Open Access
REVIEW
A Research Progress of CO2-Responsive Plugging Channeling Gels
1 Key Laboratory of Drilling and Production Engineering for Oil and Gas, Yangtze University, Wuhan, 430100, China
2 School of Petroleum Engineering, Yangtze University, Wuhan, 430100, China
* Corresponding Author: Jianxin Liu. Email:
(This article belongs to the Special Issue: Integrated Geology-Engineering Simulation and Optimizationfor Unconventional Oil and Gas Reservoirs)
Energy Engineering 2024, 121(7), 1759-1780. https://doi.org/10.32604/ee.2024.048536
Received 11 December 2023; Accepted 30 January 2024; Issue published 11 June 2024
Abstract
In the heterogeneous reservoirs, CO2 flooding easily leads to CO2 gas channeling, which can seriously affect sweeping efficiency and reduce oil recovery. After thoroughly investigating the advantages and shortcomings of various CO2 plugging technologies, this paper focuses on the feasibility of improving conventional water-alternating gas (WAG) through CO2-responsive gel materials. Based on the different chemical reaction mechanisms between the unique chemical structure and CO2, changes in the material’s physical and chemical properties can respond to CO2. The feasibility of utilizing these property changes for CO2-responsive plugging is explored. Various CO2-responsive gels and gel nanoparticles have been extensively researched in different fields, such as energy, medicine, and biology. This paper surveys the molecular structures, chemical compositions, response mechanisms, and changes of these CO2-responsive gels, aiming to draw insights into the carbon dioxide-enhanced oil recovery (CO2-EOR) field. Finally, the key issues and future development direction of CO2-responsive plugging gels were analyzed.Keywords
The carbon capture, utilization, and storage (CCUS) are the most practical and feasible carbon reduction technologies. CO2 possesses unique physicochemical properties, is widely available, easily accessible, and safe. Currently, it is extensively used for EOR, significantly improving the oil recovery of heavy oil reservoirs and low-permeability oil fields. Therefore, CCUS-EOR technologies, represented by CO2 flooding, have broad prospects for both carbon reduction and resource utilization [1]. Research indicates that due to CO2’s low minimum miscibility pressure (MMP), it is easily miscible with crude oil, causing it to expand, reducing capillary and flow resistance. Consequently, this significantly enhances the flow capacity of crude oil [2].
Gas channeling is a significant issue in the CO2 flooding process, leading to a decrease in CO2 sweep efficiency and a noticeable reduction in oil recovery. See Fig. 1a, the main cause of gas channeling is the severe heterogeneity in the reservoir. Injected CO2 is prone to channeling along the high seepage zone such as fractures and large-pore channels, greatly diminishing the efficiency of CO2 sweep. Because crude oil has a much higher viscosity than CO2, its mobility differs significantly, making it susceptible to viscous fingering, which hinders efficient oil displacement [3].
Figure 1: CO2 gas channeling
Currently, there are two main methods to inhibit CO2 channeling and enhance displacement phase sweeping efficiency. See Fig. 1b, the first method involves plugging highly permeable channels to suppress the advancement of CO2 along preferential pathways, utilizing techniques such as gel plugging and foam plugging. The second method involves increasing the viscosity of the displacing phase to reduce the mobility ratio, employing techniques like WAG and CO2 thickening.
1.1 Current Research Status of CO2 Plugging Channeling Technologies
WAG technology is a process that uses alternating slugs by injecting gas and water, which enhances gas sweep efficiency and minimizes the required gas volume [4]. The CO2-WAG injection process is seen in Fig. 2 [5]. WAG combines the advantages of water and gas injection, where water improves macroscopic sweep efficiency, and gas, due to its high permeability, enhances microscopic sweep efficiency. Additionally, CO2 has a relatively low minimum miscibility pressure (MMP) and is easily miscible with light crude oil. Injecting water increases reservoir pressure above the MMP of CO2, making it more soluble in crude oil and reducing the relative permeability of CO2 [6–8]. As early as the 20th century, CO2-WAG injection technology has been successfully applied in some sandstone reservoirs in the United States. References [9–11] reported the performance of the CO2-WAG project in the U.S. Gulf coast reservoir. These projects have achieved success, the oil production has significantly improved. However, CO2-WAG technology also has some drawbacks. Firstly, due to the higher density and viscosity of water compared to CO2, water tends to preferentially fill the high permeability layers in the reservoir, forcing CO2 to change its flow direction towards the low permeability layers, known as gravity segregation. Secondly, the presence of CO2 makes the reservoir acidic, leading to corrosion of pipelines during the injection process and precipitation of polar substances such as asphaltene that are insoluble in crude oil, causing blockages in flow channels [12]. Thirdly, water entering the formation increases the water saturation near the wellbore, increasing capillary resistance at the oil-water interface in the pores, a shielding effect that undermines the efficiency of microscopic displacement [13,14]. The presence of the Jamin effect can lead to water blocking [15], which is unfavorable for oil recovery. Lastly, WAG process design and optimization parameters are complex, with gas-water ratio, injection pattern, injection rate, injection volume, and slug size all impacting the operational effectiveness [16].
Figure 2: CO2-WAG injection process under mixed-phase pressure (modified from Dai et al. [5])
1.1.2 CO2 Thickening Technology
CO2 thickening technology utilizes polymers to increase the viscosity of CO2, thereby reducing its mobility and weakening the effect of viscous fingering. As the density of CO2 is much lower than that of formation water and crude oil, using CO2 as a displacing phase can easily lead to phenomena such as gravity segregation and viscous fingering, resulting in a significant amount of residual oil. see Fig. 3 below [17]. Currently, there are two main methods for directly thickening CO2. One approach involves using organic solvents to increase the solubility of conventional thickening agent compounds in the CO2 phase. The feasibility of this method depends on the ability of the polymer/solvent combination to increase the viscosity of CO2. Another method involves using additives with both hydrophilic and hydrophobic properties. Polymer segments can form supramolecular solution structures under binding conditions [18]. Polymers currently used in CO2 thickening technology mainly include fluorinated polymers, silicone polymers, alkanes polymers, etc. [19,20]. Silicone polymers, fluoropolymers and alkanes polymers have achieved the thickening effect [21–24]. However, low molecular weight alkanes polymers, including poly (vinyl ethyl ether) (PVEE) and poly-1-decene (P1D), it has a little CO2 thickening [25,26].
Figure 3: Illustration of challenges during CO2 injection such as viscous fingering and gravity override (modified from Pal et al. [17])
However, direct CO2 thickening technology also faces significant challenges. Firstly, although fluorinated and silicone polymers can achieve a certain thickening effect, silicone polymers have poor solubility and generally require a large amount of co-solvent, need higher temperature and pressure, making them challenging for widespread use. Secondly, fluorinated polymers have issues of high toxicity and poor degradability, which can have adverse environmental effects and are not conducive to practical production. Thirdly, alkanes polymers, despite having good CO2 solubility, face the problem of decreasing solubility with increasing carbon chain length, resulting in high costs [19]. Therefore, developing environmentally friendly, cost-effective, non-toxic, and highly effective thickening agents remains a significant challenge.
Gels in formation water can increase viscosity or swell, thereby plugging high-permeability layers and altering the flow direction of gases, enhancing the sweep efficiency of the displacing phase. It is mainly divided into two types: one is in-situ gel formation, composed of monomers or polymers, cross-linking agents, and other additives in a solution. When injected into the formation, it forms a gel under reservoir conditions, plugging the high-permeability layers. The other type is ground pre-crosslinked gels which mainly include pre-crosslinked particle gels (PPGs), polymer microspheres, and colloidal dispersion gels (CDGs) [27]. Under reservoir conditions, these particles gradually swell and plug the high-permeability Bai et al. [28]. Lantz et al. [29] reported the use of partially hydrolyzed polyacrylamide (HPAM) and Cr3+ cross-linking agent to treat some oil reservoirs, results showed the oil recovery increased. Zhou et al. [30] used free radical polymerization to prepare an acid-resistant pre-formed particle gel (AR-PPG). Results showed that AR-PPG had a swelling ratio of 1.41 in acidic environments. Compared to traditional PPG, AR-PPG exhibited better swelling performance, shear resistance, and viscoelasticity in acidic environments, achieving a plugging rate of 70.8% in CO2-driven low-permeability micro fractured cores.
Traditional gel plugging technology is mainly used for water plugging and has poor adaptability to CO2 channeling. For example, conventional in-situ gels have poor acid resistance, fast cross-linking under acidic conditions, high dehydration rates, and commonly used cross-linking agents are environmentally toxic [2]. Regarding traditional preformed particles, the matching relationship between particle size and formation pore throats directly affects the injectivity of the plugging agents. Acidic and high-temperature, high-salinity environments can accelerate particle degradation and dehydration [31]. The degradation products may contain ketone, aldehyde, carboxylic ester groups, and monomer units, which can adsorb onto mineral surfaces, causing harm to the reservoir [32] and they are poorly adapted to low-permeability reservoirs [16]. Although polymers with temperature, salt, and acid resistance have been developed, their high costs make them economically unfeasible for widespread use.
1.1.4 Foam Plugging Technology
Foam, with greater effective viscosity than gas, can alleviate gravity override and viscous fingering during gas injection, and by increasing flow resistance, it prompts the injected fluid to divert from high-permeability layers to low-permeability layers [33]. The improvement of the long-term stability of CO2 foams has been the subject of extensive research efforts. Such approaches include the use of surfactants (cationic, anionic, non-ionic, and zwitterionic), viscoelastic surfactants (VES), polymers, and nanoparticles (NPs) [34]. There are generally three methods of foam injection: generating foam externally and injecting it into porous media, injecting surfactant and CO2 simultaneously into porous media to form foam, and alternating injection of surfactant and CO2 (SAG).
Using surfacants to control the mobility of CO2 Studies showed that foam could enhance gas displacement by reducing permeability, altering mobility ratio, and preventing channeling [35–37]. The reference [38–40] have investigated the utilization of nanoparticles for enhancing foam stability,the stabilization time is evidently extended. Additionally, foam plugging technologies have also been successfully applied in some North American oilfields. Some CO2 foam tests that all decreased CO2 injection capacity, effectively altering the mobility of CO2 [41,42]. However, foam plugging technology also faces the following challenges:
1. Poor stability and oil resistance of foam systems, leading to a short effective period;
2. Poor injectivity due to additional resistance during foam injection caused by gas Jamin effect, increasing pump pressure and construction difficulty;
3. High costs due to the decreased actual injection volume caused by surfactant adsorption during the oil displacement process, requiring continuous addition of surfactant to ensure overall construction effectiveness, thus increasing economic costs;
4. Cationic surfactants, while exhibiting good foaming properties in the acidic environment induced by CO2 flooding, are mainly nitrogen-containing amine derivatives, posing toxicity risks to humans and the environment. Currently, many nanoparticles have been developed to enhance the stability of foam, which can delay bubble coalescence and improve foam stability. Reference [43] developed a CO2 foam system suitable for high-temperature and high-salinity conditions by adding 1% nano-silica to the system. The results indicate that the half-life of the foam was extended by 14 min. However, there is a problem with nanoparticle aggregation, which is not conducive to achieving the maximum performance of nanoparticles [16]. Therefore, there are certain challenges and difficulties in developing highly effective surfactants for CO2 flooding.
This article first investigates the laboratory research and field applications of various CO2-plugging technologies. After analyzing the advantages and disadvantages of existing plugging technologies, the article proposes technical measures using CO2-responsive gel materials for selectively plugging CO2. Subsequently, Based on the different chemical reaction mechanisms between the unique chemical structure and CO2, the article explores the mechanisms behind CO2-responsive plugging. Finally, the research surveys the current applications of various CO2-responsive gels and analyzes their potential applications in the field of improving crude oil recovery by preventing CO2 channeling.
CO2-responsive gel materials are environmentally sensitive intelligent plugging materials. Applying them in the development of oil-gas fields holds the potential to address the shortcomings of current CO2 plugging technologies, making a significant contribution to CO2 sequestration and resource utilization.
2.1 Reaction Mechanism of CO2 with Specific Functional Groups
The CO2 response mechanism mainly refers to the reversible changes in the properties of compounds after the introduction and removal of carbon dioxide in the system. This is achieved by involving only the introduction and release of inert gases such as CO2 and N2 in the process, thereby avoiding the generation of by-products and system contamination. Compounds with this kind of stimuli-responsive behavior controlled by a “green” switch have broad applications in sensors, surface adhesives, and drug delivery carriers. These compounds contain specific functional groups that can react with CO2 [44], primarily including amine groups, carboxyl groups, imine groups, guanidine groups, etc. The reaction mechanisms can generally be classified into the following four types, see Figs. 4–8 below.
Figure 4: Reaction mechanism of tertiary amine group with CO2
Figure 5: Reaction mechanism of carboxylic ion with CO2
Figure 6: Reaction mechanism of amidine with CO2
Figure 7: Reaction mechanism of guanidine with CO2
Figure 8: Reaction mechanism of primary amine with CO2
(a) Tertiary amine reacts with CO2 and water to form bicarbonate, see Fig. 4
Due to the basicity and water solubility of tertiary amines, upon the introduction of CO2 under acidic conditions, the amine groups of tertiary amines will be protonated by a large number of protons produced from the ionization of carbonic acid. As the ionization degree of tertiary amines increases, their hydrophilic ability strengthens. When inert gases such as N2, Ar, etc., are introduced into the system to remove CO2, this reaction will reverse, leading to a decrease in the hydrophilic properties of tertiary amines. Therefore, cationic hydrogels containing tertiary amine groups exhibit a lower degree of swelling when not in contact with CO2, allowing them to smoothly enter high-permeability layers. Upon contact with infiltrating CO2, they moderately swell to achieve plugging.
(b) Carboxylic ion reacts with CO2 and water to form carboxylic acid, see Fig. 5
Carboxylic ions exhibit low dissociation under acidic conditions when CO2 is introduced, rendering them electrically neutral. The O-H bond in the carboxyl group, however, possesses strong polarity and can form hydrogen bonds with other carboxylic acid groups. These dual effects collectively induce volume contraction in gels containing carboxylic acid groups. When the system’s pH is higher than the pKa of carbonic acid (6.35), the proton dissociation capacity of carboxylic acid groups significantly increases, transforming into carboxylate ions. This enhances the hydrophilicity of the gel, causing the gel network to swell, and resulting in a noticeable increase in volume. Therefore, anionic hydrogels containing carboxylic acid groups are only suitable for plugging under alkaline conditions and are not suitable for plugging CO2.
(c) Amidine/Guanidine reacts with CO2 and water to form the corresponding amidine or guanidine salt, see Figs. 6, 7
The secondary amino group in the imine or guanidine contains lone pair electrons, which, upon reacting with CO2 and water, form a Lewis acid-base pair with the generated carbonic acid. This triggers an electrophilic reaction, producing the corresponding imine salt or guanidine salt. The significantly increased hydrophilicity induces water absorption and swelling in gels containing imine or guanidine groups, achieving plugging. When the gel is not in contact with CO2, deprotonation occurs, leading to volume contraction, which helps improve the injectivity of the gel.
(d) Primary amines react with CO2 to form carbamates, see Fig. 8
At room temperature and pressure, CO2 can bind with two molecules of primary amines, forming the anionic aminomethanoate and protonated cationic primary amine. These two entities form a salt through electrostatic interactions. When the system is heated, the aminomethanoate salt decomposes, releasing CO2 gas from the system, and allowing the system to reversibly return to its initial state. Therefore, primary amines can block CO2 infiltration channels by generating precipitates.
2.2 Gel Plugging Channeling Mechanism
CO2-responsive plugging shares similarities with non-responsive plugging. Both involve physicochemical changes, such as expansion, thickening, adsorption, solidification, etc., occurring in underground high-permeability layers to block advantageous pathways for gas channeling. However, there are clear differences. The conventional non-responsive gel change process is typically triggered by reservoir temperature, primarily plugging water or gas fluid channeling pathways. In contrast, the responsive gel change process is stimulated by gas channeling of CO2, selectively plugging CO2 infiltration pathways. Therefore, gels with CO2 responsiveness are more suitable for selectively plugging CO2 gas channeling under injection conditions.
Gel particle water absorption and swelling refer to the diffusion of water molecules into the three-dimensional network inside gel particles upon contact with water. This causes the original polymer chains to transition from coiled to extended, increasing the system’s water content and significantly expanding its volume.
The swelling performance of gel particles (including swelling rate and swelling ratio) depends mainly on the hydrophilic properties of polymer segments, gel size, reservoir temperature, mineralization, and other factors. The slow expansion of gel particles effectively resolves the contradiction between injectivity and plugging performance. Currently used particle-sized gels and polymer microspheres operate on this plugging mechanism [45–48].
2.2.2 Increasing Viscosity Mechanism
Generally, water-soluble polymer viscosity is low, resulting in weak plugging capabilities. By adding a crosslinking agent to low-concentration polymer solutions that delays the crosslinking reaction (such as chromium ions, phenol-formaldehyde resins, etc.,), or adding initiators to monomer solutions, a gel with higher viscoelasticity can slowly undergo sol-gel transition underground at high temperatures. This forms a gel with higher viscosity and plugs the high-permeability layer, forcing injected water and gas into low-permeability layer pores, thereby improving the displacement phase’s sweep efficiency. Similar to gel particles, this delayed thickening characteristic effectively addresses the contradiction between the injectivity and plugging performance of plugging agents. Currently used chromium gels, phenol-formaldehyde resin gels, in-situ gels, etc., operate on this plugging mechanism [49–51].
Some gel materials or surfactants contain a large number of polar groups, allowing for the formation of physical or chemical adsorption on the pore surfaces of high-permeability layers through electrostatic interactions, chemical reactions, etc. This reduces pore size, increases flow resistance of the displacing phase, or alters the wettability of the rock, thereby promoting injected water and gas into low-permeability layers, expanding sweep efficiency. However, this adsorption action inevitably causes short-term or permanent damage to the reservoir. Currently used cationic polyacrylamides, cationic surfactants, etc., operate on this mechanism [52].
In summary, plugging materials, through physicochemical changes such as expansion, thickening, adsorption, etc., in high-permeability layers experiencing fluid flow, form plugging zones with certain pressure-holding capabilities. This is the primary means of inhibiting the infiltration of the displacing phase and expanding sweep efficiency. However, these plugging materials generally lack selectivity for CO2. Some plugging materials, such as polyacrylamide gels, may exhibit unfavorable changes in performance, such as viscosity reduction and volume contraction, under acidic conditions. Therefore, traditional plugging materials may not necessarily be suitable for selectively plugging CO2 infiltration.
Gel materials, due to their high viscosity or mechanical strength and strong deformability, are commonly used plugging agents in water and gas control in oil and gas extraction. To achieve selective CO2 plugging by gels, CO2-responsive functional groups are introduced into polymer molecules. Based on the reaction mechanism between CO2-responsive functional groups and CO2, various CO2-responsive gel materials have been developed in different application areas. In this article, we investigate several types of CO2-responsive gels based on cross-linking mechanisms, including chemical crosslink and physical crosslink.
3.1.1 Double-Bond Crosslink Gel
Double-bond crosslink agents are generally added during polymer preparation and participate in monomer copolymerization. The most common double-bond crosslink agents generally can be divided into two categories: N, N-methylene bisacrylamide (MBA), and N, N’-bis(acryloyl)cystamine (BACy). MBA is known for its advantages of structural stability, easy preparation, and convenient cross-linking degree adjustment [53]. Therefore, it is widely used in oil and gas development, preparation of photosensitive materials, and separation of biopolymer compounds (proteins, peptides, nucleic acids), etc. [54–57]. However, it is not convenient for underground use and is generally employed in the preparation of pre-crosslinked gel particles. The other double-bond crosslink agent is BACy, It is often used as a crosslinking agent to adsorb dyes and sewage materials with strong stability, incompatible with strong oxidants, and insoluble in water [58,59].
This article investigates CO2-responsive gel particles of different sizes. Gel particles are colloidal gel particles composed of chemically cross-linked three-dimensional polymer networks. Based on their different sizes, they can be classified into nano-gels, micro-gels, and large particle gels. Nanogel (particles with sizes <100 nm), microgel (particle size between 100 nm and 100 mm), and large particle gel (particles with sizes >100 μm) [60]. These are suitable for plugging different-sized fluid flow channels.
Gel particles can swell under certain conditions, and their swelling performance is influenced by cross-linking density, elasticity, and the nature of the polymer chain segments forming the gel. Compared to large gels, gel particles have significant advantages. Firstly, they have a larger surface area, resulting in a faster response compared to large gels. Secondly, the viscosity of micro-gel suspensions depends mainly on the viscosity of the dispersed phase and the concentration of micro-gels, making their viscosity much lower than that of soft solid-like large gels. Similar to conventional micro-gels, responsive micro-gels are typically synthesized through precipitation polymerization and inverse emulsion polymerization and have been widely used in areas such as dye adsorption, biomedical applications, and enhanced oil recovery [60]. The applications of double-bond crosslink gels are seen in Table 1.
3.1.2 The Applications of Other Types CO2-Responsive Microgels
Based on the reaction mechanism of different functional groups to achieve reversible volume expansion and contraction through alternating treatment with CO2 and N2 and play the properties of the materials [61–65].
Rabiee et al. [61] developed cationic microgels of 2-(diethylamino) ethyl methacrylate (DEAEMA) and 2-(dimethylamino) ethyl methacrylate microgels (DMAEMA). As the CO2 will induce the tertiary amine matrix derivatization, enhance the hydrophilicity, and lead to the expansion of microgel due to the effect of electrostatic repulsion. Hence, the microgel could absorb water and dehydrate through CO2/N2 bubbling and Water recovery by 50% can be achieved for these microgels. But N2 bubbling will deprotonate until the microgel is uncharged and appears as volume shrinkage. Furthermore, CO2/N2 bubbling will change the surface charge of microgels, which will affect the swelling/shrinking properties and water absorption/dehydration capacity of microgels.
Xu et al. [62] synthesized CO2-responsive waterborne polyurethane-polydimethylaminoethyl methacrylate (WPU-co-PDM) emulsion by free radical copolymerization. The particle size of the emulsion increased from 80 nm at 25°C to 180 nm at 50°C, after CO2 bubbling into the emulsion, Upon bubbling with N2, the particle size returned to the original size, and it showed CO2 responsiveness. As the temperature increased, the ionized quaternary ammonium salt underwent “deprotonation,” transforming hydrophilic quaternary ammonium cations into hydrophobic tertiary amine structures. Therefore, emulsification could be achieved by bubbling CO2 or cooling, while de-emulsification could be achieved by bubbling N2 and heating.
Shieh et al. [63] used multi-responsive amphiphilic block copolymers containing methacrylic acid (MAA) and the emulsifiers 2-dimethylaminoethyl methacrylate (DMAEMA), two-way CO2 stimuli-responsive PMMA latex particles were prepared via aqueous emulsion polymerization. By tuning the length of the PDMAEMA block in the copolymer the PMMA-surfaced latex particles can exhibit a two-way CO2 response between dispersion and precipitation at acidic (pH = 2) and alkaline (pH = 12).
In 2023, Al Akoumy et al. [64] synthesized core-shell nanoparticles as gas-controlled reduction catalysts for 4-nitrophenol (4-NP) and nitrobenzene (NB). PDEAEMA, a CO2-responsive crosslinked polymer, adjusted the catalytic reaction rate through the reversible switching of CO2/N2 bubbling, For the reduction of 4-NP, it is possible to turn on and turn off the reaction through alternating bubbling of the two gases. CO2 can promote a faster reaction of 4-nitrophenol (4-NP), while N2 facilitates the faster reaction of nitrobenzene (NB) for a mixture of 4-NP and NB.
Mu et al. [65] synthesized a series of CO2-responsive microspheres with (PAM)/polydimethylaminopropyl methylacrylamide (PDMAPMA) interpenetrating (IPN) structures by reverse-phase suspension polymerization. With increasing DMAPMA concentration, the particle morphology transitioned from IPN to a core-shell structure. These particles can undergo reversible volume expansion and contraction through alternating treatment with CO2 and N2. In addition, non-agglomerated responsive particles of different compositions can be prepared by changing the cross-linking degree. The maximum response relative swelling volume (RSV) can reach 11.6% when the PDMAPMA loading is at 87%.
Qi et al. [66] used free radical copolymerization to create a responsive polyacrylonitrile-co-poly (N, N-diethylaminoethyl methacrylate) (PAN-co-PDEAEMA) copolymer, and then electrospun it into nanofiber membranes for selective oil-water separation. Nanofiber membranes have excellent properties such as large specific surface area and high porosity. When CO2 was bubbled, the tertiary amine group was protonated, enhancing the hydrophilicity of PDEAEMA and causing molecular chain extension. Water can easily pass through the nanofiber membrane, while the oil is retained by the nanofiber membrane. In contrast, after the N2 simulation, the tertiary amine group was deprotonated, the hydrophobicity of PDEAEMA was enhanced and the molecular chain collapsed, which effectively changed the wettability of the nanofiber membrane surface and achieved the purpose of oil-water separation.
3.1.3 Metallic Ions Crosslink Gel
HPAM [67] is widely used for profile control and water shutoff, mainly involving the formation of gels through coordination bonds between multivalent metal ions and carboxyl groups in HPAM. Common metal ions include Al3+, Fe3+, Cr3+, Zr4+, Ti4+, among others, and these multivalent ions can exist in the form of simple inorganic ions or soluble coordination compounds. The rate and extent of the reaction depend on the ligand within the crosslinking agent’s molecular structure [68,69]. If carboxyl groups of metal ion complexes further bind with another carboxyl group on the same polymer chain, intramolecular crosslinking occurs.
Mo et al. [68] reported delaying the crosslinking of HPAM with Cr3+ by adding citric acid. This was effective because the ionization of HPAM is influenced by the pH of the system, and adjusting the pH of the buffering solution can control the release of CO2, thereby delaying crosslinking.
Hadi Mosleh et al. [69] evaluated the PAM/Zr+ gel system under constant pressure of 50 psi CO2 injection. The results showed that the polymer gel (polymer concentration: 1000 ppm and crosslinker concentration: 15 ppm) could reduce the CO2 permeability by 99%. Hence, low pH may reduce the crosslinking rate and push the low-viscosity polymer slug into the remote well zone to achieve deep plugging, thereby increasing the CO2 sweep efficiency.
Cross-linking mechanism of most organic crosslink gels is typically divided into two categories: One is the preparation of phenolic resin by condensation of polyhydroxyresorcinol. Further polycondensation between the phenolic resin and polyacrylamide can form a bulk polymer, thereby improving the stability and strength of the gel. The other is to form in situ gel with polyvinylimide (PEI) as a crosslinking agent under specific conditions. Among them, AM and monomers containing the tertiary amine group are used to form a gel in situ through free radical copolymerization [70,71].
Li et al. [70] reported a CO2-responsive gel system with polyacrylamide as the main agent, and resorcinol as the crosslinking agent. CO2 can create an acidic environment in the reservoir, leading to the release of formaldehyde from methenamine under acidic conditions. Polyhydroxymethyl resorcinol can be formed through the reaction between formaldehyde and resorcinol. The phenolic resin was formed via polyhydroxy resorcinol condensation. Further polycondensation between phenolic resin and polyacrylamide can form a bulk polymer, which improves the stability and strength of the gel. This effectively plugs highly permeable zones, improving CO2 sweep efficiency and oil recovery.
Wu et al. [71] used AM and DMAPMA as monomers and PEI as a crosslinking agent to synthesize a delayed swelling CO2-responsive hydrogel through free radical copolymerization, which contains tertiary amine group and crosslinking group (-NH). The hydrogel exhibited excellent swelling properties, expanding tenfold at pH ~4.5 and twofold at neutral pH ~7. Additionally, the gel remained stable for over 30 days at 80°C without dehydration, addressing CO2 channeling issues.
Physical crosslink gel can also be called “supramolecular gels” or “ self-assembled gels”, and are crucial soft materials with unique advantages. Unlike traditional chemical gels with covalent bond crosslinking, which form a network structure via inferior non-covalent interactions and have the property of reversible crosslinking [72]. Supramolecular gels are shape memory gels whose temporary shape is stabilized by reversible crosslinking. Based on the triggering mechanisms of the shape memory effect, it can be divided into two types: supramolecular interactions and dynamic covalent bonds. This kind of non-covalent bond interaction includes hydrogen bond interactions, π–π interactions, electrostatic interactions, van der Waals force interactions, dipole-dipole interactions, metal-ligand coordination, host-guest interactions, etc. [73–75]. They have found widespread applications in biomedicine, including drug delivery, gene transfection, protein delivery, biomedical imaging and diagnosis, tissue engineering, and biomimetic chemistry [76]. Self-assembly gels generally come in two forms: micromolecules self-assembly and macromolecules self-assembly [77].
The most significant feature of physical crosslinked gels is that their “sol-gel transition” is reversible under external environmental stimuli. This characteristic is particularly advantageous in the field of CO2 plugging, allowing injection in a low-viscosity sol state that gelates to block gas channels upon contact with CO2. The broken gel can self-repair, making it a new generation of “smart” gel materials capable of automatically identifying and selectively plugging gas channels.
3.2.1 Micromolecule Self-Assembly
Some surfactant systems can form wormlike micelles (WLMs) by changing the critical micelle concentration (cmc), thereby undergoing gelation. This is the main mechanism of small molecule self-assembly gels. This category of surfactants includes cationic surfactants, anionic surfactants, non-ionic surfactants, different types of combined surfactants, zwitterionic surfactants, mixed systems of surfactants and auxiliaries, mixed systems of surfactants and solubilizing salts, Gemini surfactants, oligo-surfactants, block copolymers, etc. [78]. Micromolecule self-assembly gels have the advantages of easy preparation, reversible and controllable rheology, economy, and environmental protection, and have been widely used in many fields.
Dai et al. [79] prepared a novel CO2-responsive plugging channeling system by using small molecular amine compounds and a modified long-chain alkyl anionic surfactant. Before contacting CO2, the system was a low-viscosity fluid and after contacting CO2, the primary amine molecules were protonated, bridging two anionic surfactant molecules through electrostatic forces to self-assemble into WLM, resulting in a significant increase in viscosity and viscoelasticity. Rock core displacement experiments demonstrated a plugging efficiency exceeding 90%, effectively plugging CO2 channels.
Shen et al. [80] selected a long-chain surfactant N, N-Dimethyl erucamide tertiary amine (DMETA) from 10 kinds of surfactants containing tertiary amine groups, it has a reversible CO2/N2 bubbling response. After CO2 is bubbled, tertiary amine groups are protonated, the electrostatic repulsion is enhanced, and micelles self-assemble into a network structure. The viscosity of the system is increased to 605700 mPa·s, displaying viscoelastic behavior. This property enabled plugging high-permeability layers, improving sweep efficiency, and increasing crude oil recovery by 21.7%. When N2 is bubbled, the system returns to neutral, the tertiary amine group deprotonated, and the viscosity eventually decreases. Thus, it can be used to address the issue of gas channeling during CO2 flooding in low-permeability reservoirs. Plugging/unplugging can also be achieved via CO2/N2 bubbling.
Zhang et al. [81] synthesized a CO2-responsive WLMs by mixing three different types of surfactants that containing N-erucamidopropyl-N, N′-dimethylamine (UC22AMPM), sodium dodecyl sulfate (SDS) and N,N,N′,N′-tetramethyl-1,3-propanediamine (TMPDA). When CO2 is bubbled into an aqueous mixture, the tertiary amines in the TMPDA molecules are protonated. Through electrostatic attraction, “bridging” occurred between two SDS molecules, forming pseudo-gemini surfactants and creating a viscoelastic WLM. After CO2 removal, the quaternary ammonium salts acting as spacer groups were deprotonated into tertiary amines, and the vermicular micelles of the pseudo-gemini surfactants were dissociated into spheroidal micelles of conventional SDS molecules with low viscosity. Ten years later, Luo et al. [82] first reported to use of UC22AMPM to block CO2 gas channeling for WAG through in-situ gelation. When N2 is bubbled into the system, the system becomes neutral, and the tertiary amine group deprotonates, ultimately forming a low-viscosity emulsion. Compared to traditional WAG, the recovery rate is increased by 8.0%, proving effective in mitigating gas channeling.
Wang et al. [83] developed a switching foam using long-chain cationic surfactants N-sinapide-propyl-N and N-dimethylammonium bicarbonate (UC22AMPM·H+) with carbon dioxide/ammonia water as an irritant. It acted as both a foaming agent and foam stabilizer. Under CO2 conditions, the long-chain amine surfactant was protonated into cationic surfactant, promoting UC22AMPM·H+ self-assembly into WLM. This increased the bulk phase viscosity and gas/liquid surface viscosity, enhancing foam stability. Upon adding NH3·H2O, the WLM network structure was disrupted, and the bulk phase viscosity and surface viscosity subsequently decreased and reducing foam stability. In addition, CO2 can also act as a stimulus to protonate long-chain tertiary amine surfactants into cationic surfactants and form viscoelastic WLMs to stabilize the foam.
Gao et al. [84] prepared a novel CO2-responsive zymphoteric WLMs by mixing N-octadecyl-N,N-dimethyl-3ammonio-2-hydroxy-1-propane-sulfonate (OHSB),N,N,2,2-tetramethyl-1,3-propanediamine (TADPA) and sodium salicylate (NaSal). The OHSB/NaSal/TADPA system shows morphological transformations between spherical micelles (a good flowing capacity) and WLMs (conducive to plugging) before and after purging CO2. After CO2 is bubbled into an aqueous mixture of these reactants, which will occur the reaction of CO2-responsive mechanism one. On the one hand, it can react with metal ions on the rock surface to precipitate for storage. On the other hand, the viscosity of the system is significantly increased with the increase of the intermolecular electrostatic repulsion, and the system is self-assembled into a three-dimensional network structure, making it effective in inhibiting gas channeling and increasing recovery.
Zhao et al. [85] prepared the surfactant erucic amide propyl dimethylamine (EAPD) as raw materials at a molar ratio of 1:1.1. In the absence of CO2, EAPD molecules in solution exist mainly in the form of spherical micelles. When CO2 is bubbled, EAPD molecules undergo a protonation reaction and become cationic molecules. With the introduction of counter ions, self-assembled aggregates are formed based on the synergistic effect of hydrophobic association and static electricity. It transforms the spherical micelle into a WLMs. Due to the formation of a three-dimensional network structure, the viscosity of the system increased significantly after CO2 bubbling, and formed a gel with a certain strength. When encountering oil or N2, the EAPD molecule undergoes a deprotonation reaction, and the WLMs turn into a spherical micelle again. Through mobility control ability plugging by the double pipes flooding experiments, the gel system can increase the recovery rate of low permeability reservoir by 18.7%.
Liu et al. [86] developed a double cross-linked frustrated Lewis pair network (FLPN) through a specific three-component reaction among triaryl borane, triarylphosphine, and CO2. which is composed of permanent chemical crosslinks and dynamic CO2 gas-bridged connections. The addition of CO2 could adjust the density of supramolecular nodes in such FLPN, thus controlling the strength and toughness of the gel material. In addition, the self-healing of broken gels can be achieved by CO2 stimulus through the reconstruction of dynamic covalent networks.
Zheng et al. [87] prepared a CO2-switched, injectable, block copolymer hydrogel by the combination of coordination-driven self-assembly and stepwise post-assembly polymerization, star block copolymers (SBCPs) containing well-defined hexagonal metallacycles as cores. SBCP formed a stable single molecule micelle in an aqueous solution, in which the hydrophobic metal ring may be wrapped by the folded block copolymer chain. After CO2 simulation, CO2 and charged tertiary amines form water-soluble ammonium bicarbonate. The supramolecular amphiphilic polymer aggregates into large vesicles in aqueous solution through the hydrophilic/hydrophobic interaction.After N2 simulation. The protonation of CO2 with dimethylamino group is inferiored, the hydrophilicity is reduced, and the vesicles are transformed into single molecule micelles.
Huang et al. [88] prepared a switchable pseudo-hydrophobic associative polymer using PEI and SDS. Under the stimulation of CO2, the imide group of PEI is protonated, and produces electrostatic interaction and hydrophobic association with the SDS of the anion, thus forming a physical cross-linked network in the mixed aqueous solution, and the viscosity of the solution is significantly increased. However, as a result of the electrostatic interaction between negatively charged SDS and positively charged PEI, the hydrophilicity of PEI decreases, leading to the hydrophobic association physical crosslinked network contraction, and the solution precipitates white precipitates when PEI approaches neutral electricity. Continuous CO2 bubbling increased the protonation degree of the amine group on the PEI molecular chain, enhancing the hydrophilicity of PEI and dissolving the precipitate, resulting in a significant increase in the solution viscosity of the SDS/PEI.
Yan et al. [89] reported a completely biobased pickering emulsion gel stabilized from modified cellulose (CNF), which was modified with a CO2-responsive rosin-based surfactant, maleopimaric acid glycidyl methacrylate ester 3-dimethylaminopropylamine imide (MPAGN). As this surfactant has sensitive CO2-responsive properties, allowing for switchable emulsification/demulsification, MPAGN can reversibly respond to CO2 and N2 between active cationic (MPAGNH+) and inactive nonionic (MPAGN).
Ali et al. [90] prepared a pH-responsive gel system by using cellulose nanocrystals (CNC) and imidazole salts to form a suspension. After the CO2 is bubbled into a supension, the pH of the system decreases, the generated carbonic acid dissociates a large number of protons, and through electrostatic interaction with the sulfonic acid groups on the surface of the imidazole salt, the cross-linking density of the internal network of the CNC hydrogel was enhanced, and in-situ gelation was achieved. Upon removal of CO2, the system’s pH increased and the imidazole ions were deprotonated, the gel structure was destroyed and restored to the original sol, forming a tunable hydrogel by changing the concentration of imidazolium salt.
3.2.2 Macromolecule Self-Assembly
The CO2-responsive macromolecular self-assembly unit structure can be linear polymer or nonlinear polymer, or biological macromolecule, making the self-assembled of macromolecule more intricate than the self-assembled of micromolecule [77]. These macromolecules are typically divided into two categories: one with uncharged alkaline units (such as amine groups), which are hydrophilic at low pH and protonate into hydrophobic segments at high pH; and the other is macromolecules with acidic units (such as carboxyl groups), protonating into positively charged segments at low pH and carrying a negative charge at high pH. Common macromolecules with CO2 responsiveness include polyacrylic acid (PAA), polyvinylpyridine (PVPy), polymethyl methacrylate (PMAA), PDMAEMA, PDEA, etc. [77]. As shown in Fig. 9.
Figure 9: The common pH-responsive polymers (modified from Jiang et al. [77])
Luo et al. [91] synthesized a CO2-responsive triblock copolymer by one-step dispersion/emulsion polymerization. DMAEMA was used as a CO2-responsive monomer, 2,2’-azobis (2, 4-dimethylglutaronitrile) (AVBN) and lauroyl peroxide (LPO) as initiator. By grafting PDMAEMA onto the triblock copolymer Pluronic F127, PEO100-PPO65-PEO100 for use to increase the viscosity of water in the WAG process. After CO2 simulation, the viscosity of the aqueous phase increases significantly. The mechanism can be explained as follows: PDMAEMA is a weak base (pKa~7.4). Upon bubbling with CO2, the generated carbonic acid dissociated into a large number of protons, leading to the protonation of the tertiary amine groups in PDMAEMA. This caused the side chains to extend due to electrostatic repulsion, resulting in the polymer having a larger hydrodynamic diameter, and increased entanglement of the polymer chains, thereby increasing solution viscosity. Upon N2 simulation, the tertiary amine groups underwent deprotonation, restoring the solution to its initial state, and achieving CO2/N2 reversibility. Core flooding experiments demonstrated a 21.7% increase in conventional WAG oil recovery, effectively sealing CO2 migration.
Younis et al. [92] synthesized carbon dioxide (H+/CO2)-sensitive poly (ethylene glycol)-b-poly (2-(diisopropylamino) ethyl methacrylate)-b-polystyrene triblock polymer (PEG-b-PDPA-b-PS) for programmed release of chemotherapeutic drugs against glioblastoma tumor. The release mechanism is as follows: the vesicle structure of multifunctional gold vesicles (GVND) first expands in the acidic microenvironment of the tumor, and then partially breaks through GVND under near-infrared (NIR) laser irradiation. Subsequently, the mild and high temperature generated by GV triggers the decomposition of NH4HCO3 to produce CO2. The in-situ CO2 reacts with the tertiary amine group of PDPA, enhancing the hydrophilicity of the vesicles, disrupting its vesicle structure, and ultimately leading to the “bomb-like” release of doxorubicin (DOX) in the tumor tissues. This multi-stimulus-responsive programmed drug delivery and mild hyperthermia under near-infrared laser activation demonstrate potent antitumor efficacy and completely eradicated U87MG glioblastoma tumor.
4 Conclusion and Recommendations
Currently, the most relevant technologies involve measures and plugging agents for mitigating water flooding in heterogeneous reservoirs. It is evident that while CO2 gas channeling and water channeling share similarities, there are significant differences as well. Therefore, the application of plugging agents used for water flooding control in CO2 flooding presents considerable adaptability challenges and technical risks.
This paper, starting from the chemical response mechanism of CO2 and the mechanism of gel channeling, thoroughly investigates the molecular structures, chemical compositions, response mechanisms, and response characteristics of CO2-responsive gels in various application fields, including double crosslink gel, metallic ions crosslink gel, organic crosslink gel, micromolecule self-assembly, and macromolecule self-assembly, aiming to apply them in CO2-EOR field. However, there are still the following deficiencies in the application of CO2-responsive gels in the development of oil and gas field:
1. The current application conditions only involve the gel performance under room temperature and pressure, which is greatly different from the use environment of CO2 plugging channeling agents, and also limits the synthesis and application of CO2 response plugging system to a certain extent, with certain limitations;
2. There are few studies on the influence of chemical response characteristics and reaction rate of CO2 under different reservoir conditions;
3. There are few relevant studies on the effect of temperature and pressure on the phase change of supercritical CO2 under reservoir conditions, and the effect of application technology on the formations and properties of gels;
4. The existing plugging agents have a high cost and are difficult to be widely used.
Therefore, developing low-cost and high-tolerance CO2-responsive gels is a crucial topic for solving various problems in current CO2-EOR technology. In-depth research on the relationship between structure and performance under reservoir conditions and the plugging mechanisms will be a major direction for future development.
Acknowledgement: None.
Funding Statement: Supported by the Open Fund Project of Hubei Key Laboratory of Oil and Gas Drilling and Production Engineering (YQZC202105) and Yangtze University Student Innovation Program (Yz2022018).
Author Contributions: Study conception and design: Yang Xiong, Jianxin Liu; data collection: Xianhao Yi, Bangyan Xiao, Dan Wu, Biao Wu, Chunyu Gao. All authors reviewed the results and approved the final version of the manuscript.
Availability of Data and Materials: The authors ensure the authenticity and validity of the materials and data in the article.
Conflicts of Interest: The authors declare that they have no conflicts of interest to report regarding the present study.
References
1. Yuan, S. Y., Ma, D. S., Li, J. S., Zhou, T. Y., Ji, Z. M. et al. (2022). Progress and prospects of carbon dioxide capture, EOR-utilization and storage industrialization. Petroleum Exploration and Development, 49(4), 955–962. https://doi.org/10.1016/S1876-3804(22)60324-0 [Google Scholar] [CrossRef]
2. Kang, W. L., Zhou, B. B., Issakhov, M., Gabdullin, M. (2022). Advances in enhanced oil recovery technologies for low permeability reservoirs. Petroleum Science, 19(4), 1622–1640. https://doi.org/10.1016/j.petsci.2022.06.010 [Google Scholar] [CrossRef]
3. Qi, L., Yajie, L. (2023). A review of channeling blocking gel systems for CO2 flooding. Petroleum Geology and Recovery Efficiency, 30(2), 122–134 (In Chinese). https://doi.org/10.13673/j.cnki.cn37-1359/te.202106026 [Google Scholar] [CrossRef]
4. Wang, L., Tian, Y., Yu, X. Y., Wang, C., Yao, B. W. et al. (2017). Advances in improved/enhanced oil recovery technologies for tight and shale reservoirs. Fuel, 210, 425–445. https://doi.org/10.1016/j.fuel.2017.08.095 [Google Scholar] [CrossRef]
5. Dai, Z. X., Middleton, R., Viswanathan, H., Fessenden-Rahn, J., Bauman, J. et al. (2014). An integrated framework for optimizing CO2 sequestration and enhanced oil recovery. Environmental Science & Technology Letters, 1(1), 49–54. https://doi.org/10.1021/ez4001033 [Google Scholar] [CrossRef]
6. Han, L., Gu, Y. (2014). Optimization of miscible CO2 water-alternating-gas injection in the bakken formation. Energy & Fuels, 28(11), 6811–6819. https://doi.org/10.1021/ef501547x [Google Scholar] [CrossRef]
7. Panjalizadeh, H., Alizadeh, A., Ghazanfari, M., Alizadeh, N. (2015). Optimization of the WAG injection process. Petroleum Science and Technology, 33(3), 294–301. https://doi.org/10.1080/10916466.2014.956897 [Google Scholar] [CrossRef]
8. Carpenter, C. (2019). Experimental program investigates miscible CO2 WAG injection in carbonate reservoirs. Journal of Petroleum Technology, 71(1), 47–49. https://doi.org/10.2118/0119-0047-jpt [Google Scholar] [CrossRef]
9. Hsie, J. C., Stephen Moore, J. (1988). The quarantine bay 4RC CO2 WAG pilot projects: A postflood evaluation. SPE Reservoir Engineering, 3(3), 809–814. https://doi.org/10.2118/15498-pa [Google Scholar] [CrossRef]
10. Kumar, S., Mandal, A. (2017). A comprehensive review on chemically enhanced water alternating gas/CO2 (CEWAG) injection for enhanced oil recovery. Journal of Petroleum Science and Engineering, 157, 696–715. https://doi.org/10.1016/j.petrol.2017.07.066 [Google Scholar] [CrossRef]
11. Brokmeyer, R. J., Borling, D. C., Pierson, W. T. (1996). Lost soldier tensleep CO2 tertiary project, performance case history; Bairoil, Wyomingt. SPE Permian Basin Oil and Gas Recovery Conference, SPE-35191-MS. Midland, USA. https://doi.org/10.2118/35191-MS [Google Scholar] [CrossRef]
12. Wang, Q., Yang, S. L., Lorinczi, P., Glover, P., Lei, H. (2019). Experimental investigation of oil recovery performance and permeability damage in multilayer reservoirs after CO2 and water-alternating-CO2 (CO2–WAG) flooding at miscible pressures. Energy & Fuels, 34(1), 624–636. https://doi.org/10.1021/acs.energyfuels.9b02786 [Google Scholar] [CrossRef]
13. Zhou, X., Yuan, Q., Rui, Z., Wang, H., Feng, J. et al. (2019). Feasibility study of CO2 huff ‘n’ puff process to enhance heavy oil recovery via long core experiments. Applied Energy, 236, 526–539. https://doi.org/10.1016/j.apenergy.2018.12.007 [Google Scholar] [CrossRef]
14. Han, J., Lee, M., Lee, W., Lee, Y., Sung, W. (2016). Effect of gravity segregation on CO2 sequestration and oil production during CO2 flooding. Applied Energy, 161, 85–91. https://doi.org/10.1016/j.apenergy.2015.10.021 [Google Scholar] [CrossRef]
15. He, C. Z., Hua, M. Q. (1996). Study on water block effect. Drilling Fluid & Completion Fluid, 6, 14–16 (In Chinese). [Google Scholar]
16. Massarweh, O., Abushaikha, A. S. (2022). A review of recent developments in CO2 mobility control in enhanced oil recovery. Petroleum, 8(3), 291–317. https://doi.org/10.1016/j.petlm.2021.05.002 [Google Scholar] [CrossRef]
17. Pal, N., Zhang, X., Ali, M., Mandal, A., Hoteit, H. (2022). Carbon dioxide thickening: A review of technological aspects, advances and challenges for oilfield application. Fuel, 315, 122947. https://doi.org/10.1016/j.fuel.2021.122947 [Google Scholar] [CrossRef]
18. Enick, R., Eric, J., Chunmei, S., Zhihua, H., Sevgi, K. (2000). Direct thickeners for carbon dioxide. Proceedings of the SPE/DOE Improved Oil Recovery Symposium, SPE-59325-MS. Tulsa, USA. https://doi.org/10.2118/59325-MS [Google Scholar] [CrossRef]
19. Liu, B., Wang, Y., Liang, L. (2020). Current status and prospects of supercritical carbon dioxide thickeners. Applied Chemical Industry, 49(12), 3120–3125 (In Chinese). https://doi.org/10.16581/j.cnki.issn1671-3206.20201021.007 [Google Scholar] [CrossRef]
20. Lemaire, P. C., Alenzi, A., Lee, J. J., Beckman, E. J., Enick, R. M. (2021). Thickening CO2 with direct thickeners, CO2-in-oil emulsions, or nanoparticle dispersions. Literature Review and Experimental Validation. Energy & Fuels, 35(10), 8510–8540. https://doi.org/10.1021/acs.energyfuels.1c00314 [Google Scholar] [CrossRef]
21. Xiong, Y., Kiran, E. (1995). Miscibility, density and viscosity of poly (dimethylsiloxane) in supercritical carbon dioxide. Polymer, 36(25), 4817–4826. https://doi.org/10.1016/0032-3861(95)99298-9 [Google Scholar] [CrossRef]
22. Li, Q., Wang, Y., Li, Q., Foster, G., Lei, C. (2018). Study on the optimization of silicone copolymer synthesis and the evaluation of its thickening performance. RSC Advances, 8(16), 8770–8778. https://doi.org/10.1039/C7RA13645E [Google Scholar] [PubMed] [CrossRef]
23. Dai, C., Liu, P., Gao, M., Liu, Z., Liu, C. et al. (2022). Preparation and thickening mechanism of copolymer fluorinated thickeners in supercritical CO2. Journal of Molecular Liquids, 360, 119563. https://doi.org/10.1016/j.molliq.2022.119563 [Google Scholar] [CrossRef]
24. Zaberi, H. A., Lee, J. J., Enick, R. M., Beckman, E. J., Cummings, S. D. et al. (2020). An experimental feasibility study on the use of CO2-soluble polyfluoroacrylates for CO2 mobility and conformance control applications. Journal of Petroleum Science and Engineering, 184, 106556. https://doi.org/10.1016/j.petrol.2019.106556 [Google Scholar] [CrossRef]
25. Zhang, M., Zhao, F. L., Hou, J. R., Feng, R. H., Zhang, D. M. (2020). Effect of thickener and carbon dioxide system on mobility reduction and gas flooding improvement. Oilfield Chemisty, 37(2), 273–278 (In Chinese). https://doi.org/10.19346/j.cnki.1000-4092.2020.02.016 [Google Scholar] [CrossRef]
26. Shah, P., Cole, M., Beckman, E., Enick, R. (2024). Advances in thickeners of CO2: Application potential of poly-1-decene. Gas Science and Engineering, 121, 205168. https://doi.org/10.1016/j.jgsce.2023.205168 [Google Scholar] [CrossRef]
27. Lei, S., Sun, J., Lv, K., Zhang, Q., Yang, J. (2022). Types and performances of polymer gels for oil-gas drilling and production: A review. Gels, 8(6), 386. https://doi.org/10.3390/gels8060386 [Google Scholar] [PubMed] [CrossRef]
28. Bai, B., Li, L., Liu, Y., Liu, H., Wang, Z. et al. (2007). Preformed particle gel for conformance control: Factors affecting its properties and applications. SPE Reservoir Evaluation & Engineering, 10(4), 415–422. https://doi.org/10.2118/89389-pa [Google Scholar] [CrossRef]
29. Lantz, M., Muniz, G. (2014). Conformance improvement using polymer gels: A case study approach. Proceedings of the SPE Improved Oil Recovery Symposium, SPE-169072-MS. Tulsa, USA. https://doi.org/10.2118/169072-MS [Google Scholar] [CrossRef]
30. Zhou, B., Kang, W., Yang, H., Zhu, T., Zhang, H. et al. (2021). Preparation and properties of an acid-resistant preformed particle gel for conformance control. Journal of Petroleum Science and Engineering, 197, 107964. https://doi.org/10.1016/j.petrol.2020.107964 [Google Scholar] [CrossRef]
31. Kwok, A. Y., Qiao, G. G., Solomon, D. H. (2003). Synthetic hydrogels. 1. Effects of solvent on poly(acrylamide) networks. Polymer, 44(20), 6195–6203. https://doi.org/10.1016/S0032-3861(03)00671-2 [Google Scholar] [CrossRef]
32. Schuman, T., Salunkhe, B., Al Brahim, A., Bai, B. (2022). Evaluation of ultrahigh-temperature-resistant preformed particle gels for conformance control in north sea reservoirs. SPE Journal, 27(6), 3660–3673. https://doi.org/10.2118/206007-pa [Google Scholar] [CrossRef]
33. Yan, W., Miller, C. A., Hirasaki, G. J. (2006). Foam sweep in fractures for enhanced oil recovery. Colloids and Surfaces A: Physicochemical and Engineering Aspects, 282–283, 348–359. https://doi.org/10.1016/j.colsurfa.2006.02.067 [Google Scholar] [CrossRef]
34. Mansha, M., Ali, S., Alsakkaf, M., Karadkar, P. B., Harbi, B. G. et al. (2023). Advancements in nanoparticle-based stabilization of CO2 foam: Current trends, challenges, and future prospects. Journal of Molecular Liquids, 391, 123364. https://doi.org/10.1016/j.molliq.2023.123364 [Google Scholar] [CrossRef]
35. Holm, L. W. (1968). The mechanism of gas and liquid flow through porous media in the presence of foam. Society of Petroleum Engineers Journal, 8(4), 359–369. https://doi.org/10.2118/1848-pa [Google Scholar] [CrossRef]
36. Sagir, M., Tan, I. M., Mushtaq, M., Pervaiz, M., Tahir, M. S. et al. (2016). CO2 mobility control using CO2 philic surfactant for enhanced oil recovery. Journal of Petroleum Exploration and Production Technology, 6(3), 401–407. https://doi.org/10.1007/s13202-015-0192-8 [Google Scholar] [CrossRef]
37. Ren, G., Nguyen, Q. P., Lau, H. C. (2018). Laboratory investigation of oil recovery by CO2 foam in a fractured carbonate reservoir using CO2-soluble surfactants. Journal of Petroleum Science and Engineering, 169, 277–296. https://doi.org/10.1016/j.petrol.2018.04.053 [Google Scholar] [CrossRef]
38. Dehaghani, A. H. S., Gharibshahi, R., Mohammadi, M. (2023). Utilization of synthesized silane-based silica Janus nanoparticles to improve foam stability applicable in oil production: Static study. Scientific Reports, 13(1), 18652. https://doi.org/10.1038/s41598-023-46030-1 [Google Scholar] [PubMed] [CrossRef]
39. Mheibesh, Y., Hethnawi, A., Sessarego, S., Manasrah, A. D., Nassar, N. N. (2023). Stabilization of carbon dioxide foam by a low concentration of cetyltrimethylammonium bromide-grafted nano-faujasite zeolite. Part 1: Experimental study. Energy & Fuels, 37(22), 17187–17202. https://doi.org/10.1021/acs.energyfuels.3c02188 [Google Scholar] [CrossRef]
40. Thakore, V., Wang, H., Wang, J. A., Polsky, Y., Ren, F. (2024). Stability study of aqueous foams under high-temperature and high-pressure conditions relevant to enhanced geothermal systems (EGS). Geothermics, 116, 102862. https://doi.org/10.1016/j.geothermics.2023.102862 [Google Scholar] [CrossRef]
41. Alcorn, Z. P., Føyen, T., Zhang, L., Karakas, M., Biswal, S. L. et al. (2020). CO2 foam field pilot design and initial results. Proceedings of the SPE Improved Oil Recovery Conference, SPE-200450-MS. https://doi.org/10.2118/200450-MS [Google Scholar] [CrossRef]
42. Mukherjee, J., Norris, S. O., Nguyen, Q. P., Scherlin, J. M., Vanderwal, P. G. et al. (2014). CO2 foam pilot in salt creek field, Natrona County, WY: Phase I: Laboratory work, reservoir simulation, and initial design. Proceedings of the SPE Improved Oil Recovery Symposium, SPE-169166-MS. Amsterdam, The Netherlands. https://doi.org/10.2118/170729-MS [Google Scholar] [CrossRef]
43. Kang, W., Jiang, H., Yang, H., Li, Z., Zhou, B. et al. (2021). Study of nano-SiO2 reinforced CO2 foam for anti-gas channeling with a high temperature and high salinity reservoir. Journal of Industrial and Engineering Chemistry, 97, 506–514. https://doi.org/10.1016/j.jiec.2021.03.007 [Google Scholar] [CrossRef]
44. Lin, S. J., Theato, P. (2013). CO2-responsive polymers. Macromolecular Rapid Communications, 34(14), 1118–1133. https://doi.org/10.1002/marc.201300288 [Google Scholar] [PubMed] [CrossRef]
45. Pu, J. Y., Bai, B. J., Geng, J. M., Zhang, N., Thomas, S. (2023). Dual crosslinked poly(acrylamide-co-N-vinylpyrrolidone) microspheres with re-crosslinking ability for fossil energy recovery. Geoenergy Science and Engineering, 224, 211604. https://doi.org/10.1016/j.geoen.2023.211604 [Google Scholar] [CrossRef]
46. Song, B., Qin, A., Tang, B. Z. (2022). Syntheses, properties, and applications of CO2-based functional polymers. Cell Reports Physical Science, 3(2), 100719. https://doi.org/10.1016/j.xcrp.2021.100719 [Google Scholar] [CrossRef]
47. Zhou, Y. H., Luo, Z., Xu, M., Zhao, T. Y., Ma, X. et al. (2023). Preparation and properties of temperature-sensitive P(NIPAM-AM) nano-microspheres in enhanced oil recovery. Energy & Fuels, 37(1), 204–213. https://doi.org/10.1021/acs.energyfuels.2c02972 [Google Scholar] [CrossRef]
48. Pu, J. Y., Bai, B. J., Geng, J. M., Zhang, N., Schuman, T. (2023). Dual crosslinked poly(acrylamide-co-N-vinylpyrrolidone) microspheres with re-crosslinking ability for fossil energy recovery. Geoenergy Science and Engineering, 224, 211604. https://doi.org/10.1016/j.geoen.2023.211604 [Google Scholar] [CrossRef]
49. Zhao, J. B., Zhou, Z. J., Wang, Y. X., Guo, Y. X., Cui, X. (2017). Research on in-depth profile control gel system for moderate-high temperature and low-permeability reservoir. Chemical Engineering Journal, 31(8), 1–3. https://doi.org/10.16247/j.cnki.23-1171/tq.20170801 [Google Scholar] [CrossRef]
50. Dovan, H. T., Hutchins, R. D., Sandiford, B. B. (1997). Delaying gelation of aqueous polymers at elevated temperatures using novel organic crosslinkers. Proceedings of the International Symposium on Oilfield Chemistry, SPE-37246-MS. Houston, USA. https://doi.org/10.2118/37246-MS [Google Scholar] [CrossRef]
51. Raje, M., Asghari, K., Vossoughi, S., Green, D. W., Willhite, G. P. (1999). Gel systems for controlling CO2 mobility in carbon dioxide miscible flooding. SPE Reservoir Evaluation & Engineering, 2(2), 205–210. https://doi.org/10.2118/55965-PA [Google Scholar] [CrossRef]
52. Massarweh, O., Abushaikha, A. S. (2023). The synergistic effects of cationic surfactant and smart seawater on the recovery of medium-viscosity crude oil from low-permeability carbonates. Journal of Molecular Liquids, 389, 122866. https://doi.org/10.1016/j.molliq.2023.122866 [Google Scholar] [CrossRef]
53. Pu, W. F., Du, D. J., Fan, H. C., Chen, B. W., Yuan, C. D. et al. (2021). CO2-responsive preformed gel particles with interpenetrating networks for controlling CO2 breakthrough in tight reservoirs. Colloids and Surfaces A: Physicochemical and Engineering Aspects, 613, 126065. https://doi.org/10.1016/j.colsurfa.2020.126065 [Google Scholar] [CrossRef]
54. Sun, X., Alhuraishawy, A. K., Bai, B., Wei, M. (2018). Combining preformed particle gel and low salinity waterflooding to improve conformance control in fractured reservoirs. Fuel, 221, 501–512. https://doi.org/10.1016/j.fuel.2018.02.084 [Google Scholar] [CrossRef]
55. Zhang, H. J., Zhang, Y. N., Yang, B. A., Wang, L. G. (2018). Swelling or deswelling? Composition-dependent CO2-responsive swelling behaviors of poly(acrylamide-co-2-dimethylaminoethyl methacrylate) hydrogels. Colloid and Polymer Science, 296(2), 393–403. https://doi.org/10.1007/s00396-018-4262-6 [Google Scholar] [CrossRef]
56. Zhang, Y. S., Mao, X., Liu, T., Guo, Z. R., Xiong, L. Y. et al. (2022). Reversible adsorption/release for anionic dyes of magnetic and CO2 dual-responsive degradable microgels. Journal of Functional Polymers, 35(2), 164–171 (In Chinese). https://doi.org/10.14133/j.cnki.1008-9357.20210322003 [Google Scholar] [CrossRef]
57. Chen, S., Lin, X., Zhai, Z., Lan, R., Li, J. et al. (2018). Synthesis and characterization of CO2-sensitive temperature-responsive catalytic poly(ionic liquid) microgels. Polymer Chemistry, 9(21), 2887–2896. https://doi.org/10.1039/c8py00352a [Google Scholar] [CrossRef]
58. Corona Rivera, M. A., Cisneros Covarrubias, C. A., Fernández Escamilla, V. V. A., Mendizábal Mijares, E., Pérez López, J. E. (2022). Synthesis and characterization of pH-responsive water-dispersed nanohydrogels of cross-linked polyacrylamide-co-polyacrylic acid. Polymer Engineering & Science, 62(6), 1797–1810. https://doi.org/10.1002/pen.25965 [Google Scholar] [CrossRef]
59. He, Z. F., Chen, Q., Luo, Y. Y., He, Y., Zhang, Y. S. et al. (2021). Degradable CO2-responsive microgels with wrinkled porous structure for enhanced, selective and recyclable removal of anionic dyes, Cr(VI) and As(V). European Polymer Journal, 149, 110374. https://doi.org/10.1016/j.eurpolymj.2021.110374 [Google Scholar] [CrossRef]
60. Jansen-van Vuuren, R. D., Naficy, S., Ramezani, M., Cunningham, M., Jessop, P. (2023). CO2-responsive gels. Chemical Society Reviews, 52(10), 3470–3542. https://doi.org/10.1039/d2cs00053a [Google Scholar] [PubMed] [CrossRef]
61. Rabiee, H., Jin, B., Yun, S., Dai, S. (2018). Gas-responsive cationic microgels for forward osmosis desalination. Chemical Engineering Journal, 347, 424–431. https://doi.org/10.1016/j.cej.2018.04.148 [Google Scholar] [CrossRef]
62. Xu, L. F., Wang, Z. F., Dong, X. Y., Yang, G. W., Zhang, G. B. et al. (2021). Preparation and properties of efficient emulsification/demulsification CO2-triggered waterborne polyurethane. Polymer Matierals Science and Energing, 37(7), 8–13 (In Chinese). https://doi.org/10.16865/j.cnki.1000-7555.2021.0185 [Google Scholar] [CrossRef]
63. Shieh, Y. T., Yeh, Y. C., Cheng, C. C. (2020). Two-way CO2-responsive polymer particles with controllable amphiphilic properties. ACS Omega, 5(4), 1862–1869. https://doi.org/10.1021/acsomega.9b03319 [Google Scholar] [PubMed] [CrossRef]
64. Al Akoumy, C., Augé, A., Ma, D. L., Zhao, Y. (2022). Yolk-shell nanoparticles with CO2-responsive outer shells for gas-controlled catalysis. ACS Applied Nano Materials, 5(12), 18237–18246. https://doi.org/10.1021/acsanm.2c04121 [Google Scholar] [CrossRef]
65. Mu, M., Yin, H., Feng, Y. (2017). CO2-responsive polyacrylamide microspheres with interpenetrating networks. Journal of Colloid and Interface Science, 497, 249–257. https://doi.org/10.1016/j.jcis.2017.03.012 [Google Scholar] [PubMed] [CrossRef]
66. Qi, Z. B., Liu, Y. F., Gao, Q., Tao, D. W., Wang, Y. et al. (2023). CO2-responsive nanofibrous membranes with gas-tunable wettability for switchable oil/water separation. Reactive and Functional Polymers, 182, 105481. https://doi.org/10.1016/j.reactfunctpolym.2022.105481 [Google Scholar] [CrossRef]
67. Sun, X., Bai, B., Long, Y. F., Wang, Z. (2020). A comprehensive review of hydrogel performance under CO2 conditions for conformance control. Journal of Petroleum Science and Engineering, 185. https://doi.org/10.1016/j.petrol.2019.106662 [Google Scholar] [CrossRef]
68. Mo, A. G., Wang, Y. P., Liu, Y. K., Zhou, W. F., Zhou, Q. et al. (2018). Enhanced viscosity of poly(acrylamide) solution in the presence of chromium citrate triggered by release of CO2. Chinese Journal of Chemical Physics, 31(1), 117–122. https://doi.org/10.1063/1674-0068/31/cjcp1706128 [Google Scholar] [CrossRef]
69. Hadi Mosleh, M., Govindan, R., Shi, J. Q., Durucan, S., Korre, A. (2016). Application of polymer-gel solutions in remediating leakage in CO2 storage reservoirs. Proceedings of the SPE Europec Featured at 78th EAGE Conference and Exhibition, SPE-180135-MS. Vienna, Austria. https://doi.org/10.2118/180135-MS [Google Scholar] [CrossRef]
70. Li, D. X., Zhang, L., Liu, Y. M., Kang, W. L., Ren, S. R. (2016). CO2-triggered gelation for mobility control and channeling blocking during CO2 flooding processes. Petroleum Science, 13(2), 247–258. https://doi.org/10.1007/s12182-016-0090-9 [Google Scholar] [CrossRef]
71. Wu, Y., Liu, Q., Liu, D., Cao, X. P., Yuan, B. et al. (2023). CO2 responsive expansion hydrogels with programmable swelling for in-depth CO2 conformance control in porous media. Fuel, 332, 126047, https://doi.org/10.1016/j.fuel.2022.126047 [Google Scholar] [CrossRef]
72. Skilling, K. J., Citossi, F., Bradshaw, T. D., Ashford, M., Kellam, B. et al. (2014). Insights into low molecular mass organic gelators: A focus on drug delivery and tissue engineering applications. Soft Matter, 10(2), 237–256. https://doi.org/10.1039/c3sm52244j [Google Scholar] [PubMed] [CrossRef]
73. Lu, W., Le, X. X., Zhang, J. W., Huang, Y. J., Chen, T. (2017). Supramolecular shape memory hydrogels: A new bridge between stimuli-responsive polymers and supramolecular chemistry. Chemical Society Reviews, 46(5), 1284–1294. https://doi.org/10.1039/c6cs00754f [Google Scholar] [PubMed] [CrossRef]
74. Xia, D., Wang, P., Ji, X., Khashab, N. M., Sessler, J. L. et al. (2020). Functional supramolecular polymeric networks: The marriage of covalent polymers and macrocycle-based host-guest interactions. Chemical Reviews, 120(13), 6070–6123. https://doi.org/10.1021/acs.chemrev.9b00839 [Google Scholar] [PubMed] [CrossRef]
75. Jian, Y. K., Lu, W., Zhang, J. W., Chen, T. (2018). Research progress in supramolecular shape memory hydrogels. Journal of Functional Polymers, 11, 1385–1399 (In Chinese). https://doi.org/10.11777/j.issn1000-3304.2018.18150 [Google Scholar] [CrossRef]
76. Dong, R., Zhou, Y. F., Huang, X. H., Zhu, X. Y., Lu, Y. et al. (2015). Functional supramolecular polymers for biomedical applications. Advanced Materials, 27(3), 498–526. https://doi.org/10.1002/adma.201402975 [Google Scholar] [PubMed] [CrossRef]
77. Jiang, C. Q., Xu, G. H., Gao, J. P. (2022). Stimuli-responsive macromolecular self-assembly. Sustainability, 14(18), 11738. https://doi.org/10.3390/su141811738 [Google Scholar] [CrossRef]
78. Han, Y. G., Wang, Y. F., Li, Y., Ma, Z. H. (2019). Research progress on properties and oilfield application of worm-like micelles. Applied Chemical Industry, 48(S1), 30–36 (In Chinese). https://doi.org/10.16581/j.cnki.issn1671-3206.2019.s1.005 [Google Scholar] [CrossRef]
79. Dai, M. L., Wei, F. L., Lu, Y. J., Xiong, C. M., Shao, L. M. et al. (2020). A new type of CO2 responsive gel based channeling blocking system. Oil Drilling & Production Technology, 42(6), 772–778 (In Chinese). https://doi.org/10.13639/j.odpt.2020.06.018 [Google Scholar] [CrossRef]
80. Shen, H., Yang, Z. H., Li, X. C., Peng, Y., Lin, M. Q. et al. (2021). CO2-responsive agent for restraining gas channeling during CO2 flooding in low permeability reservoir. Fuel, 292. https://doi.org/10.1016/j.fuel.2021.120306 [Google Scholar] [CrossRef]
81. Zhang, Y., Feng, Y., Wang, Y., Li, X. (2013). CO2-switchable viscoelastic fluids based on a pseudogemini surfactant. Langmuir, 29(13), 4187–4192. https://doi.org/10.1021/la400051a [Google Scholar] [PubMed] [CrossRef]
82. Luo, X. J., Wei, B., Gao, K., Jing, B., Huang, B. et al. (2023). Gas channeling control with an in-situ smart surfactant gel during water-alternating-CO2 enhanced oil recovery. Petroleum Science, 20(5), 2835–2851. https://doi.org/10.1016/j.petsci.2023.03.003 [Google Scholar] [CrossRef]
83. Wang, J., Liang, M. Q., Tian, Q. R., Feng, Y. J., Yin, H. Y. et al. (2018). CO2-switchable foams stabilized by a long-chain viscoelastic surfactant. Journal of Colloid and Interface Science, 523, 65–74. https://doi.org/10.1016/j.jcis.2018.03.090 [Google Scholar] [PubMed] [CrossRef]
84. Gao, M. W., Zhang, M. S., Liu, P., Dai, C. L., You, Q. et al. (2023). From nonionic to zwitterionic: Novel CO2-responsive zwitterionic wormlike micelles based on zwitterionic surfactant and bola-type asymmetric diamine. Fuel, 344, 127951. https://doi.org/10.1016/j.fuel.2023.127951 [Google Scholar] [CrossRef]
85. Zhao, M. W., Yan, X. W., Wang, X. Y., Yan, R. Q., Dai, C. L. (2023). The development of a smart gel for CO2 mobility control in heterogeneity reservoir. Fuel, 342, 127844. https://doi.org/10.1016/j.fuel.2023.127844 [Google Scholar] [CrossRef]
86. Liu, R. J., Wang, Y. X., Yan, Q. (2021). CO2-strengthened double-cross-linked polymer gels from frustrated lewis pair networks. Macromolecular Rapid Communications, 42(6). https://doi.org/10.1002/marc.202000699 [Google Scholar] [PubMed] [CrossRef]
87. Zheng, W., Yang, G., Shao, N. N., Chen, L. J., Ou, B. et al. (2017). CO2 stimuli-responsive, injectable block copolymer hydrogels cross-linked by discrete organoplatinum(II) metallacycles via stepwise post-assembly polymerization. Journal of the American Chemical Society, 139(39), 13811–13820. https://doi.org/10.1021/jacs.7b07303 [Google Scholar] [PubMed] [CrossRef]
88. Huang, X., Su, X. (2023). Pseudo hydrophobically associative polymer with CO2-switchable viscosity. Journal of Molecular Liquids, 372, 121170. https://doi.org/10.1016/j.molliq.2022.121170 [Google Scholar] [CrossRef]
89. Yan, X. Y., Wang, D. C., Wang, J., Huang, X. J., Cai, Z. S. (2023). CO2 responsive self-standing Pickering emulsion gel stabilized with rosin-based surfactant modified cellulose nanofibrils. International Journal of Biological Macromolecules, 246, 125717. https://doi.org/10.1016/j.ijbiomac.2023.125717 [Google Scholar] [PubMed] [CrossRef]
90. Ali, T., Christopher, J., Steven, B. (2020). Cellulose nanocrystal switchable gel for improving CO2 sweep efficiency in enhanced oil recovery and gas storage. Proceedings of the SPE Annual Technical Conference and Exhibition, SPE-201609-MS. https://doi.org/10.2118/201609-MS [Google Scholar] [CrossRef]
91. Luo, X. J., Zheng, P. F., Gao, K., Wei, B., Feng, Y. J. (2021). Thermo- and CO2-triggered viscosifying of aqueous copolymer solutions for gas channeling control during water-alternating-CO2 flooding. Fuel, 291, 120171. https://doi.org/10.1016/j.fuel.2021.120171 [Google Scholar] [CrossRef]
92. Younis, M. R., He, Y. L., Yao, X. K., He, G., Liu, H. K. et al. (2023). Acidity/carbon dioxide-sensitive triblock polymer-grafted photoactivated vesicles for programmed release of chemotherapeutic drugs against glioblastoma. Acta Biomaterialia, 157, 442–450. https://doi.org/10.1016/j.actbio.2022.11.053 [Google Scholar] [PubMed] [CrossRef]
Cite This Article
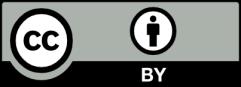
This work is licensed under a Creative Commons Attribution 4.0 International License , which permits unrestricted use, distribution, and reproduction in any medium, provided the original work is properly cited.