Open Access
REVIEW
Implication of Water-Rock Interaction for Enhancing Shale Gas Production
1 Shale Gas Research Institute, PetroChina Southwest Oil and Gas Field Company, Chengdu, 610056, China
2 Sichuan Province Key Laboratory of Shale Gas Evaluation and Exploitation, PetroChina Southwest Oil and Gas Field Company, Chengdu, 610056, China
3 State Key Laboratory of Oil and Gas Reservoir Geology and Exploitation, Southwest Petroleum University, Chengdu, 610500, China
* Corresponding Author: Lijun You. Email:
(This article belongs to the Special Issue: Fluid and Thermal Dynamics in the Development of Unconventional Resources II)
Fluid Dynamics & Materials Processing 2024, 20(7), 1441-1462. https://doi.org/10.32604/fdmp.2024.051200
Received 29 February 2024; Accepted 07 June 2024; Issue published 23 July 2024
Abstract
Horizontal well drilling and multi-stage hydraulic fracturing technologies are at the root of commercial shale gas development and exploitation. During these processes, typically, a large amount of working fluid enters the formation, resulting in widespread water-rock interaction. Deeply understanding such effects is required to optimize the production system. In this study, the mechanisms of water-rock interaction and the associated responses of shale fabric are systematically reviewed for working fluids such as neutral fluids, acid fluids, alkali fluids and oxidative fluids. It is shown that shale is generally rich in water-sensitive components such as clay minerals, acid-sensitive components (like carbonate minerals), alkali-sensitive components (like quartz), oxidative-sensitive components (like organic matter and pyrite), which easily lead to change of rock fabric and mechanical properties owing to water-rock interaction. According to the results, oxidizing acid fluids and oxidizing fracturing fluids should be used to enhance shale gas recovery. This study also indicates that an aspect playing an important role in increasing cumulative gas production is the optimization of the maximum shut-in time based on the change point of the wellhead pressure drop rate. Another important influential factor to be considered is the control of the wellhead pressure considering the stress sensitivity and creep characteristics of the fracture network.Keywords
In organic-rich shale reservoirs with abundant natural gas resources, methane gas resides in both a free state and an adsorbed state in organic matter hosted pores and intercrystalline or intergranular pores among clay minerals [1]. Shale gas output generally goes through a multi-scale transmission including desorption, diffusion, and flow, and production efficiency is very low owing to the slow desorption and diffusion of adsorbed gas and large diffusional and filtrational resistance against free gas in nano-pores [2]. Therefore, commercial shale gas production is achieved through horizontal well drilling and multi-stage hydraulic fracturing of sweet-spot sections to create a flow field with high permeability [3,4].
Physical and chemical reactions usually occur between shale rock and working fluids, such as water-based drilling fluids, alkaline oil-based drilling fluids, slick water, acid fluids, and oxidative gel breakers, in the whole process of economic shale gas production [5]. Water-rock interaction in shale reservoirs may affect borehole stability during drilling, fracturing stimulation performance, shut-in performance and flowback operations. This raises a challenge for well drilling, well hydraulic fracturing, and well management in the context of geology-engineering integration for shale gas development.
Comprehensively understanding the mechanism and characteristics of water-rock interaction in shale gas reservoirs is important to the optimization of working fluid formulas and production systems. Hence, in this study, the interaction mechanisms between shale rock and working fluids commonly used in shale gas wells, as well as the responses of shale fabric to water-rock interaction, are reviewed, and the implications of water-rock interaction for enhancing gas production are discussed.
2 Mechanisms of Water-Rock Interaction in Shale Gas Wells
According to the principles of mineral classification, shale components are classified into three categories: clay minerals, carbonate minerals (including calcite and dolomite), and others (including quartz, pyrite, feldspar, and phosphate) [6]. Clay minerals exposed to water are liable to swell carbonate minerals exposed to acid fluids are liable to dissolve quartzes and clay minerals exposed to alkaline fluids are prone to erode, and organic matter and pyrite tend to settle in a reducing environment, and are apt to be dissolved in an oxygen-rich environment.
As shown in Table 1, the efficiency of shale components’ dissolution by neutral fluids is characterized using a hydrolysis constant, where a small hydrolysis constant indicates a large difficulty of mineral hydrolysis [7]. Ali et al.’s experiments showed indistinct mineral dissolution in shale debris samples (with a particle diameter of ~1 μm) soaked in distilled water for 21 d at 65°C (with a solid-to-liquid ratio of 1 g:5 ml), and these samples exhibited extremely low hydrolysis rates for each mineral component (Table 2) [8].
Shale is rich in clay minerals that comprise illites, illite/smectite mix-layers, chlorites, and kaolinites, and the crystal structures and physical-chemical properties of which determine the strong hydratability of shale rock. Water molecules and hydrated cations are adsorbed and accumulated on the surface of the clay mineral or crystal layers to form an electric double layer, which increases the interlayer repulsion force and interlayer spacing interval and leads to the hydration swelling of clay minerals [9]. Clay hydration is controlled by three main mechanisms: surface hydration, ionic hydration, and osmotic hydration. Surface hydration is initiated by water molecule absorption by clay minerals due to surface hydration energy, ionic hydration refers to the hydration shell forming around compensatory cations on silicate crystals in clay minerals, and osmotic hydration launched after the first two processes is jointly actuated by the repulsion force of the diffuse electric double layer and osmotic pressure [10]. The distance needed to generate surface hydration, which occurs for all clay minerals, is about 1 nm. The distance needed to generate osmotic hydration is larger than 10 nm, and it takes a long time to reach equilibrium due to large volumetric expansion and small swelling pressure [11].
Compared to water-based drilling fluids, oil-based drilling fluids with strong anti-pollution capacity and inhibitory capacities can maintain borehole stability and have been widely applied to shale gas wells. To preserve the rheological properties of oil-based drilling fluids and the activities of various treating agents, sodium hydroxide (NaOH) or lime is generally used to adjust the pH value of drilling fluids. The pH value of oil-based drilling fluids is usually higher than 9 [12,13].
Due to high clay content and small clastic mineral particles, shale samples exposed to alkaline fluids are liable to be eroded [12,13]. The corrosion rates of quartz, feldspar, and clay minerals increase with an increasing pH value in an alkaline environment [14]. The corrosion rate of quartz remains unchanged at a pH value of 2.0–8.5 and then sharply increases with the pH value of alkaline fluids; a rise in temperature will also elevate the corrosion rate [15]. Feldspar may react with NaOH solutions at certain concentrations. Alkaline fluids could erode clay minerals, such as kaolinite, smectite, and illite. After erosion, kaolinites will generate amorphous silicon, gibbsite, albites, and analcimes [12]. Table 3 shows complicated and even multi-step associated reaction equations between shale components and alkaline fluids. The intensity of shale mineral erosion by alkaline fluids is ranked as smectite > kaolinite > illite > plagioclase > quartz > orthoclase [16]. The reaction between shale and alkaline fluids mainly generates silicate with some carbonate compounds and hydrated oxides [17]. According to the experiments by Kang et al., the reaction between Longmaxi shale samples from the Sichuan Basin and alkaline fluids is very slow; additionally, they found a positive power correlation between alkaline fluid concentration and reaction rate and a positive exponential correlation between temperature and reaction rate [18].
As a type of working fluid frequently used in reservoir stimulation, acid fluids may erode the microstructure of shale rock directly and initiate various complicated reactions (Table 4). Carbonate minerals exposed to acid fluids show high activity; hydrochloric acid (HCl) reacts quickly with calcites and slowly with dolomites. The reaction experiments at 60°C for individual minerals and acid fluids (pH = 4) showed a calcite reaction rate of 10−5–10−3 mol/m2 s and a dolomite reaction rate of 10−7–10−5 mol/m2s [19]. When HCl erodes chlorite crystal layers, the leaching out of Al3+, Mg2+, and Fe2+ ions causes excessive dissolution of chlorites [20], and H+ will cause surface dissolution [21]. Surface crystal lattices of kaolinites with almost no isomorphous replacement exhibit extremely high stability in acid fluids and chemically react only with high-concentration (about 21–26%) HCl at high temperatures [22]. The overall structure of illites is destroyed when reacting with HCl, and then, the corroded illites completely peel off from the surface of the shale samples [23]. The products of illite acidification reactions are the primary source of fine migration and could result in pore throat blinding and reservoir permeability decline [24]. In addition, a high-temperature environment facilitates the acid corrosion of illites, chlorites, and albites. Experiments by Black et al. [25] showed an albite reaction rate of ~10−10 mol/m2s and a clay mineral reaction rate of 10−14–10−10 mol/m2s in acid fluids of pH = 4 at 60°C. The reaction rate of kaolinite or illite is higher than that of chlorite and smectite. Moreover, pyrite is among the common minerals in the sulfide family and could be dissolved effectively in HCl.
When black shale formations are tectonically uplifted to crop out, the chemical equilibrium of organic matter and pyrite occurrence is upset due to rising oxidation-reduction potential; hence, oxygenolysis reactions can easily occur in oxygen-rich surface water [26]. Acid water usually generated in the oxidative dissolution of organic matter or pyrite, may corrode carbonate minerals and cause metallic cations to leach out of feldspar, illites, and chlorites and could cause hydrolysis of silicate minerals. It is hard to decompose quartzes in acid and oxidative environments [27]. As shown in Table 5, which compares black shale component stabilities in the land surface environment, organic matter and pyrite are the major objects of weathering and oxidation.
Oxidizing agents such as hydrogen peroxide (H2O2), ammonium persulfate (Na2S2O8), and chlorine dioxide (ClO2) have been widely used in well drilling, well completion, stimulation, and enhanced recovery [28–30]. Experiments by Mikutta et al. [31] revealed the dissolution of organic matter in the soil by H2O2, Na2S2O8 and sodium hypochlorite (NaClO) solutions. Oxidative degradation of organic matter in coals accompanies decarboxylation and then the generation of olefin. As the degree of oxidation increases, decarboxylation and ring-opening reactions arise in turn [32]. Some experiments have confirmed the dissolution of organic matter, epyrite, and carbonate minerals in shale by NaClO/H2O2 [33,34]. In the oxidation process of pyrite by H2O2 molecules, thiosulfates (e.g., S2O22− and S2O32) are produced first and are then oxidized to generate sulfates or are decomposed into elementary sulfurs and bisulfites [35]. There is a power correlation between the oxidation reaction rate of shale rock and H2O2 solution concentration and an exponential correlation between the reaction rate and temperature. For an H2O2 solution with a mass concentration ranging from 2–10%, the oxidation activation energy of Longmaxi shale samples ranges from 3.51–12.10 kJ/mol [36].
3 Responses of Shale Fabric to Water-Rock Interaction
According to the chemical properties of working fluids used in shale gas wells, water-rock interactions can be classified into two categories: non-chemical dissolution and chemical dissolution. The former mainly refers to the interaction between neutral fluids and shale rock. In spite of the extremely low degree of hydrolysis in shale, hydration swelling of clay minerals may give rise to a lot of cracks. The latter includes acid corrosion, alkaline erosion, and oxidative dissolution. Shale rock containing a complicated pore structure comprises organic matter-hosted pores, clay-mineral pores, and intercrystalline pores in brittle minerals. Besides, there are also abundant laminations and microfractures. The distribution of pores and microfractures is closely related to chemically unstable components, such as quartz, carbonate minerals, organic matter, and clay minerals [37].
The capillary effect in shale rocks and strong hydration swelling after water imbibed into pores and microfractures among mineral particles jointly lead to the generation, propagation, and connection of microfractures and final macroscopic rock failure [38]. According to fracture mechanics, material failure originates in the generation, propagation, and connection of internal microfractures. Tightly cemented shale is rich in microfractures, and swelling stress caused by clay hydration tends to induce stress concentration at the tip of the microfractures. As the swelling stress increases, continuous microfracture propagation l causes shale to burst and collapse once the swelling stress exceeds the critical stress at the fracture tip [39–41]. As shown in Fig. 1, non-clay mineral particles on the shale surface may fall off to form inorganic pores with a diameter from several microns to dozens of microns due to water-rock interaction. In addition, microfractures may occur between organic matter and inorganic minerals and inside inorganic minerals [38]. Liang et al. [42] established a shale crack propagation model involving hydration and wettability (capillary effect), and their results showed that hydration has a great impact on the increment of the stress intensity factor and tends to cause crack propagation. Yang established a model for calculating the release rate of mechanical energy at the tip of shale fractures under capillary force, and they quantitatively discussed the mechanical mechanism of subcritical crack growth under capillary force and explained the reason for shale bursts after water imbibition [43].
Figure 1: In situ field emission scanning electron microscopy (FESEM) observations of Longmaxi shale before and after soaking in distilled water
In situ conditions, inorganic pores that manifest as microfractures among clay mineral and non-clay mineral particles tend to cause hydration damage [44]. Hydration that mainly occurs along the lamination or pre-existing fractures goes through three stages: a stage with enlarged macropores and cracks, a stage with generated fine pores and enlarged macropores and cracks, and a stage with enlarged fine pores, connected macropores and induced cracks that cause hydration-induced fracturing [45]. Due to the combined effect of surface hydration of clay minerals, ionic hydration, and osmotic hydration, micro-scale hydration damage gradually evolves into macro-scale failure, which is regarded as successive loss of local rock mechanical strength. Hydration damage tends to occur in shale samples with high clay content, when shale fabric may be seriously destroyed over a short period of time [46]. Under high isotropic compressive stress, shale hydration is likely to close microfractures instead of producing new fractures; under low isotropic compressive stress, hydration induces fractures preferentially growing along lamination and reopening or extending pre-existing fractures [47,48]. Compared with isotropic compressive stress, anisotropic compressive stress is liable to produce hydration-induced fractures; the size of the induced fracture geometry and the number of fractures increase with increasing stress anisotropy [49–51].
Intense erosion of surface minerals on shale by alkaline fluids may generate many pores (Fig. 2), which increases the internal nano-pore volume and specific surface area, resulting in loosened rock fabric and significantly decreasing rock compression strength. This can consequently expand fractures, enlarge fracture width, and increase the stress sensitivity of shale fractures [18]. As indicated by Kang et al., hydration swelling and alkaline erosion induced by drilling fluid intrusion are the dominant types of shale chemical damage, burst and instability [52]. Since hydroxyl (OH−) concentration has a great positive effect on the hydration of non-swelling shale rock, alkaline erosion may also accelerate shale hydration swelling. Hydration swelling is intensified when NaOH or potassium hydroxide (KOH) solutions are nearly neutral, alleviated when the pH value ranges from 8 to 9, and enhanced once again when pH > 9 [53]. The hydratability of organic-rich shale increases with pH value; alkaline erosion and its effect on strengthening clay hydration play a role in promoting fracture propagation [54].
Figure 2: Scanning electron microscopy (SEM) observations of Longmaxi shale before and after soaking in alkaline fluids. Adapted with permission from reference [18], Copyright © 2016, Elsevier B. V
After treatment of acid fluids, the Bakken shale sample generated mass dissolved pores with the largest diameter of 120 μm, which remarkably improved pore connectivity [55]. Morsy et al. [56] used CT scanning to investigate the porosity change of shale samples acquired from different regions in North America after the acid treatment, in which carbonate-rich shale (Eagle Ford shale) showed a greatly improved porosity after acid corrosion, and clay-rich shale (Barnett shale) showed a negatively growing porosity (Table 6). Three types of microfractures may occur inside shale after acid treatment: stress-induced fractures, acid corrosion-induced fractures, and acidizing-induced contraction fractures. For clay-rich shale, acid corrosion may generate more stress-induced fractures at the boundaries of kaolinites and chlorites/biotites and more acidizing-induced contraction fractures in chlorite zones. For carbonate-rich shale, acid corrosion-induced fractures turn up in zones with anhydrites, calcites, illites, and organic matter [57].
Acid corrosion, which is affected by carbonate mineral distribution, may alter the microstructure of shale rock (Fig. 3), cementing strength of mineral particles, strength of rock mass, and rock deformation and failure [58]. According to the experiments of reactions between Eagle Ford shale samples and dilute hydrochloric acid (HCl) solutions reported by Morsy et al. at 93°C, the elastic moduli of rock samples were reduced by 25–82% after reactions [59]. Decreased shale hardness and microstructure alteration after acid damage are all related to the distribution of reaction minerals [55]. Acid corrosion deteriorates the mechanical strength and breakdown pressure of shale rock, which is favorable for hydraulic fracturing stimulation in shale reservoirs [60].
Figure 3: In situ FESEM observations of Longmaxi shale before and after soaking in acid fluids
Outcrop shale weathering mainly works on organic matter and pyrite, and acidulous water produced in the process corrodes carbonate minerals. Due to the erosion by acidulous water, mineral dissolution, generation of secondary minerals, and ion exchange adsorption lead to a change in mineral components and the ways of mineral particle connection, which induces a large number of micropores [61].
Kuila et al. [62] used low-pressure gas adsorption, X-ray diffraction, rock pyrolysis, and nitrogen adsorption to characterize the pore structures in shale samples from the Silurian System in Eastern Europe, Haynesville, the Paleozoic Erathem and Marcellus in North America, and the Baltic Sea Basin after the treatment with NaClO solutions. After the organic matter in shale rock with high thermal maturities was removed by oxidative dissolution, the volume of pores with diameters smaller than 5 nm was greatly reduced, whereas the volume of pores with diameters greater than 10 nm was greatly increased. Zhu et al. [63] adopted the same methodology to investigate the porosity, total organic carbon (TOC), and mineralogical features of shale samples from the Yanchang Formation in the Ordos Basin in China after treatment with H2O2 solutions, and the results showed a positive correlation between a decrease in TOC content and an increase in pore volume.
Chen et al. reported that the average diameter of nano-pores in Longmaxi outcrop shale increased from 4.9 to 24.5 nm after a sufficient reaction with H2O2 solutions, the nano-pore volume (with a pore diameter <193.5 nm) increased from 0.025 to 0.041 cm3/g, and the overall pore volume increased from 0.015 to 0.079 cm3/g [33]. Oxidative dissolution may accelerate subcritical crack growth, promote hydration-induced fracturing, and cause oxidative damage to shale rock to intensify macroscopic fracturing under axial stress [64–66]. Fig. 4 shows in situ dissolved pores and fractures generated by the oxidative dissolution of pyrite and organic matter [67].
Figure 4: In situ SEM observations of shale before and after oxidative dissolution. Adapted with permission from reference [67], Copyright © 2019, ACS Publishing
4 Implications for Enhancing Shale Gas Production
In view of many publications about the mechanisms as well as prevention and control of wellbore instability induced by water-rock interaction [17,52,54,68,69], this study mainly emphasized the improvement of fracturing stimulation and shut-in performance, particularly in terms of the optimization of working fluid formulas and the production system of shale gas wells, to discuss the potential significance of water-rock interaction in enhancing shale gas production.
4.1 Optimization of Working Fluid Formulas
With respect to acid fluids and slick water used in the fracturing stimulation of shale gas wells, this section deals with oxidizing acid fluids, which enhances the performance of acid fluids in reducing the breakdown pressure of shale rock, and with oxidizing fracturing fluids, which facilitates adsorbed gas desorption.
To strengthen hydraulic fracturing stimulation, a moderate amount of acid fluids is usually pumped into shale gas wells in advance to corrode carbonate minerals or other types of cement and thus reduce the breakdown pressure of rocks, which is mainly dependent on mineral composition, content, and distribution [64]. According to Eq. (1) (or Eq. (2)) for calculating the breakdown pressure of rocks with vertical (or horizontal) fractures after open hole completion, breakdown pressure is closely related to the porosity at the contact point, Poisson’s ratio, and unidirectional tensile strength in the reservoir [70]. Macroscopically, the chemical denudation of minerals impairs the cementing strength of rocks and consequently decreases the cohesive force and the angle of internal friction. Microscopically, microfractures originate in the propagation and connection of mass microfractures under stress.
Pbv=3σH−σh+σf−2ηPo1+ϕc−2η (1)
Pbh=Pob+σf−2ηPoϕc−2η (2)
η=ϕ(1−2ν)2(1−ν) (3)
Here σf —unidirectional tensile stress intensity of rocks (MPa);
σh and σH —horizontal minor principal stress and horizontal major principal stress (MPa);
Po —pore fluid pressure (MPa);
Pbv —vertical fracture actuating pressure (MPa);
Pbh —horizontal fracture actuating pressure (MPa);
Pob —burden pressure (MPa);
ϕc —porosity at the contact point (%);
ϕ —rock porosity (%);
ν —Poisson’s ratio.
For example, Table 7 shows the basic parameters of a shale gas well. Based on porosity change in the process of the oxidative dissolution shown in Table 8, Eq. (1) was used to calculate the breakdown pressure variation corresponding to the generation of a vertical fracture in the shale in the process of oxidative dissolution (Table 8). It indicates that oxidative dissolution plays an important role in the reduction of breakdown pressure for shale rock. Acid-oxidizing agents, such H2O2, could coexist with acid fluids, such as dilute HCl. Thus, oxidizing acid fluids (or mixtures of acid fluids and oxidative fluids) are superior to a single acid fluid in reducing the breakdown pressure of shale rock.
4.1.2 Oxidizing Fracturing Fluids
The statistical data of over 300 wells with pressure-relief production systems using chokes of 1013 mm in the Sichuan Basin showed a quick decline in wellhead pressure and daily gas production. Moreover, these wells did not show a period of stabilized production, and contained two-stage production with early quick decline and late slow decline, corresponding to casing-based production and tubing-based production, respectively. The stage of casing-based production witnessed an initial wellhead pressure of 27 MPa, an annual decline rate of 67%, and the estimated ultimate recovery (EUR) in this stage of 28%. The stage of tubing-based production featured an initial wellhead pressure of 7 MPa, an annual decline rate of 35%, and a EUR of 14%; the additional 58% of EUR can be recovered using other methods.
Regarding the production system, the constant-production reduced-pressure system was implemented at the initial stage of gas well production in this field (the first 2 years), when the production decline rate was high. The constant-pressure reduced-production system was adopted later, with a low production decline rate over a long period of time (Fig. 5). The stage of constant-pressure and reduced-production witnessed cumulative gas production of 6000 × 104 m3, accounting for 54% of EUR. It indicates that free gas in the fracture network was the major output with relatively high daily production at the initial stage. As the formation pressure dropped quickly, adsorbed gas was rapidly desorbed from the surface of organic matter and released through the fracture network, resulting in a descending decline rate and stable production over a long period at the late stage of production [72]. He et al. examined normal-pressure shale gas production in the Pengshui-Wulong area of Southeastern Chongqing, China, and demonstrated that adsorbed gas output as early as possible may slow down production decline to some extent [73].
Figure 5: Shale gas production performance from over 300 wells in a field in the Sichuan Basin. (a) Daily gas production and wellhead pressure variations with time; (b) Annual gas production, cumulative gas production, and production decline rate variations with time
Adsorbed gas, with its content accounting for 20%–85% of total gas content in shale, mainly occurs in nano-scale organic matter-hosted pores and inorganic pores with descending adsorptive capacities as organic matter-hosted pores > clay-mineral pores > quartz-hosted pores [74]. Shale samples with high TOC content show a large specific surface area and more sites for the adsorption of methane molecules, for which a linear positive correlation between methane adsorption quantity and TOC content can be found [75]. Adsorbed gas in shale mainly occurs in nano-pores with a diameter <5 nm [62], and organic matter provides more nano-pore volume for adsorbed gas. The structural damage of organic matter molecules and the structural improvement of micron-to nano-scale pores by oxidative dissolution may reduce the methane adsorption quantity in shale. Besides, water film on the pore surface could change the interaction properties of methane adsorption, and water molecules occupy the pore space due to the effect of capillary condensation and consequently decrease the effective specific surface area of methane adsorption [76,77]. That is why wet shale samples show a decreasing methane adsorption quantity [74]. Similar to adsorbed gas substitution by conventional fracturing fluids, oxidative dissolution of organic matter can generate more adsorbed water in shale [78]. Therefore, oxidizing fracturing fluids may promote adsorbed gas desorption and thus enhance shale gas recovery.
With respect to intra-fracture fluid pressure drop due to the filtration of fracturing fluids in shut-in, Yang concluded that water-rock interaction may always contribute to enhanced shut-in performance before intra-fracture fluid pressure falls to the minor principal stress according to simulations of microfracture propagation accelerated by water imbibition in shales [43]. Based on hydration-induced fracturing, oxidizing fracturing fluids will further promote rock fracturing through shut-in and will densify the fracture network.
4.2 Optimization of the Production System
A shale gas well usually goes through three stages after the fracturing operation: shut-in, flowback operations, and production testing. This section deals with the optimization of shut-in time in view of water-rock interaction and pressure-controlled production systems (PCPs).
At present, the shut-in time for a shale gas well is established mainly based on two points: proppant flowback control and sustained post-frac stimulation related to rock fracturing induced by water-rock interaction- and formation damage induced by the hydration [79]. In field applications, proppant flowback control usually conforms to the principle of intra-fracture fluid pressure falling below the fracture closing pressure after the termination of pumping; this is the shortest shut-in time. In lab experiments, the longest shut-in time is determined in accordance with the double effects of microscopic reservoir stimulation microfractures and formation damage induced by water-rock interaction.
In shut-in, fracturing fluids spread out along net-like hydraulic fractures, corresponding to intra-fracture fluid pressure attenuation due to fracturing fluid imbibition. The extension of early fracturing fluids from hydraulic fractures to secondary fractures is indicated by a large quick pressure drop, during which rock fracturing induced by water-rock interaction-may densify secondary fractures and increase the space for the water phase. As intra-fracture fluid pressure drops continuously, imbibition-induced fracturing is mitigated gradually. The fluids mainly invade along fracture planes into pores, leading to decreasing efficiency of fluid distribution, which is manifested as a small slow pressure drop. This means that a change point in the pressure drop occurs during the entire process; in other words, the efficiency of imbibition-induced fracturing arrives at a peak (Fig. 6).
Figure 6: The template to estimate the proper shut-in time of a shale gas well
To validate this viewpoint, wellhead pressure variation with shut-in time was investigated for nine shale gas wells in a field in the Sichuan Basin (Fig. 7a). A change point in the magnitude of the pressure drop can be found. Correspondingly, a change point in the rate of the pressure drop can be found at the same time point according to the derivative of logarithmic wellhead pressure with respect to logarithmic time (Fig. 7b). Shale fracturing induced by water-rock interaction dominates in the time interval before the change point, when a long shut-in time creates more good than harm for shut-in performance. Formation damage dominates in the time interval after the change point, when a longer shut-in time creates more harm than good. Hence, the change point is important for the optimization of the maximum shut-in time.
Figure 7: Relationships between wellhead pressure (a) and its derivative (b) and time for nine shale gas wells in the Sichuan Basin
For PCPs of a shale gas well, to slow down the decline of fracture conductivity, the production pressure difference usually is increased to the maximum gradually, accompanied by increasing in gas production to a peak and then decreasing gradually [80]. In spite of low daily gas production and cumulative gas production at the initial stage, there is a period of stabilized production with relatively high production to achieve a higher EUR (Fig. 8a) [81]. Similarly, shale gas reservoirs with high formation pressure in the Haynesville Basin in North America show strong stress sensitivity in the fracture network. Using PCPs, the average EUR of shale gas wells in Haynesville is generally elevated by 28% [82].
Figure 8: Comparisons between pressure-relief production and pressure-controlled production of shale gas wells in the Sichuan Basin: (a) Casing pressure and tubing pressure. (b) Daily gas production and cumulative gas production
A complicated hydraulic fracture network may be partially supported by the proppant (proppant-supported fractures or PF), and the others are self-supported with no proppant filled (self-supported fractures or SF). Compared with PF, SF with extremely strong stress sensitivity is easy to close [83]. During increased pressure-difference production with fast pressure relief from major fractures over a short period of time, the pressure sensitivity of fracture networks will result in a dramatic decrease in formation permeability due to the generation of formation damage areas close to the major fractures. It could prevent surrounding gas from moving into major fractures prematurely and thus remarkably decrease gas well production and cumulative production [84,85]. According to the study made by Zhu et al., daily gas production for a single shale well may decrease by 73.5% from reservoirs rich in microfractures and by 39.7% from reservoirs with underdeveloped microfractures owing to stress sensitivity [86]. Moreover, lubrication, hydration, and erosion triggered by the water-rock interaction may aggravate the stress sensitivity of fracture permeability [66,87–89]. Thus, the mechanical strength of shale rock is degraded [90–94], which could seriously harm the conductivity of artificial fractures with the help of stress loading [95–97].
Fig. 8b shows that the effective stress of shale gas reservoirs is relatively low at the early stage of gas well production. If the positive effect of increasing drawdown pressure on increasing production is poorer than the negative effect caused by the stress sensitivity of fracture networks, the drawdown pressure should be limited within a proper range to maintain hydraulic fracture conductivity. It can improve the utilization efficiency of the formation energy to produce gas, and can prolong the period of stabilized production in accordance with the stress sensitivity of fracture networks. At the late stage of gas well production, the effective stress of reservoirs is relatively high. If the effect of increasing drawdown pressure on increasing production is greater than the negative effect caused by the stress sensitivity of fracture networks, the drawdown pressure should be boosted in advance to fully release production, increase potential, and maximize the EUR, in view of the time effect of hydration-induced creep, which may promote fracture closure in shale.
In the context of hydraulic fracturing as a current irreplaceable technique and water-rock interaction which is unavoidable in subsurface conditions, understanding how to properly balance and control the double-sided effects of water-rock interaction on increasing production is important to shale gas production, as it both reduces costs and increases benefit. The main conclusions of this study are as follows:
(1) Shale is rich in water-sensitive clay minerals, acid-sensitive carbonate minerals, alkali-sensitive quartzes, and oxidative-sensitive organic matter and pyrite, and water-rock interaction is liable to change the rock fabric and rock mechanical properties.
(2) Promoting the application of oxidizing acid fluids and oxidizing fracturing fluids could be favorable for improving hydraulic fracturing and adsorbed gas output and consequently for enhancing shale gas recovery.
(3) The change points in the magnitude of the pressure drop and the peak time of water imbibition-induced fracturing could be determined through the statistical analysis of the measured shut-in pressure drop of shale gas wells to optimize the shut-in time.
(4) A PCPs with preserved fracture conductivity is significant to proper pressure control in the whole process of shale gas production, full utilization of producing energy, prolonged period of stabilized production, and EUR maximization.
However, the engineering adaptability of oxidizing acid fluids and oxidizing fracturing fluids needs further demonstration, and PCPs application needs to build a method to design the pressure drop path in production for target wells in the future.
Acknowledgement: This work is supported by the Innovative Research Project for Sichuan Youth Scientific and Technological Innovation and Postdoctoral Research Project of Petrochina Southwest Oil and Gas Field Company.
Funding Statement: Lijun, You, Innovative Research Project for Sichuan Youth Scientific and Technological Innovation (Grants No. 2016TD0016). Qiuyang Cheng, Postdoctoral Research Project of Petrochina Southwest Oil and Gas Field Company (Grants No. 20230304-13).
Author Contributions: The authors confirm contribution to the paper as follows: study conception and design: Qiuyang Cheng & Lijun You; data collection: Weiyang Xie & Haoran Hu; analysis and interpretation of results: Qiuyang Cheng & Cheng Chang; draft manuscript preparation: Qiuyang Cheng & Xingchen Wang. All authors reviewed the results and approved the final version of the manuscript.
Availability of Data and Materials: The data is available from the authors upon reasonable request.
Conflicts of Interest: The authors declare that they have no conflicts of interest to report regarding the present study.
References
1. Curtis JB. Fractured shale-gas systems. Am Assoc Pet Geol Bull. 2002;86(11):1921–38. [Google Scholar]
2. Zhang Y, Zhang B, Liu BH, Liu J, Wei QS, Zhang Q, et al. Status quo and development trends of research on shale gas adsorption and seepage in shale gas reservoirs. Oil & Gas Geol. 2024;45(1):256–80. [Google Scholar]
3. Nie HK, Dang W, Zhang K, Su HK, Ding JH, Li DH, et al. Two decades of shale gas research & development in China: review and prospects. Nat Gas Indus. 2024;44(3):20–52. [Google Scholar]
4. Ma XH, Zhang XW, Xiong W, Liu YY, Gao JL, Yu RZ, et al. Prospects and challenges of shale gas development in China. Petrol Sci Bul. 2023;8(04):491–501. [Google Scholar]
5. Pearce JK, Turner L, Pandey D. Experimental and predicted geochemical shale-water reactions: roseneath and Murteree shales of the Cooper Basin. Int J Coal Geol. 2018;187:30–44. doi:https://doi.org/10.1016/j.coal.2017.12.008. [Google Scholar] [CrossRef]
6. Jiang YQ, Dong DZ, Lin Q, Shen Y, Chan J, He F. Basic features and evaluation of shale gas reservoirs. Nat Gas Indu. 2010;30(10):7–12. [Google Scholar]
7. Krauskopf K, Bird D. Introduction to geochemistry. USA: McGraw-Hill; 1995. [Google Scholar]
8. Ali M, Hascakir B. Water/rock interaction for Eagle Ford, Marcellus, Green River, and Barnett shale samples and implications for hydraulic-fracturing-fluid engineering. SPE J. 2018;22(1):162–71. doi:https://doi.org/10.2118/177304-PA. [Google Scholar] [CrossRef]
9. Simpson JP, Dearing HL, Salisbury DP. Downhole simulation cell shows unexpected effects of shale hydration on borehole wall. SPE Dril Eng. 1989;4(1):24–30. doi:https://doi.org/10.2118/17202-PA. [Google Scholar] [CrossRef]
10. Williams PA, Anderson RL, Ratcliffe I, Greenwell HC, Cliffe S, Coveney PV. Clay swelling—a challenge in the oilfield. Earth-Sci Review. 2010;98(3):201–16. doi:https://doi.org/10.1016/j.earscirev.2009.11.003. [Google Scholar] [CrossRef]
11. Dachuan L. Review on the study of shale hydration mechanism. Dril Fluid Compl Fluid. 1997;14(16):31–3. [Google Scholar]
12. Mohnot SM, Bae JH, Foley WL. A study of mineral/alkali reactions. SPE Res Eng. 1987;2(04):653–63. doi:https://doi.org/10.2118/13032-PA. [Google Scholar] [CrossRef]
13. Kang YL, Wu ZJ, Wang JJ. Alkali sensitivity damage: a critical factor causing drastic decrease in oil production for Well Donghe 1 in Tarim Basin. J Southwest Petrol Uni. 1997;19(4):14–9. [Google Scholar]
14. Thomas M. The effect of supplementary cementing materials on alkali-silica reaction: a review. Cement Concrete Res. 2011;41(12):1224–31. doi:https://doi.org/10.1016/j.cemconres.2010.11.003. [Google Scholar] [CrossRef]
15. Yunfu Z, Wenjie X. Sedimentary petrology. Beijing, China: Geological Publishing House Press; 1986. p. 195–220 (In Chinese). [Google Scholar]
16. Xiong SC. Study on transport law of alkali solution in porous media (Ph.D. Thesis). School of Chinese Academy of Sciences: China; 2009. [Google Scholar]
17. She JP. Sudden instability mechanism of formation and sealing zone system around shale Wells (Ph.D. Thesis). Southwest Petroleum University: China; 2016. [Google Scholar]
18. Kang YL, She JP, Zhang H, You LJ, Yu YF, Song MG. Alkali erosion of shale by high-pH fluid: reaction kinetic behaviors and engineering responses. Nat Gas Sci Eng. 2016;29(2):201–10. doi:https://doi.org/10.1016/j.jngse.2016.01.013. [Google Scholar] [CrossRef]
19. Gonnenthal E, Spyoher N. Drift-Scale coupled processes (DST and THC seepage) Models. Las Vegas, NV, USA: Yucca Mountain Project; 2001. [Google Scholar]
20. Simon DE, Anderson MS. Stability of clay minerals in acid. In: SPE Formation Damage Control Symposium; 1990; Lafayette: Louisiana. SPE-19422-MS. [Google Scholar]
21. Hamer M, Graham RC, Amrhein C, Bozhilov KN. Dissolution of ripidolite (Mg, Fe-chlorite) in organic and inorganic acid solutions. Soil Sci Soci America. 2003;67(2):654–61. [Google Scholar]
22. Gajam SY. Some aspects of hydrochloric acid-leaching of kaolinite clay (Ph.D. Thesis). University of Arizona Campus Repository: USA, 1985. [Google Scholar]
23. Bibi I, Singh B, Silvester E. Dissolution of illite in saline-acidic solutions at 25°C. Geo Et Cosmo Acta. 2011;75(11):3237–49. doi:https://doi.org/10.1016/j.gca.2011.03.022. [Google Scholar] [CrossRef]
24. Kamal MS, Mahmoud M, Hanfi M. Clay minerals damage quantification in sandstone rocks using core flooding and NMR. Petrol Explo Prod Tech. 2019;9(1):593–603. doi:https://doi.org/10.1007/s13202-018-0507-7. [Google Scholar] [CrossRef]
25. Black JR, Carroll SA, Haese RR. Rates of mineral dissolution under CO2 storage conditions. Chem Geoy. 2015;399:134–44. doi:https://doi.org/10.1016/j.chemgeo.2014.09.020. [Google Scholar] [CrossRef]
26. Littke R, Klussmanu U, Krooss B, Leythaeuser D. Quantification of loss of calcite, pyrite, and organic matter due to weathering of toarcian black shale and effects on kerogen and bitumen characteristics. Geo Et Cosmo Acta. 1991;55(11):3369–78. doi:https://doi.org/10.1016/0016-7037(91)90494-P. [Google Scholar] [CrossRef]
27. Jew AD, Dustin MK, Harrison A, Joe-Wong CM, Thomas DL, Maher K, et al. Impact of organics and carbonates on the oxidation and precipitation of iron during hydraulic fracturing of shale. Energy Fuel. 2017;31(7):3643–51. [Google Scholar]
28. Sanders MP. The application of Oxidative Hydrothermal Dissolution (OHD) to organic-rich shales (Ph.D. Thesis). Southern Illinois University at Carbondale: USA; 2017. [Google Scholar]
29. Shaun D, Vikas A, Shikha S, Alexandra H. Effect of oxidative breakers on organic matter degradation, contaminant mobility and critical mineral release during shale-fracturing fluid interactions in the Marcellus Shale. Fuel. 2023;331(35):125678. doi:https://doi.org/10.1016/j.fuel.2022.125678. [Google Scholar] [CrossRef]
30. Zhu J, Zhou J, Yang Z, Xie X, Li X, Li P. Preparation and performance evaluation of microencapsulated acid used for gel breaking of fracturing fluid in low-temperature reservoirs. J Molec Liquid. 2024;403:124844–51. doi:https://doi.org/10.1016/j.molliq.2024.124844. [Google Scholar] [CrossRef]
31. Mikutta R, Kleber M, Kaiser K, Jahn R. Review: organic matter removal from soils using hydrogen peroxide, sodium hypochlorite, and disodium peroxodisulfate. Soil Sci Soci America. 2005;69(1):120–35. doi:https://doi.org/10.2136/sssaj2005.0120. [Google Scholar] [CrossRef]
32. Jing Z, Balucan RD, Underschultz JR, Steel KM. Oxidant stimulation for enhancing coal seam permeability: swelling and solubilisation behaviour of unconfined coal particles in oxidants. Fuel. 2018;221(8):320–8. doi:https://doi.org/10.1016/j.fuel.2018.02.071. [Google Scholar] [CrossRef]
33. Chen Q, Kang Y, You L, Yang P, Zhang X, Cheng Q. Change in composition and pore structure of Longmaxi black shale during oxidative dissolution. Int J Coal Geol. 2017;172(4):95–111. doi:https://doi.org/10.1016/j.coal.2017.01.011. [Google Scholar] [CrossRef]
34. Cheng QY, You LJ, Kang YL, Zhou Y, Zhang N. An experimental investigation into the oxidative dissolution of typical organic-rich shale from China. Mar & Petrol Geol. 2021;130:105117–27. doi:https://doi.org/10.1016/j.marpetgeo.2021.105117. [Google Scholar] [CrossRef]
35. Dimitrijevic M, Antonijevic MM, Jankovic Z. Kinetics of pyrite dissolution by hydrogen peroxide in perchloric acid. Hydrometallurgy. 1996;42(3):377–86. doi:https://doi.org/10.1016/0304-386X(95)00094-W. [Google Scholar] [CrossRef]
36. Cheng QY, You LJ, Kang YL, Zhou Y, Zhang N. Oxidative dissolution kinetics of organic-rich shale by hydrogen peroxide (H2O2) and its positive effects on improving fracture conductivity. Nat Gas Sci Eng. 2021;89:103875–88. doi:https://doi.org/10.1016/j.jngse.2021.103875. [Google Scholar] [CrossRef]
37. Kurotori T, Murugesu MP, Zahasky C, Vega B, Druhan JL, Benson SM. Mixed imbibition controls the advance of wetting fluid in multiscale geological media. Adv Water Resour. 2023;175:104429–41. doi:https://doi.org/10.1016/j.advwatres.2023.104429. [Google Scholar] [CrossRef]
38. Dou LD. Study on oxygen sensitivity and experimental evaluation method of organic-rich shale reservoirs (Master thesis). Southwest Petroleum University: China; 2019. [Google Scholar]
39. Wang Y, Liu X, Liang L, Liu XJ, Xiong J. Experimental study on the damage of organic-rich shale during water-shale interaction. Nat Gas Sci Eng. 2020;74:103103–17. doi:https://doi.org/10.1016/j.jngse.2019.103103. [Google Scholar] [CrossRef]
40. Liu B, Liang Y, Ito T. Numerical study on micro-cracks and permeability changes linked to clay swelling after fracturing in shale rock. Petrol Sci Eng. 2022;217:110847–61. doi:https://doi.org/10.1016/j.petrol.2022.110847. [Google Scholar] [CrossRef]
41. Li Z, Li G, Li H, Liu JY, Jiang ZJ, Zeng FH. Effects of shale swelling on shale mechanics during shale-liquid interaction. Energy. 2023;279:128098–114. doi:https://doi.org/10.1016/j.energy.2023.128098. [Google Scholar] [CrossRef]
42. Liang L, Xiong J, Liu X. Experimental study on crack propagation in shale formations considering hydration and wettability. Nat Gas Sci Eng. 2015;23:492–9. doi:https://doi.org/10.1016/j.jngse.2015.02.032. [Google Scholar] [CrossRef]
43. Bin Y. Study on fracture initiation and expansion behavior of shale induced by water phase imbibition. (Ph.D. Thesis). Southwest Petroleum University: China; 2018. [Google Scholar]
44. Chen X, Qu X, Xu S, Wang WM, Li S, He H, et al. Dissolution pores in shale and their influence on reservoir quality in Damintun Depression, Bohai Bay Basin, East China: insights from SEM images, N2 adsorption and fluid-rock interaction experiments. Mar Petrol Geol. 2020;117:104394–410. doi:https://doi.org/10.1016/j.marpetgeo.2020.104394. [Google Scholar] [CrossRef]
45. Abdullah D, Jagar AA, Mardin A. Exploring the role of hydrophobic nanofluids in reducing shale swelling during drilling: a step towards eco-friendly and sustainable practices. Coll Surf A: Physicochem Eng Aspects. 2024;694:134164–78. doi:https://doi.org/10.1016/j.colsurfa.2024.134164. [Google Scholar] [CrossRef]
46. Hafiz MA, Tanveer I, Mamdouh A, Muhammad SK. Synergistic effect of polymer and nanoparticles on shale hydration and swelling performance of drilling fluids. Petrol Sci Eng. 2021;205:108763–87. doi:https://doi.org/10.1016/j.petrol.2021.108763. [Google Scholar] [CrossRef]
47. Zhang S, Sheng JJ. Effect of water imbibition on hydration induced fracture and permeability of shale cores. Nat Gas Sci Eng. 2017;45(9):726–37. doi:https://doi.org/10.1016/j.jngse.2017.06.008. [Google Scholar] [CrossRef]
48. Zhang S, Sheng JJ. Effects of salinity and confining pressure on hydration-induced fracture propagation and permeability of Mancos shale. Rock Mech Rock Eng. 2017;50(11):2955–72. doi:https://doi.org/10.1007/s00603-017-1278-z. [Google Scholar] [CrossRef]
49. Zhang S, Sheng JJ. Effect of water imbibition on fracture generation in mancos shale under isotropic and anisotropic stress conditions.Geo-tech. Geo-Envir Eng. 2018;144(2):1–10. [Google Scholar]
50. Liu K, Sheng JJ. Experimental study of the effect of stress anisotropy on fracture propagation in Eagle Ford shale under water imbibition. Eng Geo. 2019;249:13–22. doi:https://doi.org/10.1016/j.enggeo.2018.12.023. [Google Scholar] [CrossRef]
51. Liu K, Sheng JJ, Zhang Z. A simulation study of the effect of clay swelling on fracture generation and porosity change in shale under stress anisotropy. Eng Geo. 2020;278(1–2):105829–45. doi:https://doi.org/10.1016/j.enggeo.2020.105829. [Google Scholar] [CrossRef]
52. Kang Y, Chen Q, You L, Lin C, Cheng Q. Laboratory studies of shale fracturing behaviors with rock-drilling fluid interactions. J China Uni Petrol. 2016;40(4):81–9 (In Chinese). [Google Scholar]
53. Wang X, Zhou S. Clay minerals and oil layer protection in sandstone reservoir. China: Geological Publishing House; 1992 (In Chinese). [Google Scholar]
54. Yu Y. Multi-scale structure description and instability mechanism of organic-rich shale (Ph. D Thesis). Southwest Petroleum University: China; 2013 (In Chinese). [Google Scholar]
55. Pearce JK, Blach T, Dawson G, Southam G, Paterson DJ, Golding SD. Cooper basin REM gas shales after CO2 storage or acid reactions: metal mobilisation and methane accessible pore changes. Int J Coal Geo. 2023;273(14):104271–90. doi:https://doi.org/10.1016/j.coal.2023.104271. [Google Scholar] [CrossRef]
56. Morsy S, Sheng JJ, Hetherington CJ, Soliman MY, Ezewu RO. Impact of matrix acidizing on shale formations. In: SPE Nigeria Annual International Conference and Exhibition; 2013; Lagos, Nigeria. SPE-167568-MS. [Google Scholar]
57. Ogochukwu O, Oladoyin K, Mohamed L, Tobi O, Thomas G, Hallie F, et al. Nano-to macro-scale structural, mineralogical, and mechanical alterations in a shale reservoir induced by exposure to supercritical CO2. Appl Energy. 2022;326:120051–64. doi:https://doi.org/10.1016/j.apenergy.2022.120051. [Google Scholar] [CrossRef]
58. Weldu TT, Park D, Jung H, Amini K, Abass H. Effect of dilute acid on hydraulic fracturing of carbonate-rich shale: experimental study. SPE Pro & Opera. 2019;34(1):170–84. [Google Scholar]
59. Morsy S, Hetherington CJ, Sheng JJ. Effect of low-concentration HCl on the mineralogy, physical and mechanical properties, and recovery factors of some shale. Unconv Oil Gas Res. 2015;9:94–102. [Google Scholar]
60. Zeng Y, Chen Z, Bian X. Breakthrough in staged fracturing technology for deep shale gas reservoirs in SE Sichuan Basin and its implications. Nat Gas Indus. 2016;36(1):61–7. [Google Scholar]
61. Bokanda E, Amaya A, Mary E, Florence N, Bisse S, Mokake F. Paleoclimate characteristics of source area weathering and metallogenic implication of cretaceous black shales in the Mamfe basin, (SW Cameroon). Evidence from Lithogeochem. 2023;9:17142–53. [Google Scholar]
62. Kuila U, McCarty D, Derkowski A, Fischer T, Topór T, Prasad M, et al. Nano-scale texture and porosity of organic matter and clay minerals in organic-rich mudrocks. Fuel. 2014;135:359–73. doi:https://doi.org/10.1016/j.fuel.2014.06.036. [Google Scholar] [CrossRef]
63. Zhu X, Cai J, Liu W, Lu X. Occurrence of stable and mobile organic matter in the clay−sized fraction of shale: significance for petroleum geology and carbon cycle. Int J Coal Geol. 2016;160:1–10. doi:https://doi.org/10.1016/j.coal.2016.03.011. [Google Scholar] [CrossRef]
64. Jew A, Druhan J, Ihme M, Kovscek A, Battiato I, Kaszuba J, et al. Chemical and reactive transport processes associated with hydraulic fracturing of unconventional oil/gas shales. Chem Rev. 2022;122(9):9198–63. doi:https://doi.org/10.1021/acs.chemrev.1c00504. [Google Scholar] [PubMed] [CrossRef]
65. Hull K, Singh S, Crane B, Saini R, AlRuwaili K, AlTammar M, et al. Oxidative hydraulic fracturing fluid to enhance production from source rock reservoirs. SPE J. 2024;11(3):1–10. doi:https://doi.org/10.2118/221449-PA. [Google Scholar] [CrossRef]
66. Li Y, Yang S, Liu D, Yang C, Yang Z, Li H, et al. Experimental study of shale-fluids interaction during oxidative dissolution with hydrogen peroxide, sodium hypochlorite and sodium persulfate. Appl Geochem. 2019;113:104503–12. doi:https://doi.org/10.1016/j.apgeochem.2019.104503. [Google Scholar] [CrossRef]
67. Hull KL, Jacobi D, Abousleiman Y. Oxidative kerogen degradation: a potentialapproach to hydraulic fracturing in unconventionals. Energy Fuel. 2019;33(6):4758–66. doi:https://doi.org/10.1021/acs.energyfuels.9b00104. [Google Scholar] [CrossRef]
68. Horsrud P, Holt RM, Sonstebo EF, Svano G, Bostrom B. Time dependent borehole stability: laboratory studies and numerical simulation of different mechanisms in shale. In: Rock Mechanics in Petroleum Engineering; 1994; Delft, Netherlands. SPE-28060-MS. [Google Scholar]
69. He W, Gomez S, Leonard R, Li D. Shale-fluid interactions and drilling fluid designs. In: International Petroleum Technology Conference; 2014; Doha, Qatar. IPTC-17235-MS. [Google Scholar]
70. Li C. Calculation formula of rock rupture pressure under perforation completion condition. Oil Dril Prod Tech. 2002;24(2):37–8. [Google Scholar]
71. Javed A, Eswaran P, Izhar U, Matthew A. Hydraulic fracturing with low and high viscous injection mediums to investigate net fracture pressure and fracture network in shale of different brittleness index. Geo Energy Environ. 2023;33:100416–27. [Google Scholar]
72. Hamzeh A, Chen N. Long-term production forecast in tight and shale reservoirs: adapting probability density functions for decline curve analysis. Gas Sci Eng. 2023;118:205113–24. doi:https://doi.org/10.1016/j.jgsce.2023.205113. [Google Scholar] [CrossRef]
73. He X, Zhang P, Fang D, Mei J, He G, Lu B. Production characteristics of normal pressure shale gas in Pengshui-Wulong area, southeast Chongqing. Petrol Geol Reco Effi. 2018;25(5):72–9. [Google Scholar]
74. Alharthy NS, Kobaisi AM, Kazemi H, Graves RM. Physics and modeling of gas flow in shale reservoirs. In: Abu Dhabi International Petroleum Conference and Exhibition; 2012; Abu Dhabi. UAESPE-161893-MS [Google Scholar]
75. Zhu X, Cai J, Wang X, Zhang J, Xu J. Effects of organic components on the relationships between specific surface areas and organic matter in mudrocks. Int J Coal Geol. 2014;133:24–34. doi:https://doi.org/10.1016/j.coal.2014.08.009. [Google Scholar] [CrossRef]
76. Wang L, Wang D, Cai C, Li C, Zhang N, Yang L, et al. Effect of water occupancy on the excess adsorption of methane in montmorillonites. Nat Gas Sci Eng. 2020;80:103393–400. doi:https://doi.org/10.1016/j.jngse.2020.103393. [Google Scholar] [CrossRef]
77. Babaei S, Ghasemzadeh H, Tesson S. Methane adsorption of nanocomposite shale in the presence of water: insights from molecular simulations. Chem Eng J. 2023;475:146196–11. doi:https://doi.org/10.1016/j.cej.2023.146196. [Google Scholar] [CrossRef]
78. Ross D, Bustin RM. Impact of mass balance calculations on adsorption capacities in microporous shale gas reservoirs. Fuel. 2007;86(17–18):2696–6. [Google Scholar]
79. Perera M. A review of underground hydrogen storage in depleted gas reservoirs: insights into various rock-fluid interaction mechanisms and their impact on the process integrity. Fuel. 2023;334:126677–89. doi:https://doi.org/10.1016/j.fuel.2022.126677. [Google Scholar] [CrossRef]
80. Wang G. Study on optimization method of pressure control production system in shale gas wells in South Sichuan. Modern Chem Res. 2022;18:174–6. [Google Scholar]
81. Jia A, Wei Y, Liu C, Wang J, Li B. A dynamic prediction model of pressure control production performance of shale gas fractured horizontal wells and its application. Nat Gas Indus. 2019;39(6):71–80. [Google Scholar]
82. Unomah GC, Ou L, Panfiloff A, Prasad M. Stress sensitivity of multiscale elastic properties under hydrostatic loading conditions of organic shales at different thermal maturity stages. Appl Geo. 2023;209:104906–17. [Google Scholar]
83. Zhu W, Ma D, Zhu H, An L, Li B. Stress sensitivity of shale gas reservoir and its influence on productivity. Nat Gas Geo. 2016;27(5):892–7. [Google Scholar]
84. Wei Y, Jia A, He D, Wang J, Han P, Jin Y. Comparative analysis of development characteristics and technologies between shale gas and tight gas in China. Nat Gas Indus. 2017;37(11):43–52. [Google Scholar]
85. Zhu W, Ma D. Effective stress characteristics in shale and its effect on pro-ductivity. Nat Gas Geo. 2018;29(6):845–52. [Google Scholar]
86. Jalal F, Amr I, Davood Z, Jalal D. Stress-dependent fluid dynamics of shale gas reservoirs: a pore network modeling approach. Nat Gas Sci Eng. 2021;95:104243–52. doi:https://doi.org/10.1016/j.jngse.2021.104243. [Google Scholar] [CrossRef]
87. Bandara KMAS, Ranjith PG, Haque A, Wanniarachchi WAM, Zheng W, Rathnaweera TD. An experimental investigation of the effect of long-term, time-dependent proppant embedment on fracture permeability and fracture aperture reduction. Int J Rock Mech Min Sci. 2023;161:105299–311. [Google Scholar]
88. Shoaib M, Runhua F, Muhammad A, Masood A, Ausama G, Alireza K, et al. Mohammad Sarmadivaleh aSupercritical CO2-Shale interaction induced natural fracture closure: implications for scCO2 hydraulic fracturing in shales. Fuel. 2022;313:122682–93. doi:https://doi.org/10.1016/j.fuel.2021.122682. [Google Scholar] [CrossRef]
89. Yang S, Hong W, Wang J, Ranjith P, Wang X. Experimental investigation on the influence of NaCl concentration on triaxial mechanical behaviors of a low-clay shale. Int J Rock Mech Min Sci. 2024;176:105716–24. doi:https://doi.org/10.1016/j.ijrmms.2024.105716. [Google Scholar] [CrossRef]
90. Huang H, Zhang W, Shi H, Ni J, Ding L, Yang B, et al. Experimental investigation of microscale mechanical alterations in shale induced by fracturing fluid contact. Gas Sci Eng, 2024;205264–74. [Google Scholar]
91. Tan J, Ding Y, Lyu Q, Hu C, Feng G, Yuan Q. Study on the constitutive model and brittleness variations of shale after imbibition in different fracturing fluids. Geo Energy Environ. 2023;34:100449–13. [Google Scholar]
92. Lane R, Aderibigbe A. Rock/fluid chemistry impacts on shale fracture behavior. society of petroleum engineers. In: SPE International Symposium on Oilfield Chemistry; 2013 Apr; Texas, USA: The Woodlands. SPE-164102-MS. [Google Scholar]
93. Antinao Fuentealba FJ, Blanco G, Bianchi LN, Otegui J, Bianchi G. Fracture toughness tests of shale outcrops. Geoenergy Sci Eng. 2024;232:212454–544. [Google Scholar]
94. Katende A, Rutqvist J, Benge M, Seyedolali A, Bunger A, Puckette J, et al. Convergence of micro-geochemistry and micro-geomechanics towards understanding proppant shale rock interaction: a Caney shale case study in southern Oklahoma, USA. J Nat Gas Sci Eng. 2021;96:104296–308. doi:https://doi.org/10.1016/j.jngse.2021.104296. [Google Scholar] [CrossRef]
95. Katende A, Awejori G, Bengs M, Nakagawa S, Wang Y, Xiong F, et al. Multidimensional, experimental and modeling evaluation of permeability evolution, the Caney Shale Field Lab, OK, USA. In: Unconventional Resources Technology Conference; 2023 Jun 13–15. p. 1505–26. [Google Scholar]
96. Katende A, Rutqvist J, Massion C, Radonjic M. Experimental flow-through a single fracture with monolayer proppant at reservoir conditions: a case study on Caney Shale, Southwest Oklahoma, USA. Energy. 2023;273:127181–92. doi:https://doi.org/10.1016/j.energy.2023.127181. [Google Scholar] [CrossRef]
97. Bandara K, Ranjith P, Rathnaweera T. Improved understanding of proppant embedment behavior under reservoir conditions: a review study. Powder Tech. 2019;352:170–92. doi:https://doi.org/10.1016/j.powtec.2019.04.033. [Google Scholar] [CrossRef]
Cite This Article
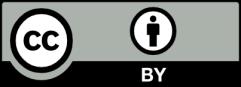
This work is licensed under a Creative Commons Attribution 4.0 International License , which permits unrestricted use, distribution, and reproduction in any medium, provided the original work is properly cited.