Open Access
ARTICLE
Energy Blockchain in Smart Communities: Towards Affordable Clean Energy Supply for the Built Environment
1 Electric Power Research Institute, State Grid Xinjiang Electric Power Co., Ltd., Urumqi, 830013, China
2 School of Energy and Power Engineering, Changsha University of Science & Technology, Changsha, 410114, China
3 Kumul Power Supply Company, State Grid Xinjiang Electric Power Co., Ltd., Urumqi, 830013, China
4 Xinjiang Key Laboratory of Extreme Environment Operation and Detection Technology of Power Transmission & Transformation Equipment, Urumqi, 830013, China
* Corresponding Author: Lida Liao. Email:
Energy Engineering 2024, 121(8), 2313-2330. https://doi.org/10.32604/ee.2024.048261
Received 02 December 2023; Accepted 08 April 2024; Issue published 19 July 2024
Abstract
The rapid growth of distributed renewable energy penetration is promoting the evolution of the energy system toward decentralization and decentralized and digitized smart grids. This study was based on energy blockchain, and developed a dual-biding mechanism based on the real-time energy surplus and demand in the local smart grid, which is expected to enable reliable, affordable, and clean energy supply in smart communities. In the proposed system, economic benefits could be achieved by replacing fossil-fuel-based electricity with the high penetration of affordable solar PV electricity. The reduction of energy surplus realized by distributed energy production and P2P energy trading, within the smart grid results in less transmission loss and lower requirements for costly upgrading of existing grids. By adopting energy blockchain and smart contract technologies, energy secure trading with a low risk of privacy leakage could be accommodated. The prototype is examined through a case study, and the feasibility and efficiency of the proposed mechanism are further validated by scenario analysis.Keywords
Given the prejudicial environmental effects of fossil fuel-based energy production, distributed renewable energy sources such as roof-top solar photovoltaics (PVs), small-scale wind turbines and photovoltaic thermal systems (PVTs) have drawn increasing attention in providing reliable affordable, and cleaner energy supplies. Statistics conducted by the IPCC revealed that renewable energy penetration has the potential to satisfy 70%–85% of the global energy demand, while in 2017, there was a global growth of 257 GW in renewable energy installation, outpacing the 70 GW increase in fossil fuels [1]. Total renewable energy generation in 2022 exceeded 8,500 TWH, an increase of more than 600 TWH from 2021. This growth was primarily driven by increases in wind and solar PV capacity, which both grew by nearly 270 TWh. Despite the constraints of climate conditions, renewable energy will account for nearly 30% of global electricity generation in 2022, an increase of 1.5 percentage points over 2021 [2]. The deployment of renewable energy has contributed significantly to global sustainability, and several nations have released ambitious carbon-neutral targets by 2050 [3,4]. In addition to environmental thinking, renewable energy harvesting alongside smart grids also explores the opportunity for cost-effective power production and distribution, which could be attributed to benefits including no supply limitations, better location matching of energy supply and demand, decreasing transmission and distribution losses, and reducing the requirement for cost-intensive grid upgrades [5]. Thus, transitioning to renewable energy and smart grids could be the trend of future energy system evolution.
Against the contribution of renewable energy and smart grids in economic low-carbon energy generation and distribution, it is important to verify their effect on energy policies and management. One of the major concerns is the challenge to existing centralized energy systems, which are unidirectional in nature [6]. However, the integration of distributed renewable energy production has been promoting a rapid transition of decentralization, decentralization, and digitization in energy supply and associated trading. In the context of bidirectional energy and information flows required in smart grids, the existing centralized energy grids soon reach their limits in facilitating the variable feed-in of renewable energy. These might result in power quality issues (e.g., voltage fluctuation, frequency jumps and drops, and reverse power flow), and empowering local P2P energy trading between prosumers and consumers to reduce the distribution of production surplus. Earlier investigations found that the output of a large PV plant could vary up to 30%–40% per minute due to uncontrollable local weather conditions, which might damage network equipment and electric appliances [7,8]. In addition, with the application of energy tariffs, the economic feasibility of renewable energy deployment might be challenged by the significant gap between feed-in and retail rates. P2P energy trading could maximize the benefits by reducing the production surplus on the condition that bidirectional physical and financial flows are enabled to satisfy the functional requirements of a smart grid [9]. In terms of secure energy trading, the existing centralized energy grid also suffers from cybersecurity concerns, as private information and sensitive energy-consuming data are exposed and shared among engaged intermediaries during transactions, which might result in a higher risk of data tampering and privacy leakage [10]. Moreover, the growth of data exchange in transactions would become the bottleneck of servers and make centralized grids more vulnerable to attack [11]. In light of the above discussion, it could be concluded that the evolution of energy systems requires novel network architectures and related technologies to empower flexible and sustainable distribution and trading.
The emergence of blockchain and smart contracts provides a solution to overcome the barriers of smart grid operations within smart communities. Although these new technologies are still in their infancy, they have shown obvious technical advances and great potential in addressing the concerns arising from the developments of the energy internet and sustainable P2P energy trading underpinned by smart contracts. As an immutable distributed ledger, blockchain possesses a unique set of inherent features that adds value to energy system operations, markets, prosumers, and consumers. On the one hand, blockchain might facilitate transparent, disintermediated, and distributed platforms for the energy internet. Previous studies indicate that smart grids and microgrids have been progressively promoting the transition of energy system decentralization, as the increasing size and complexity of data associated with the growth of residential-scale and intermittency renewable energy generation render inefficient centralized grids [12–14]. The adoption of a distributed blockchain platform provides prosumers with easier access to the energy market, which is consistent with the emerging needs in energy management and P2P trading. On the other hand, blockchain promises tamper-proof and secure data exchange for P2P energy trading in smart grids. Attributed to the decentralized consensus mechanism, instantaneous recording, and cryptographic security measurement, the energy blockchain is more resilient and reliable than existing centralized systems. Despite the absence of intermediaries, the security, resilience, efficiency, and accuracy of remaining energy blockchain systems can be empowered by predefined rules with high-level fault tolerance and resistance in data tampering, which has been examined and validated by considerable recent prototype studies and trial practices [15]. In addition, blockchain also has the potential to facilitate distributed, P2P trading with reduced transaction costs and increased prosumer choice. Recent studies have highlighted that energy blockchain might lead to 20%–30% cost savings in the absence of intermediaries, and a cost reduction of up to 71% can be achieved in specific periods and areas [16–18]. Moreover, the matching of consumption and generation in smart communities would further benefit the grid by reducing transmission losses and deferring expensive distribution network upgrades [19].
Energy blockchain has started to prove its potential in facilitating decentralized smart grids; however, a variety of challenges still need to be mitigated for future adoption and expansion. For example, scalability and social inclusion are two key challenges in energy blockchain adoption [13,20]. With the expansion of participant (both producers and consumers) groups, the operational complexity in the transfer of value and information, as well as an effective interface with the physical infrastructure, would increase significantly; therefore, maintaining real-time trading and network resilience becomes a major challenge. Meanwhile, promoting social inclusion is also of essential importance, as the participants must be encouraged to use this modern technology. However, a lack of technical knowledge and supportive legal frameworks might be barriers in participant institutions [21]. Fortunately, recent progress in consensus algorithms and smart contract development has found a pathway to overcome these barriers. Blockchain 2.0 enables smart contracts, which are digital protocols acting as a functional extension of blockchain to execute decentralized transactions in an automatic and secure manner with predefined laws. The next generation of Blockchain 3.0 is further expected to enable higher-level self-organized smart contracts with transaction records and program rules to be maintained within the chain [22]. Thus, in the context of blockchain and P2P-underpinned smart grids, smart contracts could be the cornerstone to encourage social inclusion by releasing the threshold of expert knowledge and enabling fully decentralized market platforms. Over the years, a considerable number of smart contract schemes have been developed to support the energy blockchain. Miglani et al. demonstrated a smart contract system developed to store trading information and empower automatic fund transfer in an energy blockchain [23]. Mihaylov et al. [24] highlighted the bidding mechanism adopted for renewable energy trading in smart grids. Reijnders et al. [25] introduced a combinatorial double auction mechanism for energy distribution in microgrid grids. Lu et al. [26] proposed smart dynamic tariffs for energy trading in a hierarchical electricity market by balancing both retailers’ and consumers’ benefits, where reinforcement learning is employed to illustrate the hierarchical decision-making framework. Therefore, there is wide-ranging potential for researchers to specialize in this field and add value to the concept and foundational work.
To investigate the blockchain-promoted P2P energy trading pillar of the smart grid evolutionary process, this paper proposes the development of a dual-biding-based smart contract system to improve the energy distribution capability and maximize the benefits of participants in a smart community context. The remainder of this article is structured to first include an introduction to system attributes and framework development (Section 2). This is followed by the formulation of mathematical models in Section 3, and Section 4 presents a case study with scenario analysis. Finally, Section 5 draws concluding remarks and suggestions for future work.
2 System Attribute Framework for Modeling
2.1 Energy Blockchain in Smart Communities
The concept of smart cities can be traced back to the 1960s, which was initially proposed to collect data for the US Community Analysis Bureau. However, after decades of development, there is still no global definition of the term [27]. A definition from IBM describes the concept as “a city that makes optimal use of all the interconnected information available today to better understand and control its operations and optimize the use of limited resources” [28]. The sustainability and resilience nature, as well as the functional requirement of providing a high-quality life for smart cities, have also been highlighted in some existing studies [11]. Sustainable energy supply with renewable energy penetration in smart communities has been promoting the development of distributed smart grids. However, unlike the “broadcasting” manner for energy distribution used in conventional grids, smart grids require bidirectional flows of physical energy, as well as trading and financial data. The emergence of energy blockchain provides the potential to overcome the application barriers. The conceptual framework of an energy blockchain is presented in Fig. 1.
Figure 1: The energy blockchain model in a smart community
As illustrated in Fig. 1, there are two types of participants in the smart community: Residential PV prosumers (both producers and consumers) and consumers. A smart grid composed of PV systems, smart meters, and community-level energy storage is developed to support producers with local energy trading, offer real (near) real-time pricing, and facilitate a local balance of supply and demand. To improve the resilience of a local energy system, the smart grid remains connected to the national grid. The energy blockchain is integrated with the smart grid to empower trading and transactions. Due to its distributed nature, instantaneous recording, and cryptographic signatures, the energy blockchain could be more tampering proof. Smart contracts can facilitate P2P energy trading, balance local energy production and demand, enable dynamic energy tariffs, and process transactions in a real-time, smart, and autonomous manner. The operation of this energy blockchain can be described as follows:
1) Energy surplus from the prosumers is transferred to the community energy bank for storage. Simultaneously, a transaction request would be sent by proposing a smart contract including bidding information such as the rate and volume of energy available for trading, address of the supplier (prosumer), time of the auction, and timestamp in the community.
2) Before broadcasting the smart contract on the energy internet, the state machine checks whether the transaction can fulfill the requirements for trading and confirms that the contract is legal and effective. As yet, the smart contract has been approved and is ready for bidding, with the feed-in energy from suppliers and relevant information recorded in the blockchain. Simultaneously, bidders would post their energy demand and quoted rates. The rates can be proposed by bidders or suggested by the energy internet based on local energy market profiles.
3) According to the predefined dual-bidding mechanism, the suppliers’ offers and bidders’ offers are sorted with the market clearing price (MCP) and then determined. MCP, also known as the equilibrium price, exists when an energy market is clear of shortage and surplus. The engagement of automatically generated MCP in energy blockchain trading can balance the local energy market, namely, ensuring that no energy surplus waiting to be sold and no bidders lose their opportunities to purchase. After that, the smart contract will execute the transaction after bidding according to the transaction agreement, and only the bidders’ offers matching the clearing price will be processed for the transaction. Here, confirmation is required to activate the contract once the purchase is finalized.
4) A full transaction description for bidding requires the balance of the producer’s account, the real-time electricity surplus for trading, signatures, and public keys recorded to ensure security and successful transactions. The details of the consumer’s current account are also made public to ensure that there is sufficient capital for bidding and purchasing.
5) After both parties confirmed the transaction, they would sign the smart contract separately. In the energy blockchain of the whole smart community, the smart contract will be executed strictly according to the established rules; therefore, there is no need to conduct electricity transactions through a third party, which means that the transaction efficiency can be greatly improved. When the transaction is completed, it is confirmed by the consensus mechanism and recorded in the block, which cannot be changed after the block is entered into the chain, and the transaction is finally completed.
2.2 Dual-Biding Mechanism Underpinned Smart Contracts
Widespread renewable energy harvesting with small-scale roof-top equipment such as solar PVs and heating units has been boosting the decarbonization of cities at the cost of making energy storage, distribution, and tariffs increasingly more complex. The decentralized nature of the energy blockchain enabled flexible P2P trading in the local smart grid; however, the related energy tariffs were significantly challenged. Most existing studies suggest a bidding mechanism based on local energy demand, which could maximize the benefit of prosumers and/or energy retailers; however, it might directly ‘encourage’ cut-throat competition and hurt the local energy market. A dual-biding mechanism based on the real-time energy surplus and demand in the local smart grid has been developed in this study to address this concern. With this dual-biding mechanism, dynamic rates are proposed based on both supply and demand. Additionally, both the prosumers and consumers are bidders, namely, the consumers are bidding for economic rates while the prosumers are bidding for the maximum benefits. Market clearing prices (MCPs) are applied to guide dynamic tariff generation and to enable clear, reliable, and safe bidding in the energy blockchain. Biding is a negotiation mechanism and has a well-defined operational mechanism where the negotiation is mediated by an intermediary, which is indeed a set of automated rules rather than a real agent. This is in line with the major objective of smart contract development in blockchain. In this study, the dual-bidding mechanism and transaction rules are set up with an MCP in the smart contract, which can make P2P energy transactions automatically, completing the bidding and forming the clearing price to meet the diverse transaction requirements sufficiently.
In essence, a smart contract is a series of predefined rules or a transaction protocol that is intended to automatically execute, control, or document legally relevant events and actions that occur in the blockchain [29]. Therefore, the preliminary role of a smart contract is to release the dependence on trusted intermediaries, arbitrations and enforcement costs, fraud losses, and the reduction of malicious and accidental exceptions. After generation and validation, smart contracts are broadcast on a blockchain platform such as an Ethereum network and then written into the corresponding contract account where they can be executed automatically as needed. The operation process can be described as follows:
1) System initialization followed by certificate issuing, where registered energy consumers would obtain a certificate from a trusted institution (e.g., a government department). The certificate is used as an ID; however, the consumer’s private information is concealed. The certificate is a kind of permission to join the blockchain network and obtain public/private key pairs, as well as the personal wallet. Here, the account with an energy storage device would keep the information including the wallet address, energy available for trading, account balance, and public/private key pairs.
2) After a consensus is achieved in the energy blockchain network, the smart contract is completed. Then, all network agents obtain access to the smart contract. Each smart contract upholds a set of state variables, including the account addresses of both the seller (accountp) and the buyer (accountb), energy demand (ei), corresponding payment (pi), transaction time (timet), and timestamp in the community. To ensure the successful execution of smart contracts, both the seller and buyer are required to sufficiently deposit to the contract addresses to prevent malicious charging services.
3) After consensus, the Invoke function is called. Then, the smart contract automatically executes energy transactions and financial settlements. The smart contract collects the data from the smart meters of the seller (mp) and buyer (mb) to confirm that the electricity is generated and consumed separately. Finally, the system will periodically update the state ledger of the blockchain, including the balance in household accounts, energy demand, and energy surplus for trading. The execution of smart contracts in the energy blockchain can be coded as follows:
3 Formulation of Models and Algorithms
In this section, metrics indicators, including the production cost and baseline surplus rates of PV electricity, as well as the efficiency of the community energy storage system, are defined in the manner of utility functions to support the assessment of energy blockchain sufficiency. In addition, price trading mechanisms, related algorithms, and attack models are developed to conduct operations and ensure secure energy trading within the local smart grid. Details can be described as follows.
In the smart contract system, a utility function is developed to select transactions from a miner’s transaction pool to form a block, increasing the operational value required to achieve a predetermined output through a blockchain-based network. Suppose the volume of energy surplus from household θi is {r(θi)∈Ω} and the corresponding bidding rate is {s(θi)∈Π}. The contract item set from the sellers can then be defined as {(r(θi),s(θi))|∀θi∈ς}. In the trading process, prosumers with energy surplus take the opportunity to decide whether importing electricity from community storage is needed. For example, r(θi)=0 indicates that a prosumer would not purchase energy but transfer the surplus to community storage for future use; thus, there would be no charge for the operation. In the local energy market, since all consumers would be identified as risk averters whose utility function should be concave and nondecreasing, the utility function can be expressed as
U(θi,r(θi))=V(θi,r(θi))−s(θi).(1)
where V(θi,r(θi)) presents the customer satisfaction obtained from energy trading. The efficiency of energy storage units can be determined by
R(r(θi))=s(θi)−C(θi,r(θi))(2)
As a rational energy storage device would not accept the adverse effects from a particular electricity sales service, it can be concluded with Π={s(θi)|s(θi)≥C(θi,r(θi))}. The cost function C(θi,r(θi)) is composed of the production cost of PV electricity, purchase expenditure and government subsidies, which can be expressed as
C(θi,r(θi))=(cpv−rpv)θir(θi)+pg(1−θi)r(θi)+c0+c1.(3)
where cpv and rpv are the unit costs of PV electricity generation and subsidies, respectively. c0> 0 is the fixed cost, which often includes the trading cost and storage expense. A target bound would be set as 0 ≤ cpv−rpv ≤ pg; thus, the unit rate of PV electricity is less than the grid tariff. c1 is the cost contributed by energy storage device operation and maintenance. The operating rule can be defined as the surplus of PV electricity being delivered to community storage, and only community storage can trade with the national grid. During the process, part of the profit would be spent on system operation and maintenance, and the rest would be transferred to the prosumers who contributed to surplus generation. Then, we have
([∂C(θi,r(θi))]/∂θi)≤0,([∂C(θi,r(θi))]/∂r(θi))≥0 and ([∂2C(θi,r(θi))]/∂r(θi)2)=0(4)
Therefore, the overall efficiency of community energy storage can be assessed by Eq. (5).
R=∑Ii=1τθi(s(θi)−C(θi,r(θi)))(5)
where τθi=[nθi/(∑i∈ℶnθi)] describes the percentage of type-θi consumers over the entire consumer group. nθi is the number of type-θi households. Therefore, the community surplus in energy transactions between consumers and specific consumers can be further defined as the sum of the two utilities, namely,
S(θi,r(θi))=R(r(θi))+U(θi,r(θi))=V(θi,r(θi))−C(θi,r(θi))(6)
Given that prosumer i has a total volume of Yi kWh of PV electricity for trading with an expected rate of xi per kWh, the total volume of electricity included in trading is N kWh, and the total electricity demand in the smart grid is n kWh. Successful transaction matching could be achieved on the condition that the bids placed by consumers are no less than MCP while rates offered by producers are not higher than MCP. Once transaction matching is achieved, both the seller and the buyer can then manage transactions through smart contracts. There are two methods developed to guide trading with the scenario analysis presented in the following section to examine feasibility and desirability:
1) Find the subscript µ that satisfies Zu≤N≤Zu+1, given N=∑Yi, then the liquidation can be determined by
x=αxu+(1−α)xu+1(0≤α≤1)(7)
In the energy trading that occurs in a smart community, if the overall energy demand is K kWh and the biding price is no less than x, namely, the buyer and seller have N kWh electricity with a rate higher than or equal to x. The remaining households have (N−K) kWh electricity with a rate no less than x. The remaining households would have (N−(N−K)=K) kWh electricity with a rate less than x; therefore, consumers with bids no less than the clearing price, all prosumers placed bids lower than the clearing price can match transactions and win the auctions.
2) Find the subscript µ that satisfies Zu≤N≤Zu+1, given N<∑Yi, then the liquidation can be determined by
x=xu+1(8)
Households with insufficient electricity whose bid is no less than the clearing price, as well as households that offer a selling price lower than the clearing price, can be matched for trading.
In energy trading in the smart community, all bids and offers of buyer and seller i equal x, i∈[k1,k2] and k1≤u≤k2; thus,
N≤∑ui=1Yi≤∑k2i=1Yi=∑xi≥xuYi(9)
In method 1, the equality of Eq. (9) can be achieved on the condition that ∑Yi=N, while in method 2, the condition would be N≤∑Yi. Households with insufficient energy need to purchase k1 kWh electricity with a purchase price higher than x. The remaining households have a k2 kWh surplus for trading with a price lower than x. Households with insufficient energy have k3 kWh demand with a purchase price no less than x. The remaining households would sell k4 kWh electricity with a rate no more than x; therefore,
∑k1−1i=1Yi<∑k1−1i=1Yi=Zu≤N(10)
The surplus from households with a rate greater than x is less than (N−k1) kWh, and the surplus from households with a selling price not higher than x is more than (N−(N−k1)=k1) kWh, namely, k1≤k2. Thus, all energy-insufficient households with bids higher than x would win the auction. Similarly, if k2≤k3, all households offered a selling price less than x would win the auction. Only part of the buyers and sellers whose price equals x would be successful in trading. Among those who placed the same bid, the one with the highest demand would win the bid, considering the maximum self-sufficiency of the community. To identify the successful trading ratio on the condition that the price is equal to x, k3 and k4 need to be compared. When k3≤k4, households placed a bid equal to x that would match their demand with other households who have some surplus for trading. If k3=k4, all items with bids equal to x would be involved in energy trading.
The consensus mechanism is an important piece of blockchain that can ensure the consistency and validity of the records made by distributed nodes using a set of specified algorithms while being independent of intermediaries. By applying an internal consensus mechanism, the nodes with mutual trust degrees could be enhanced automatically; therefore, decentralized nodes can operate firmly independent of any mutual trust system built by a third-party organization. In the evolution of blockchain technology, a considerable number of consensus mechanisms have emerged with the efforts of pioneering researchers. Their role is to ensure the consistency of blockchain data and to achieve the characteristics of a trustless model. Popular consensus mechanisms in existing blockchain simulations and practices include PoS, DPoS, and improved algorithms inspired by the former two mechanisms. Consensus algorithms could be either developed for decision-making only, where decentralized group decision-making is the target, or proposed for a full consensus practice, which is a type of “mechanical consensus” [30]. Proof of work (PoW) is widely applied in cryptocurrency mining for validating transactions and mining new tokens through thousands of hashing calculations. The first hash of the block would satisfy the present difficulty of winning the write access to the block. After a successful operation, the miner obtains a reward for the job.
Execution of any smart contract between advanced users would trigger off broadcasting on the network. There are four types of nodes developed in the system: Energy production (prosumers), consumption (both consumers and prosumers), and storage nodes, as well as consensus nodes. During smart contract execution, the consensus nodes receive all those transactions within a timeframe and then sequence the transaction priorities before the transaction day. Here, ineffective trades would be screened out and deactivated. After that, the consensus nodes simulate the execution of intelligent contracts and record the modified state in their native status ledgers. All the effective transactions would be received by each consistency node within a specific timeframe, identified by the timestamp, and bundled as blocks. Each former blockchain includes cryptographic hashes. Finally, all the nodes involved in the blockchain are required to respond to the negotiation process.
With the confirmation of consensus, the settlement module would then be activated. The procedure can be described as follows: Production nodes transmit energy surplus to the storage nodes using the Internet of Things (IoT) transmission method. Simultaneously, consumption nodes send the request for energy demand. The energy storage nodes take responsibility for receiving and validating the contract information, followed by the IoT transmission of energy from fuel cells to the consumption nodes. As tokens are used as the tool for virtual payment, all nodes involved in energy trading are required to register a wallet containing the address used for settlement and information exchange in the blockchain system. The production nodes perform transactions and settlements with storage nodes in the blockchain-based on a present merchant transaction contract, while the consumption nodes perform transactions and settlements with the storage nodes based on a present user transaction contract.
4 Case Study and Discussion of Results
In this section, the proposed energy blockchain is employed to demonstrate the decentralized energy trading within a smart community and to assess the economic-technical merits contributed by the energy blockchain. The objective is to examine the technical feasibility and maximum benefits of local energy trading for both consumers and prosumers promoted by renewable energy harvesting (from roof-top solar PVs) and smart grids. Considering the energy resilience concern from solar PV penetration (which is vulnerable to daylight), the smart grid remains connected with the national grid. However, it is operated on the assumption that there is only wholesale from the national grid to the community storage, while there is no retail for both prosumers and consumers. In addition, the energy surplus from prosumers is assumed to be traded and consumed within the smart community.
4.1 Community Profiles and Case Settings
The community chosen for this study is a residential suburb selected by the local government to showcase modern ecological-economic redevelopment of urban precincts with a master plan based on smart sustainable principles. The community is considered desirable for the study due to the following characteristics:
1) Community buildings are equipped with modern technologies, smart meters, and high PV penetrations, which can be recognized as the trend of future smart communities.
2) Full data access is guaranteed by in-home monitoring systems installed in all the dwellings and a high-performance workstation recording the energy consumption with a per-minute resolution, and
3) Typical demographic features with a holistic data record.
Given the requirements on the simplification, commonality, and flexibility of modeling, improving versatility of the proposed method, as well as predicting consumptions and expenditures under diverse demographics. Three typical household types, young families without children (households 2, 5, and 9), middle-aged families with children (households 4, 6, and 7), and senior families (households 1, 3, 8, and 10), were employed in the analysis. In addition, considering the future trend of smart sustainable community development as well as a comprehensive simulation setting to demonstrate the proposed energy blockchain, all these selected families own solar PV systems, namely, they are all prosumers. The amount of each typical household included in the modeling is scaled down based on the census data. The annual energy profiles of the selected 10 families are summarized in Table 1.
As energy blockchain operation requires a comprehensive understanding of household energy imports, consumption, and exports, the profile data have been refined with resolutions of a quarter and a day, and the changes are illustrated in Figs. 2 and 3, respectively.
Figure 2: Household quarterly electricity consumption
Figure 3: Household daily electricity profiles
Given the ten households involved in the dual bidding for local energy trading with blockchain, the mean values of household daily energy imports and exports are calculated to examine whether a “partition wall” is needed. In other words, to identify the feed-in and import of electricity from the grid to satisfy the energy demand of households but without wasting. As demonstrated in Fig. 4, households 2, 5, 7, 9, and 10 have a daily electricity surplus for trading, while households 1, 3, 4, 6, and 8 might need to purchase electricity from community storage. As the bid x proposed by the ten households would be selected according to a descending order in the dual-biding implementation, the biding order then needs to be updated with high-to-low and presented in Table 2.
Figure 4: Income and expenditure in daily energy trading (AUD)
According to the dual-biding mechanism pricing based on method 1 (Eq. (7)), with the MCP applied in Scenario 1, u=6, and the MCP can be determined by (0.16+0.05α)=0.17. Households 7, 9 and 10, which have an electricity surplus, would match households 1, 3, 4 and 6, which have energy demands, requesting a total of 10.2 kWh electricity for local trading. Households 2, 5 and 8 with surplus or demand would be excluded from the current trading since they could not meet the MCP. Buyers are required to calculate the expenditure and transfer the full payment to the sellers’ accounts. The transaction is then finalized with the transaction moment presented by the node to the moment of mining to generate the block.
Buyers and sellers trade on the principle that “the highest bidder wins”. The process based on the current context can be described as follows: In the first round of trading, household 7 trades with households 1 and 3 with volumes of 3 and 3.5 kWh electricity, respectively. After this round, household 7 still has a 1 kWh surplus, which can be used in the next round of trading with household 4 to satisfy part of its energy demand. Then, household 9 trades with household 4 to fulfill its 1.6 kWh electricity demand in the third round of trading. Finally, household 10 trades with household 6 to fill the 1.1 kWh demand gap. The trading results and node details of Ethereum accounts can be summarized in Table 3.
According to the tariff statistics, the mean value of the electricity rate for national grid imports is 0.37 Australian dollars per kWh (0.37 AUD/kWh), while the feed-in price is 0.06 AUD/kWh. ETH is the unit of Ethereum employed by blockchain as the transaction currency, 1 ETH = 370 AUD in this study.
The results in Table 2 indicate that after energy trading, households 2 and 5 would have a 4.4 kWh surplus, while household 8 still has a shortage of 2.5 kWh. The self-sufficiency of the smart grid is 80.31% (obtained from 10.2/12.7 * 100%). In terms of the trading cost, by comparing the total pretrading ETH with the total post-trading ETH, it is reasonable to conclude that a zero ETH loss was achieved, namely, there is no financial loss associated with energy blockchain trading in this scenario.
Compared to Scenario 1, the values of x4, x5, x6, and x9 are modified according to the different bids placed. The biding order can then be updated with a high-to-low sequence and is presented in Table 4. Although there is no change in the energy surplus and demand gap, the different bids would direct to different trading processes and correspondingly present different results. According to the dual bidding mechanism pricing based on method 2 (Eq. (8)), with the MCP applied, u=6, and the MCP is 0.16. Households 5, 7, 9, and 10, which have electricity surplus, would match with households 1, 3, 4, 6, and 8 for an energy trading of 12.7 kWh in total. Household 2 with surplus could not participate in the trading since it does not meet the clearing price.
Buyers and sellers would remain in trade on the principle of “the highest bidder wins”. The process based on the current context can be described as follows: In the first round of trading, household 5 trades with household 1 with a volume of 3 kWh of electricity. After that, household 7 trades with households 1, 3, 4, and 6 with amounts of 0.5, 3.5, 2.6 and 0.9 kWh, respectively. In the third round, household 9 trades with households 6 and 8 with 0.2 and 1.4 kWh of electricity received, respectively. In the final round, household 10 trades with household 8 with a volume of 1.1 kWh. During energy trading, prosumers can meet the trading requirement by ‘oversell’ and fill the gap by purchasing from others later. The trading results and node details of Ethereum accounts can be summarized in Table 5.
As shown in Table 4, after energy trading, households 1 and 2 would have 0.5 and 2.1 kWh surpluses, respectively, while household 5 has a 0.7 kWh demand gap to be fulfilled. The self-sufficiency of the smart grid is 94.49% (obtained from 12/12.7 * 100%), which is a 14.18% improvement compared to Scenario 1.
Table 6 presents the results of Scenario 3 with the context that both the PV surplus and demand gap are satisfied by direct trading with the centralized national grid. It can be concluded that the conventional energy retail predominated by the centralized grid is often economically undesirable due to the considerable gap between grid feed-in and export rates. However, sufficiency could be fully guaranteed due to the resilience of a national-level distribution network.
By compiling the results in Tables 3, 5 and 6, Fig. 4 demonstrates the daily income and expenditure of households from energy trading considering 100% energy sufficiency (namely, all the surplus is sold, and all the demand is satisfied). Due to the higher electricity import from and export to the national grid (13.7 and 14.6 kWh, respectively), as well as the significant gap between import and feed-in rates (0.37 and 0.06 AUD/kWh, respectively), Scenario 3 is not economically desirable. Direct energy trading in the centralized grid results in 0.876 AUD income at the cost of 4.689 AUD on electricity import from the national grid. Thus, the net benefit position of energy trading in Scenario 1 is –3.813 AUD. Scenario 1 and Scenario 2 have net benefits of −0.7852 and −0.103 AUD, respectively. Although there are still some expenditures on energy imports from the national grid due to the low penetration of solar PVs, these expenses are only 20.52% and 2.7% of the cost in Scenario 3. Further comparison between Scenario 1 and Scenario 2 indicates that Scenario 2 is more economically desirable than Scenario 1, which could be attributed to the increase in the self-sufficiency of the local smart grid, namely, less import from the national grid reduced the overall cost. However, this achievement is at the cost of a more complex energy trading process, with prosumers allowed to ‘oversell’ and purchase later to fill the energy demand gap caused by ‘overselling’. In Scenario 1, the overall income from energy trading is 2.0858 AUD, and the total expense is 2.871 AUD. In Scenario 2, the total income is 2.25 AUD, and the overall expenditure is 2.353 AUD. Therefore, the approach applied in Scenario 2 presents a better solution for P2P energy trading within smart grids; the income of prosumers is increased while the expenditure of consumers is reduced at the same time.
In light of the above, energy blockchain has significant advantages in facilitating smart communities with affordable cleaner energy supplies. The integration of the energy blockchain could reduce the overall expenditure on energy trading from the system perspective and maximize the benefits for both buyers and sellers at the same time. However, the achievement is realized at the cost of high computational requirements and smart grid upgrades. Fortunately, technological advances have been removing the application barriers. Scenarios 1 and 2, both with energy blockchain integration, are employed in this study to investigate the effects of different bidding practices. In the operations, the energy blockchain would guide the bidding toward an optimal solution to maximize both the benefits of buyer and seller, as well as reduce the total loss/cost. This can be achieved by developing and applying a set of fitness and objective functions.
5 Concluding Remarks and Future Studies
Affordable cleaner energy production and distribution is high on the agenda for the sustainable development of human society. Fortunately, the rapid growth of distributed renewable energy penetration has found a way to facilitate smart communities with more sustainable and cleaner energy supplies. However, the decentralized property of rooftop renewable energy harvesting units also introduced large challenges in energy trading and storage for existing centralized grids. In this study, an energy blockchain with a smart contract system is developed to support local energy trading within smart communities to maximize benefits for both prosumers and consumers. By employing a case study on residential precinct operation, a scenario analysis with 10 nodes has been implemented to validate the proposed methodology and examine the potential to promote the economic feasibility of local energy trading. There are three contexts considered: MCP determined by Eq. (7) model (Scenario 1), MCP determined by Eq. (8) model (Scenario 2), and business as usual in a centralized grid (Scenario 3). The results demonstrate that the energy blockchain has great adaptability and outstanding advantages in local energy trading. The comparative study indicates that both the smart community and individuals have obtained maximum benefits from local energy trading in Scenarios 1 and 2. Compared to Scenario 1, Scenario 2 could further improve self-sufficiency and expand revenue by encouraging more nodes to participate in trading and allowing “oversell”. On the other hand, local energy trading can shift the peaks by balancing the demand and production surplus, therefore improving the resilience of the national grid when the energy feed-in requirement is high. It can then reduce the costly grid upgrade work. Although there are only 10 nodes included in the case study to demonstrate the method and validate the models, the proposed approach is fully applicable to large-scale energy trading in smart grids, considering the recent technical advances in computation and communication.
In light of the findings from this study, large-scale scenario analysis with comprehensive considerations of influencing factors, such as computational costs of energy blockchain, energy loss of storage device and grid, energy behaviors of residents, as well as potentials of PV installation and solar access. Need to be further conducted to validate the simulation results and to explore the economic potential of energy blockchain. Therefore, recommendations for future studies can include further scenario analysis on energy blockchain assessment considering embodied elements of overall costs (e.g., lifecycle costs of PVs, smart grid devices, energy storage cells, etc.), computational cost, and technological factors in dynamic smart communities to inform optimal solutions toward affordable, cleaner and sustainable energy supplies in smart communities.
Acknowledgement: None.
Funding Statement: Fundings that permitted this research were granted by Australia CRC for Low Carbon Living through the Project “Integrated Carbon Metrics (ICM)” (RP2007); the National Natural Science Foundation of China (51908064); and the Natural Science Foundation of Hunan Province (2021JJ30717).
Author Contributions: Conceptualization and methodology, Lida Liao; programming and experiment, Mingguan Zhao, Penglong Liang, Meng Li and Xinsheng Dong; writing—original draft preparation, Lida Liao; resources and project administration, Mingguan Zhao, Yang Yang, Hongxia Wang and Zhenhao Zhang; writing—review and editing, Lida Liao. All authors have read and agreed to the published version of the manuscript.
Availability of Data and Materials: Data availability is not applicable to this article as no new data were created or analyzed in this study.
Conflicts of Interest: The authors declare that they have no conflicts of interest to report regarding the present study.
References
1. Frankfurt School (FS) and United Nations Environment Programme (UNEP) Collaboration Center, Bloomberg New Energy Finance (BNEF). Global Trends in Renewable Energy Investment 2018. 2018. Accessed: Jun 5, 2022. [Online]. Available:http://www.iberglobal [Google Scholar]
2. O. O. Yolcan, “World energy outlook and state of renewable energy: 10-year evaluation,” Innov. Green Dev., vol. 2, pp. 100070, 2023. doi: 10.1016/j.igd.2023.100070. [Google Scholar] [CrossRef]
3. M. Willuhn, “New Danish government roadmap displays climate ambition,” PV Magazine, Jun. 2019. Accessed: Jun. 5, 2022. [Online]. Available: https://www.pv-magazine.com/2019/06/27/new-danish-government-roadmap-displays-climate-ambition/ [Google Scholar]
4. B. Huang et al., “Ecological-economic assessment of renewable energy deployment in sustainable built environment,” Renew. Energy, vol. 161, pp. 1328–1340, 2020. doi: 10.1016/j.renene.2020.08.004. [Google Scholar] [CrossRef]
5. A. Richard and G. Peter, “Future pathways for energy networks: A review of international experiences in high income countries,” Renew. Sustain. Energy Rev., vol. 171, pp. 1364–0321, 2023. [Google Scholar]
6. H. Farhangi, “The path of the smart grid,” IEEE Power Energy Mag., vol. 8, no. 1, pp. 18–28, 2009. [Google Scholar]
7. A. Mills et al., Understanding Variability and Uncertainty of Photovoltaics for Integration with the Electric Power System. United States: Lawrence Berkeley National Laboratory, 2009. Accessed: Jun. 5, 2022. [Online]. Available: http://debarel.com/BSB_Library/2009_pv_variability.pdf [Google Scholar]
8. N. Stringer et al., “Fair consumer outcomes in the balance: Data driven analysis of distributed PV curtailment,” Renew. Energy, vol. 173, pp. 972–986, 2021. doi: 10.1016/j.renene.2021.04.020. [Google Scholar] [CrossRef]
9. P. Andreas et al., “Uniform taxation of electricity: Incentives for flexibility and cost redistribution among household categories,” Energy Econ., vol. 127, pp. 107024, 2023. doi: 10.1016/j.eneco.2023.107024. [Google Scholar] [CrossRef]
10. Y. Liu et al., “A practical privacy-preserving data aggregation (3PDA) scheme for smart grid,” IEEE Trans. Ind. Inform., vol. 15, pp. 1767–1774, 2018. [Google Scholar]
11. A. Pieroni et al., “Smarter city: Smart energy grid based on blockchain technology,” Int. J. Adv. Sci. Eng. Inf. Technol., vol. 8, pp. 298–306, 2018. doi: 10.18517/ijaseit.8.1.4954. [Google Scholar] [CrossRef]
12. P. Emrani et al., “Efficient voltage control of low voltage distribution networks using integrated optimized energy management of networked residential multi-energy microgrids,” Appl. Energy, vol. 349, pp. 121391, 2023. doi: 10.1016/j.apenergy.2023.121391. [Google Scholar] [CrossRef]
13. E. Mengelkamp et al., “Designing microgrid energy markets: A case study: The Brooklyn microgrid,” Appl. Energy, vol. 210, pp. 870–880, 2018. doi: 10.1016/j.apenergy.2017.06.054. [Google Scholar] [CrossRef]
14. M. Andoni et al., “Blockchain technology in the energy sector: A systematic review of challenges and opportunities,” Renew. Sustain. Energ. Rev., vol. 100, pp. 143–174, 2019. doi: 10.1016/j.rser.2018.10.014. [Google Scholar] [CrossRef]
15. L. Wu et al., “Democratic centralism: A hybrid blockchain architecture and its applications in energy internet,” in 2017 IEEE Int. Conf. Energy Internet (ICEI), Beijing, China, Apr. 2017, pp. 176–181. [Google Scholar]
16. V. Brilliantova and T. W. Thurner, “Blockchain and the future of energy,” Technol. Soc., vol. 57, pp. 38–45, 2019. doi: 10.1016/j.techsoc.2018.11.001. [Google Scholar] [CrossRef]
17. R. Alvaro-Hermana et al., “Peer to peer energy trading with electric vehicles,” IEEE Intell. Transp. Syst. Mag., vol. 8, no. 3, pp. 33–44, 2016. doi: 10.1109/MITS.2016.2573178. [Google Scholar] [CrossRef]
18. C. Long et al., “Peer-to-peer energy sharing through a two-stage aggregated battery control in a community microgrid,” Appl. Energy, vol. 226, pp. 261–276, 2018. doi: 10.1016/j.apenergy.2018.05.097. [Google Scholar] [CrossRef]
19. R. Kamel, A. Chaouachi, and K. Nagasaka, “Carbon emissions reduction and power losses saving besides voltage profiles improvement using microgrids,” Low Carbon Econ., vol. 1, no. 1, pp. 1–7, 2010. doi: 10.4236/lce.2010.11001. [Google Scholar] [CrossRef]
20. D. Efanov and P. Roschin, “The all-pervasiveness of the blockchain technology,” Procedia Comput. Sci., vol. 123, pp. 116–121, 2018. doi: 10.1016/j.procs.2018.01.019. [Google Scholar] [CrossRef]
21. K. Hojčková, B. Sandén, and H. Ahlborg, “Three electricity futures: Monitoring the emergence of alternative system architectures,” Futures, vol. 98, pp. 72–89, 2018. doi: 10.1016/j.futures.2017.12.004. [Google Scholar] [CrossRef]
22. K. Panetta, “Top trends in the gartner hype cycle for emerging technologies,” 2017. Accessed: Oct. 7, 2021. [Online]. Available: www.gartner.com/smarterwithgartner/top-trends-in-the-gartner-hype-cycle-for-emerging-technologies-2017/. [Google Scholar]
23. A. Miglani et al., “Blockchain for internet of energy management: Review, solutions, and challenges,” Comput. Common, vol. 151, pp. 395–418, 2020. doi: 10.1016/j.comcom.2020.01.014. [Google Scholar] [CrossRef]
24. M. Mihaylov et al., “NRG-X-change—A novel mechanism for trading of renewable energy in smart grids,” in Proc. 3rd Int. Conf. Smart Grids Green IT Syst., Barcelona, Spain, Apr. 4–5, 2014, pp. 101–106. [Google Scholar]
25. V. Reijnders et al., “A hybrid electricity pricing mechanism for joint system optimization and social acceptance within energy communities,” Energy Rep., vol. 8, pp. 13281–13292, 2022. doi: 10.1016/j.egyr.2022.10.021. [Google Scholar] [CrossRef]
26. R. Lu, S. Hong, and X. Zhang, “A dynamic pricing demand response algorithm for smart grid: Reinforcement learning approach,” Appl. Energy, vol. 220, no. 15, pp. 220–230, 2018. [Google Scholar]
27. A. Osman, “A novel big data analytics framework for smart cities,” Future Gener. Comput. Syst., vol. 91, pp. 620–633, 2019. doi: 10.1016/j.future.2018.06.046. [Google Scholar] [CrossRef]
28. J. P. Cabrera-Sánchez and Á.F. Villarejo-Ramos, “Acceptance and use of big data techniques in services companies,” J. Retail. Consum. Serv., vol. 52, pp. 101888, 2020. doi: 10.1016/j.jretconser.2019.101888. [Google Scholar] [CrossRef]
29. T. Don and T. Alex, “Blockchain revolution: How the technology behind bitcoin is changing money,” in Business, and the World, USA: Penguin Publishing Group, 2016. [Google Scholar]
30. N. Szabo, “Formalizing and securing relationships on public networks,” First Monday, vol. 2, no. 9, 1997. doi: 10.5210/fm.v2i9.548. [Google Scholar] [CrossRef]
Cite This Article
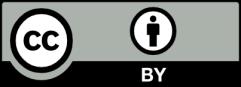
This work is licensed under a Creative Commons Attribution 4.0 International License , which permits unrestricted use, distribution, and reproduction in any medium, provided the original work is properly cited.