chen K, Liu H, Tian Y. Macroporous hydrogel for high-performance atmospheric water harvesting. ACS Appl Mater. 2022;14(28):32433–43. doi:10.1021/acsami.2c04228. [Google Scholar] [PubMed] [CrossRef]
29. Li W, Liao GC, Duan W, Gao FF, Wang YS, Cui RX, et al. Synergistically electronic interacted PVDF/CdS/TiO2 organic-inorganic photocatalytic membrane for multi-field driven panel wastewater purification. Appl Catal B-Environ. 2024;354:124108. doi:10.1016/j.apcatb.2024.124108. [Google Scholar] [CrossRef]
30. Ghosh R, Baut A, Belleri G, Kappl M, Butt HJ, Schutzius TM. Photocatalytically reactive surfaces for simultaneous water harvesting and treatment. Nat Sustain. 2023;6(12):1663–72. doi:10.1038/s41893-023-01159-9. [Google Scholar] [CrossRef]
31. Lou XX, Gao X, Liu Y, Chu MY, Zhang CY, Qiu YH, et al. Highly efficient photothermal catalytic upcycling of polyethylene terephthalate via boosted localized heating. Chin J Catal. 2023;49:113–22. doi:10.1016/S1872-2067(23)64435-3. [Google Scholar] [CrossRef]
32. Ma JH, Xu L, Yin ZY, Li ZF, Dong XY, Song ZG, et al. One stone four birds design atom co-sharing BiOBr/Bi2S3 S-scheme heterojunction photothermal synergistic enhanced full-spectrum photocatalytic activity. Appl Catal B-Environ. 2024;344:123601. doi:10.1016/j.apcatb.2023.123601. [Google Scholar] [CrossRef]
33. Zhang LJ, Jin ZL, Tsubaki N. Activating and optimizing the MoS2@MoO3 S-scheme heterojunction catalyst through interface engineering to form a sulfur-rich surface for photocatalyst hydrogen evolution. Chem Eng J. 2022;438:135238. doi:10.1016/j.cej.2022.135238. [Google Scholar] [CrossRef]
34. Altan O, Altintas E, Alemdar S, Metin Ö. The rational design of a graphitic carbon nitride-based dual S-scheme heterojunction with energy storage ability as a day/night photocatalyst for formic acid dehydrogenation. Chem Eng J. 2022;441:136047. doi:10.1016/j.cej.2022.136047. [Google Scholar] [CrossRef]
35. Xiong RZ, Ke XX, Jia WF, Xiao YH, Cheng BC, Lei SJ. Photothermal-coupled solar photocatalytic CO2 reduction with high efficiency and selectivity on a MoO3−x@ZnIn2S4core-shell S-scheme heterojunction. J Mater Chem A. 2023;11(5):2178–90. doi:10.1039/D2TA09255G. [Google Scholar] [CrossRef]
36. Yu F, Luo CJ, Niu XH, Chao M, Zhang PF, Yan LK. An advanced 2D/3D g-C3N4/TiO2@MnO2 multifunctional membrane for sunlight-driven sustainable water purification. Nano Res. 2023;17(4):2368–80. doi:10.1007/s12274-023-6071-6. [Google Scholar] [CrossRef]
37. Yang CC, Zhang X, Zhou Y, Hao SX. Well-designed MOF-derived hollow octahedral structure TiO2 coupled with ultra-thin porous g-C3N4 to enhance the degradation of real liquor brewing wastewater. Appl Surf Sci. 2023;616:156471. doi:10.1016/j.apsusc.2023.156471. [Google Scholar] [CrossRef]
38. He PP, Lan HY, Bai HY, Zhu YY, Fan ZF, Liu J, et al. Rational construction of all-in-one metal-organic framework for integrated solar steam generation and advanced oxidation process. Appl Catal B-Environ. 2023;337:123001. doi:10.1016/j.apcatb.2023.123001. [Google Scholar] [CrossRef]
39. Li PX, Yan XY, Gao SY, Cao R. Boosting photocatalytic hydrogen production coupled with benzyl alcohol oxidation over CdS/metal-organic framework composites. Chem Eng J. 2021;421:129870. doi:10.1016/j.cej.2021.129870. [Google Scholar] [CrossRef]
40. Zhang BP, Wong PW, An AK. Photothermally enabled MXene hydrogel membrane with integrated solar-driven evaporation and photodegradation for efficient water purification. Chem Eng J. 2022;430:133054. doi:10.1016/j.cej.2021.133054. [Google Scholar] [CrossRef]
41. Tao P, Ni G, Song CY, Shang W, Wu JB, Zhu J, et al. Solar-driven interfacial evaporation. Nat Energy. 2018;3(12):1031–41. doi:10.1016/j.nanoen.2022.108115. [Google Scholar] [CrossRef]
42. Li R, Li Y, Jia XH, Yang J, Miao X, Shao D, et al. 2D/2D ultrathin polypyrrole heterojunct aerogel with synergistic photocatalytic-photothermal evaporation performance for efficient water purification. Desalination. 2024;574:117295. doi:10.1016/j.desal.2024.117295. [Google Scholar] [CrossRef]
43. Jiang MM, Zheng RQ, Wang MJ, Li XQ. A MoS2-based evaporator with porous structure and interlayer channels for solar desalination and photocatalytic degradation. Sol Energy. 2024;279:112853. doi:10.1016/j.solener.2024.112853. [Google Scholar] [CrossRef]
44. Shu L, Zhang XF, Yang ST, Qiu JH, Yao JF. Integration of CuS@MIL-100 into cellulose hydrogel for synergistic seawater desalination and photocatalytic decontamination. Sep Purif Technol. 2024;334:125971. doi:10.1016/j.seppur.2023.125971. [Google Scholar] [CrossRef]
45. Yong ZJ, Yap LW, Fu RF, Shi QQ, Guo ZR, Cheng WL. Seagrass-inspired design of soft photocatalytic sheets based on hydrogel-integrated free-standing 2D nanoassemblies of multifunctional nanohexagons. Mater Horiz. 2021;8(9):2533–40. doi:10.1039/D1MH00753J. [Google Scholar] [PubMed] [CrossRef]
46. Zhao YW, Zhang YJ, Liu AR, Wei ZZ, Liu SQ. Construction of three-dimensional hemin-functionalized graphene hydrogel with high mechanical stability and adsorption capacity for enhancing photodegradation of methylene blue. ACS Appl Mater. 2017;9(4):4006–14. doi:10.1021/acsami.6b10959. [Google Scholar] [PubMed] [CrossRef]
47. Zhang W, Zhang YM, Liu Y. Cyclodextrin-cross-linked hydrogels for adsorption and photodegradation of cationic dyes in aqueous solution. Chem Asian J. 2021;16(16):2321–17. doi:10.1002/asia.202100535. [Google Scholar] [PubMed] [CrossRef]
48. Huang DL, Li ZH, Zeng GM, Zhou CY, Xue WJ, Gong XM, et al. Megamerger in photocatalytic field: 2D g-C3N4 nanosheets serve as support of 0D nanomaterials for improving photocatalytic performance. Appl Catal B-Environ. 2019;240:153–73. doi:10.1016/j.apcatb.2018.08.071. [Google Scholar] [CrossRef]
49. Wang Q, Wang T, Laila N, Huang K, Wang XW, Lei RB, et al. Carbon dots/TiO2 enhanced visible light-assisted photocatalytic of leachate: simultaneous effects and Mechanism insights. Water Res. 2023;245:120659. doi:10.1016/j.watres.2023.120659. [Google Scholar] [PubMed] [CrossRef]
50. Zhang LN, Liu TL, Liu TF, Hussain S, Li QY, Yang JJ. Improving photocatalytic performance of defective titania for carbon dioxide photoreduction by Cu cocatalyst with SCN-ion modification. Chem Eng J. 2023;463:142358. doi:10.1016/j.cej.2023.142358. [Google Scholar] [CrossRef]
Cite This Article
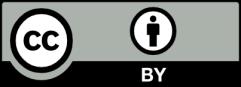
This work is licensed under a Creative Commons Attribution 4.0 International License , which permits unrestricted use, distribution, and reproduction in any medium, provided the original work is properly cited.