Open Access
REVIEW
Market Operation of Energy Storage System in Smart Grid: A Review
1 North China Dispatching Center, North China Branch of State Grid Corporation of China, Beijing, 100053, China
2 College of Electrical Engineering, Sichuan University, Chengdu, 610065, China
* Corresponding Author: Yuan Huang. Email:
Energy Engineering 2024, 121(6), 1403-1437. https://doi.org/10.32604/ee.2024.046393
Received 28 September 2023; Accepted 05 January 2024; Issue published 21 May 2024
Abstract
As a flexible resource, energy storage plays an increasingly significant role in stabilizing and supporting the power system, while providing auxiliary services. Still, the current high demand for energy storage contrasts with the fuzzy lack of market-oriented mechanisms for energy storage, the principle of market-oriented operation has not been embodied, and there is no unified and systematic analytical framework for the business model. However, the dispatch management model of energy storage in actual power system operation is not clear. Still, the specific scheduling process and energy storage strategy on the source-load-network side could be more specific, and there needs to be a greater understanding of the collaborative scheduling process of the multilevel scheduling center. On this basis, this paper reviews the energy storage operation model and market-based incentive mechanism, For different functional types and installation locations of energy storage within the power system, the operational models and existing policies for energy storage participation in the market that are adapted to multiple operating states are summarized. From the point of view of the actual scheduling and operation management of energy storage in China, an energy storage regulation and operation management model based on “national, provincial, and local” multilevel coordination is proposed, as well as key technologies in the interactive scenarios of source-load, network and storage.Keywords
With the rapid development of intermittent power sources such as wind power and photovoltaic power generation, the stabilizing and supporting role of energy storage technology in the power system is becoming increasingly significant [1–3]. In addition, energy storage systems (ESS) can provide auxiliary services for power systems, such as load tracking [4], spinning reserve [5], voltage adjustment [6], and black start capability [7]; and ESS can balance the instability associated with intermittent energy sources, thereby strongly contributing to the ability of renewable energy sources to connect to the grid. Energy storage is an important option to efficiently enhance the power system’s flexibility and smooth the source load’s random fluctuations. With the optimization of the market environment, independent energy storage gradually eliminates the constraints of the access location. It provides multiple services, not only to meet the mandatory distribution of storage requirements but also through the rental income, auxiliary service income, etc., to enhance the economic benefits and the commercial value of the gradual emergence.
The mature market-based incentive mechanism is conducive to the healthy and sustainable development of the energy storage industry. Massa et al. [8] described the ESS business model from three aspects: the application of energy storage equipment, the role of potential investors in the market, and the revenue stream in operation. Aravind et al. [9] explored a business model for ESS on an islanded power grid. Ririka et al. [10] discussed what policies and regulations are needed for customer-side solar PV-ESS to mitigate the conflict of interest between grid companies and aggregators when providing services that maintain grid stability and resilience. The lack of an appropriate ESS market-oriented regulatory framework creates substantive, procedural, and institutional barriers to commercializing and integrating renewable energy storage technologies [11]. At the same time, the Chinese government has put forward requirements for the market-based development of ESS. 2022 On March 21, China’s National Development and Reform Commission and National Energy Administration issued a notice on the issuance of the “14th Five-Year Plan” Implementation Plan for the Development of Renewable Energy Storage. The statement pointed out that by 2025, the new type of energy storage will go from the early commercialization stage into the background of large-scale development with large-scale commercial application conditions. The new type of energy storage technology innovation capacity significantly improved, the core technology and equipment independent and controllable level greatly improved, the standard system is perfect, the industrial design is becoming more and more complete, and the market environment and business model are basically mature. By 2030, ESS will be fully marketized. The core technology and equipment of ESS will be independently controllable, the technological innovation and industrial level will be at the forefront of the world, the market mechanism, business model and standard system will be mature and sound, and the development of deep integration with all aspects of the power system will meet the needs of building a new type of electric power system. It will comprehensively support realizing the carbon peaking goal in the energy field as scheduled.
New type of energy storage is rapidly developing, but there are also many problems. First, the construction cost is high, a stable and reasonable market mechanism has not yet been formed, and the investment income model is immature, resulting in the price not being reasonably channeled [12]. Secondly, new type energy storage technology is rapidly developing, and there is a specific risk of safe operation. Equipment and scheduling modes need to be further explored to ensure the safety of the power grid [13,14]. Third, new type energy storage technology is diverse; the application level is very different for different types of energy storage, and there is a lack of adaptation to the operational characteristics of the management mechanism [15]. Scientific transformation of source-load-network storage scenarios to realize the economical and efficient use of energy storage equipment, the ESS operation mechanism needs to be market-oriented, and the development of ESS operation with the current stage of the market mechanism. The recent research on ESS scheduling mainly focuses on realizing the deep integration of ESS with power trading, transmission, and distribution. For example, establishing a nice ESS scheduling and operation mechanism to adapt to the needs of different application scenarios [16] and the cooperative operation and optimization management of ESS and renewable energy [17]. Previously, there have been many studies on the scheduling strategies of ESS on the grid side [18,19], generation side [20–22], and load side [23,24].
However, sorting out voltage levels, capacities, and specific ESS access scheduling processes needs to be more specific. In the existing research on market-based operation of ESS, the description and design of business models are mainly based on the realization of particular functions, the principle of market-based operation is not reflected, and there is no unified and systematic framework for business model analysis. Moreover, considering the operation mode and organizational structure of China’s power grid, the existing research lacks research on multi-level synergy at all dimensions of the dispatch center. China has the most extensive power grid system in the world, and the dispatching department is divided into five groups: national dispatching, grid dispatching, provincial dispatching, local dispatching, and county dispatching. this multilevel synergistic dispatching can improve the overall economy and stability. Similarly, how to realize ESS scheduling in such a large-scale power grid with all levels of electrical energy scheduling is of great research significance.
The main contributions of this paper are as follows:
• The operational characteristics of energy storage are summarized for different access points and access modes of energy storage in the smart grid;
• Summarized the commercial operation modes and scheduling operation models of energy storage for different grid access categories in market-oriented scenarios;
• A new energy storage scheduling method in the “national-division-provincial-local” scheduling architecture is proposed based on China’s unique power scheduling architecture.
The remainder of this article is organized as follows: In the second part, it summarizes the technical classification of renewable energy storage and the type of grid-connected mode and gives the energy storage operation model; in the third part, it analyzes the commercial operation mode of energy storage in smart grid and the operation and scheduling model in the market scenario; in the fourth part, it proposes a scheduling method of energy storage in the ``national-divisional-provincial-district" scheduling architecture of China’s electric power grid; and in the fifth part, it summarizes the concluding remarks and outlooks.
2 Energy Storage Classification and Modeling
2.1 Classification of Energy Storage
Energy storage mainly refers to the storage of electrical energy and the process of storing various forms of energy through some medium or equipment and releasing specific energy according to the application structure at the site. Energy storage technology is widely used in the four significant segments of power generation, transmission, distribution and consumption in the construction of smart grids. It is also a necessary supporting technology for developing renewable energy access, distributed power generation, microgrids and electric vehicles. According to the specific form of energy, energy storage can be categorized into chemical, electromagnetic, physical, etc., and the classification of energy storage technologies are shown in Table 1.
In microgrid and distributed power supply, the intermittent nature of renewable energy generation such as wind and light requires energy storage units with high energy density. In contrast, the rapid fluctuation of the load requires energy storage units with high power density, compared with a single ESS, usually choose a hybrid energy storage system [43] that complements the advantages of high energy density and high power density.
Classified according to the grid-connected operation mode, it is divided into power-side energy storage, grid-side energy storage and user-side energy storage. Under the current policy environment and demonstration application background, the main application scenarios of ESS on the power generation side include renewable energy smoothing, clean heating, and frequency control auxiliary service. The main application scenarios of grid-side ESS include grid power preservation, peak adjustment, and frequency control additional service. The main application scenarios of grid-side ESS include grid power preservation, grid peak shifting, frequency shifting other services, and grid rotation standby. Typical application scenarios of ESS on the user side include peak and valley spread arbitrage, park-integrated energy system formation, exceptional user power quality, demand response, etc. From the functional point of view, the renewable energy storage is firstly to store and provide energy, and secondly to regulate power. The energy storage grid-connected operation mode and function are matched, and the energy storage functions of different grid-connected operation modes are shown in Table 2.
Energy supply-demand imbalance and system contingencies are inherent characteristics of power system operation, and thus, frequency regulation is an intrinsic need of the power system. In the traditional energy structure, conventional units such as thermal power and hydropower regulate the short-term energy imbalance in the power grid by responding to AGC signals. As the global energy structure is adjusted, many renewable energy sources are connected to the grid to exacerbate the energy imbalance of the grid in a short period. The traditional energy sources, especially thermal power, are slow to regulate frequency and need to respond to the signals, which makes it challenging to satisfy the new demand for frequency regulation. The ESS can track load changes, fast response speed, and precise output control, and almost realize that the frequency control tracking curve is consistent with the command curve. Therefore, equipping ESS on the power generation side to provide frequency control auxiliary service independently or jointly with thermal power units can effectively enhance the grid frequency control capability, which is of great significance to improve the quality of power supply and ensure the safe and stable operation of the power grid.
At present, China’s central time-sharing tariff policy, a user-side ESS, can be based on time-sharing tariffs to take low charging and high discharge strategy, charging in the load valley and low tariffs, discharging in the load peak and high tariffs, the use of the difference between the peak and valley tariffs to obtain economic benefits.
In addition, energy storage has four quadrant operation characteristics: it can absorb or issue reactive power at any time of charging and discharging, maintain system voltage stability, and improve the power quality of the grid, thus reducing the investment in reactive power equipment of the distribution network. With the increasing demand for power quality from industrial, commercial, and other particular users, manufacturers can install energy storage to replace various types of power quality improvement devices and utilize the redundant capacity of energy storage to manage power quality problems such as low power factor and voltage imbalance that occur during the production process. Separate the ownership of energy storage resources from the right to use through the integration of idle energy storage resources to face the different application needs of multiple users, the use of regulating the complementary characteristics of demand in time and space to realize the shared use of energy storage resources, scientific integration of existing energy storage resources to enhance the utilization rate of energy storage and the rate of return [44,45].
2.2 Energy Storage Monolithic Modeling
2.2.1 Electrochemical Energy Storage Operation Model
Typically, when energy storage is configured to determine the capacity size of the warehouse, the rated capacity is generally considered, and the model of the charge state during the operation of the storage device can be expressed as:
In the formula,
(1) Power constraints for energy storage
The hourly charge/discharge of the energy storage is limited by the rated charge/discharge power of the energy storage and can be expressed as:
(2) Capacity constraints for energy storage
The energy storage charging state should be limited by the rated capacity of the energy storage to prevent overcharging and over-discharging from affecting the lifetime of the energy storage, so the capacity constraints of the ESS at the time should be satisfied:
(3) Energy storage charge/discharge state constraints
Assume that it is the energy storage’s charge/discharge state flag. Since there can only be one state in each period, its value is taken as the principle:
The formula
It is worth noting that the stored energy is self-discharged when in an idle float state, as given in Fig. 1, which plots the stored power for one charge/discharge cycle. Therefore, the energy recession in the graph is measured in terms of self-discharge rate:
where
(4) Energy storage charge/discharge quantity constraint
Figure 1: Energy diagram of stored energy in one charge/discharge cycle
Considering the charging and discharging losses, the charging and discharging quantities should be kept equal during the dispatch cycle of the ESS.
(5) ESS charge state constraints
where is the capacity of the energy storage system;
(6) Power system renewable energy power abandonment constraints
Considering the power-side energy storage is mainly applied to renewable energy supporting energy storage and other scenarios, because for the power-side energy storage, it is also necessary to include the system’s renewable energy abandonment rate into consideration, that is, the renewable energy abandonment rate is controlled within the limit:
where
(7) Nodes are allowed to install energy storage-rated power and capacity constraints
Considering that the ESS needs to follow the grid security standards when accessing the grid, in addition to avoiding the waste of investment in energy storage resources, the installed energy storage power and capacity need to be constrained according to the load density of the layout location, i.e., the system is optimized for peak shaving and valley filling.
where {
There is life loss during the operation of energy storage, which is reflected by the number of charge/discharge cycles, which can be estimated by the depth of discharge (with different depths of release, the number of times the energy storage can be cycled is different, and the actual service life of the energy storage battery is also different). Define the depth of discharge of the kth cycle of the energy storage battery as DODk, the total number of equivalent life depreciation of a storm in a day’s operating process can be expressed as:
Ndcyc is the number of charge/discharge cycle cycles per day for the energy storage battery; Ndcyc (DOD1) is the number of processes corresponding to reaching the end of life when the depth of discharge is 100%. The profit of energy storage through tariff arbitrage can be expressed as:
The depreciation factor, operational and maintenance (O&M) cost factor, and payback factor for the energy storage investment (all as a function of the optimization variable
where depreciation cost and O&M cost can be directly amortized to each year over the life of the investment since depreciation is generally calculated based on the average life method and O&M costs can be calculated based on minimization.
2.2.2 Pumped Storage Operation Model
For pumped storage systems, there is water hammer pressure in the water diversion system of the pumping and storage unit, which affects the dynamic performance of the unit speed control system. The water and pipe walls are elastic. Some studies have cited an approximate flexible water hammer model to describe the water diversion system, and the transfer function of this model is shown in Eq. (14).
where
The pumped storage system is constrained by reservoir capacity and operating power during operation.
In the formulas:
Constraints of pumped storage units can be expressed as:
where
where
2.2.3 Hydrogen Energy Storage Operation Model
The photovoltaic hydrogen storage part includes a photovoltaic power generation unit, electrolytic tank, hydrogen tank, and fuel cell, in which the electrolytic tank is responsible for converting electric energy into hydrogen energy, and the fuel cell is accountable for converting hydrogen energy into electric power. The heat energy generated by these 2 devices can be supplied to heat load or stored in the heat storage device. Through the cogeneration characteristics of the hydrogen energy storage device and the high-quality heat supply of the solar thermal collector unit, the operation mode of heat to electricity can be broken, and high heat and power supply efficiency can be realized.
An electrolytic tank is a device that electrolyzes pure water into hydrogen and oxygen, which can convert electrical energy into hydrogen energy, and its energy conversion characteristic model can be simplified and expressed as:
where
The hydrogen tank can be used to store the hydrogen produced by the electrolytic tank, and its storage capacity model can be simplified as follows:
where
In contrast to electrolytic tank, fuel cell converts hydrogen energy into electrical energy, and its energy conversion characteristic model can be expressed as follows:
where
2.2.4 Compressed Air Energy Storage Operation Model
Compressed air energy storage (CAES) system consists of compressor, turbine, heat exchanger, and gas reservoir. When the system releases energy, the flow rate of the compressed gas in the pool is controlled by adjusting the opening L of the regulating valve, which enters the heat exchanger for heating and realizes the power generation of the system through the work done by the turbine.
(1) Gas storage model
Let the CAES gas storage reservoir store high-pressure gas as a constant volume process, and the air as an ideal state. According to the law of conservation of mass and energy, the expression for the trend of pressure and temperature changes in the gas reservoir during the energy release stage is:
where
(2) Regulating valve model
Assuming that the mass flow rate Q (L) at the rated power output of the CAES is only related to the regulating valve opening L, and utilizing the percentage to express the valve opening, the relationship between the regulating valve opening and the mass flow rate can be approximated as an exponential function at this time.
where
The transfer function of the control valve opening actuator is:
where
(3) Heat exchanger model
Release of energy, each level of heat exchanger back to the heat after the air temperature is:
where i represents the number of turbine stages;
(4) Turbine model
Turbine outlet air temperature and unit mass of air through the turbine work expression is:
where
CAES output power expression is:
if
3 Market-Based Operation of Energy Storage in Smart Grids
With the gradual increase in the penetration of renewable energy generation, some conventional power sources are replaced by renewable energy sources, the system flexibility regulation capacity is not sufficient, the power system will face more tremendous peak pressure, resulting in wind abandonment, load shedding and other phenomena, so it is vital to enhance the enthusiasm of energy storage to participate in the flexible regulation of the power system through market means. This section compares different energy storage market-based incentive mechanisms. It summarizes the trading forms and profit-making methods of energy storage participating in various markets such as the electric and auxiliary service markets.
3.1 Business Models for Energy Storage Operation
The functional role of energy storage in the power system is shown in Table 3. The energy storage business model is closely related to many factors, such as policy support, electricity price system, type of energy storage, initial investment, operation main body, subsidy method, operation and maintenance and payback period cost. Despite the rapid growth of installed energy storage scale, China’s renewable energy storage is still in the early stage of commercialization, the market mechanism and business model are immature, coupled with China’s low electricity price and slight price difference, resulting in energy storage project profitability difficulties, facing high cost, complex integration, poor security and other issues [46,47]. It has become particularly urgent to explore incentives for energy storage to participate in the market to form a business model and actively find a breakthrough point. This section summarizes the practical experience of developing energy storage business models in China [48–50].
3.1.1 Cost Composition of Energy Storage Equipment
An electrochemical energy storage power station’s entire lifecycle process includes the project construction and operation phases [51–54]. On one hand, it is essential to analyze the cost composition of the electrochemical energy storage power station. On the other hand, examining the parameters influencing the calculation of various costs is crucial.
(1) Construction cost
The construction cost of an energy storage power station, also called system cost, pertains to the price of a unit capacity of the ESS. It primarily consists of equipment installation costs (including battery costs) and construction costs (excluding land costs).
Energy storage equipment comprises batteries, battery management systems, energy storage inverters, and distribution systems, among other components. The acquisition costs of these devices collectively constitute the equipment installation cost. An electrochemical ESS is primarily composed of a battery pack, a Battery Management System (BMS), an Energy Management System (EMS), a Power Conversion System (PCS), and other electrical components.
The battery pack cost is a primary expense within the electrochemical ESS, representing a critical focal point for future industry chain technological iterations and cost reduction efforts. According to data from HighTech Lithium, within a complete electrochemical ESS, the battery pack cost accounts for the highest proportion, reaching up to 67%. Following this, the energy storage inverter constitutes 10%, while the BMS and EMS account for 9% and 2%, respectively.
(2) Charging cost
The charging cost of an energy storage power station refers to all the expenses incurred during the charging process. Due to the inability to achieve 100% energy conversion efficiency during charging, costs are generated from energy losses. Therefore, this cost component primarily depends on the energy conversion efficiency. Calculating the charging cost for energy storage involves considering factors such as charging electricity prices, equipment utilization efficiency, annual discharge capacity of the ESS, and energy conversion efficiency parameters.
(3) Operational labor costs
In certain situations, the operation of energy storage equipment may require manual intervention. Within a specific range, fixed labor costs are unrelated to the size of the stored capacity, and their total remains constant. Variable labor costs are directly proportional to the frequency and duration of storage usage. In many cases, the accounting of labor costs for battery energy storage stations is primarily determined by the size of the equipment and its storage capacity, with no clear standard value currently established.
(4) Maintenance costs
An energy storage power station’s operating and maintenance costs are the expenses required to maintain the station in good standby condition. These costs include solar panel cleaning expenses, station management, and maintenance fees. Regardless of the amount of storage used, fixed maintenance costs remain the same. Variable maintenance costs are proportional to the frequency and duration of storage usage. Operational maintenance costs are generally calculated by multiplying the initial investment by the operating maintenance rate. Considering that the lifespan of ESS is limited, there will be a certain amount of wear and tear during operation, resulting in costs associated with the system’s lifespan. Therefore, when calculating the operating and maintenance costs of an energy storage power station, it is necessary to comprehensively consider parameters such as the total construction cost reduction ratio, operational maintenance rate, unit energy construction cost, and energy storage capacity.
(5) The cost of industrial electricity for energy storage applications
The industrial electricity cost for energy storage applications refers to the cost of electricity used by an energy storage power station for its energy storage purposes. It includes the cost of externally purchased electricity incurred to keep the energy storage power station running, encompassing purchased electricity costs, self-use electricity costs, and related expenses such as salary and management fees. In calculating the industrial electricity cost for energy storage applications, factors such as the annual average electricity consumption rate, the proportion of electricity used for energy storage purposes compared to the total industrial electricity consumption, charging electricity prices, and energy storage capacity need to be considered.
(6) The cost of replacing energy storage batteries and equipment
In electrochemical energy storage projects, the batteries used as energy storage components often require frequent replacements due to their limited cycle life. This leads to the cost of replacing energy storage batteries and equipment. Typically, the lifespan of batteries and equipment is shorter than the overall project cycle of an ESS. Therefore, the replacement cost of batteries needs to be considered during the project’s operational period. This includes acquiring updated materials and equipment, installation expenses, labor costs, and other related expenditures.
The replacement cost is considered a variable cost, and its magnitude depends on the battery’s lifespan and frequency of use. For example, if we believe a lithium-ion battery (such as lithium iron phosphate) with a cycle life of 6000 cycles and charging/discharging twice a day, the battery would need replacement every 10 years. It is economically and environmentally impractical to replace all batteries when they exceed their helpful lifespan.
Therefore, in practical operation, it is expected to supplement a specific capacity each year based on the battery’s annual degradation rate, ensuring the available power of the ESS. The cost of replacing energy storage batteries and equipment can be calculated by considering the annual cost of battery replacement, energy storage device capacity, and energy storage device conversion efficiency. Factors affecting the yearly battery replacement cost include replacement cost, replacement cycle, return on investment period, discount rate, battery charge-discharge cycle count, and the number of operating days per year.
Due to the limited number of charge-discharge cycles batteries can undergo, and the gradual degradation of battery capacity, frequent replacements are required during the operation of ESS. Therefore, it is necessary to consider the cost of battery capacity degradation and the time value of funds. However, the lifespan of different energy storage batteries varies, and their actual lifespan is related to factors such as charge-discharge depth and cycle count, which introduces uncertainty. Hence, this parameter is determined based on its influencing factors in practical applications.
(7) Assessment cost
From the perspective of the entire lifecycle cost, the assessment costs not considered in traditional cost pricing models are indispensable. According to the Shandong Power Grid Photovoltaic Power Station Scheduling Management Regulations (Trial), photovoltaic power stations must strictly adhere to the daily power generation scheduling curve and dispatch instructions issued by the provincial dispatch. They should promptly adjust active power output, and any deviation between actual production and scheduling plans is subject to assessment costs according to the specified curve. The calculation of assessment costs involves scheduling assessment fees, electricity penalty prices, planned output of the solar-storage power station, and the grid-connected power of the solar-storage system.
(8) Disposal cost
When the various components of an ESS reach the end of their lifespans, the funds invested in their harmless disposal constitute the disposal cost. This cost mainly comprises two aspects: environmental expenditure and equipment residual value. The cost incurred in recycling batteries represents the environmental expenditure, while the initial investment cost and recycling coefficient will determine the equipment’s residual value size.
Currently, in China, the industry for resource recovery of batteries has not fully developed and is still in the early stages of growth. Due to this reason, a significant portion of waste components does not receive effective disposal. Such waste poses a substantial threat to the natural environment and human health. Existing methods for handling waste batteries include solidification and burial, storing in abandoned mines, and resource recovery. Based on international practices, retirement costs associated with any storage system should not be overlooked.
After the end of their lifespan, batteries must be dismantled to remove chemical substances. Ideally, the dismantled batteries and their chemical components can be recycled, offsetting some losses incurred in dismantling and disposing of hazardous substances. However, the ultimate costs associated with removal should be included in the overall price of the energy storage power station.
In the foreseeable future, the impact of recovery value on the economic viability of battery energy storage is expected to increase, driven by the establishment and improvement of battery recycling mechanisms. For example, in the case of lead-acid batteries, advancements in lead recovery technology now allow for 100% recovery, and the residual value of equipment can reach 20% of the initial investment. On the other hand, recycling waste lithium batteries faces numerous challenges. The recycling technology is complex, expensive, and currently lacks a well-established solution, resulting in unclear recovery value and equipment residual value that can be considered negligible.
Therefore, in estimating the disposal cost of energy storage, it is essential to consider parameters such as unit capacity purchase price, rated capacity of the ESS, unit power inverter price, unit power auxiliary equipment cost, and residual value rate. These considerations are crucial for a comprehensive assessment of the economic aspects of battery energy storage, considering both the initial investment and potential recovery value at the end of the system’s lifecycle.
(9) Other cost
The cost of an energy storage power station also encompasses financial fees, taxes, and other expenditures. Economic costs primarily involve the interest expenses incurred from long-term energy storage power station loans. The calculation of interest expenses considers parameters such as the loan ratio, scheduling period, repayment term, loan interest rate, and initial investment.
Tax expenditures must be considered during the energy storage power station’s operational phase. Taxes primarily include value-added tax, income tax, and additional sales taxes. The other sales taxes include city maintenance and construction tax and education surcharges.
Before the opening of the spot market, power balancing could be achieved through a broadly defined peaking auxiliary service dispatch organization that could cope with the volatility and uncertainty of net load. However, system power balancing needs to be realized through competitive bidding and trading [55]. In the spot market situation, grid-side energy storage, as an independent market entity, needs to participate in spot market transactions to participate in power system peaking [56,57].
(1) Electricity market
Independent energy storage power station refers to an energy storage power station that has the condition of direct control of dispatching, signs a grid connection agreement directly with the power grid company as an independent market entity, commits to belong to the management of the power grid company, and signs a contract with the power grid enterprise and relevant parties such as the appropriate power generation enterprises or power users according to its access location, agreeing on the rights and obligations of each party. Its source of income is shown in Fig. 2. The current spot market spread arbitrage mechanism is gradually improved, 2022 day spot price difference of more than 1 RMB/kWh sometimes appears, for the independent energy storage power station using spread arbitrage to obtain greater profits has brought the space.
Figure 2: Sources of revenue for independent shared energy storage
There are 2 main ways to generate revenue related to independent energy storage power, i.e., provinces that have not opened the spot market realize the value through peak shifting, and areas that have opened the spot market learn the real-time value through node spread arbitrage. Since the ESS has power characteristics when discharging and load characteristics when charging, in electric energy trading, the grid-side energy storage plant is both the supply side and the demand side, and it needs to determine the charging and discharging power in each period through market bidding. As the demand side, too low an offer may lead to unsuccessful bidding and charging, and too high an offer may lead to high charging costs, i.e., grid-side storage power stations have to take into account the success of the bidding and charging fees to make decisions on the amount of charging power to be declared for each period and the displayed price; as a supplier, too low an offer may lead to insufficient discharging revenues, and too high an offer may lead to unsuccessful bidding and discharging, i.e., grid-side storage power stations have to consider the success and cost of charging to decide on the charging power declaration for each period. Grid-side energy storage power stations must consider the success of bidding and discharge revenue to determine the declared amount of discharge power and said price for each period. In Shandong, the average 2-h maximum price is 0.7 RMB/kWh, and the average minimum fee is 0.1 RMB/kWh. Considering the capacity tariff (0.099 RMB/kWh), which needs to be borne by the energy storage charging, as well as some additional costs under the rules of spot trading (about 0.02 RMB/kWh), the charging/discharging tariff difference that can be obtained by one 2-h energy storage power station is approximately 0.5 RMB/kWh. 85% cycle efficiency, running for 330 days a year, with 1 charge/discharge cycle per day, the annual spot market gain can be about 24.81 million RMB.
(2) Ancillary services market
At present, the common forms of auxiliary services of renewable energy storage are mainly two types: peak shifting and frequency shifting (including primary and secondary frequency going), with different specific benefits in each region, but rise turning mostly charging compensation according to the amount of peaking power, with the price ranging from 0.15 to 0.8 yuan/kWh; and frequency shifting is mostly compensation according to the frequency shifting mileage, according to the unit responding to the number of AGC turning commands, and giving the frequency shifting payment of 0.1 to 15 yuan/MW of frequency control compensation.
Peaking auxiliary service compensation is the most essential means for independent energy storage power plants to obtain revenue. Due to uncertainties such as wind power output and load forecast errors, the power system must reserve a certain amount of upward and downward adjustment capacity for intra-day balancing adjustment. Under the spot market environment, the system’s upward and downward adjustment capacity must also be determined through auxiliary service transactions. In addition to the single compensation price, the frequency of use is also vital in determining its profitability. Taking a 100 MW/200 MWh energy storage power station in Shandong Province as an example, the independent energy storage power station has a peak compensation of 0.2 yuan/kWh, the guaranteed calling hours of 1000 h/a, the annual salary can be 20 million yuan.
As shown in Fig. 3, in the charging state, the ESS may provide a down-regulated capacity by reserving the ability to increase the charging power and an up-regulated capacity by decreasing the charging power. In the discharging state, the ESS may provide an up-regulated accommodation by reserving the ability to increase the discharging power and a down-regulated capacity by decreasing the discharging power. Since the regulated degree that the ESS can provide in a specific period directly depends on its current charging and discharging control, the grid-side energy storage plant needs to take into account the revenue from electric energy and the income from auxiliary services to decide on the declared upward/downward regulated capacity and said price in each period. In addition, considering the correlation between energy trading and auxiliary service trading, grid-side energy storage plants need to make optimal coordination decisions between multiple market transactions from a holistic perspective, and formulate a correlation offer strategy to maximize revenue.
Figure 3: Schematic diagram of the regulation capacity that can be provided by energy storage
Take the Shanxi provincial power grid as an example [58]. Shanxi local power grid primary frequency control demand times, short cycle, speed and precision requirements are high, electrochemical energy storage frequency control speed, adjustable capacity, can be used as a high-quality frequency control resource. Grid secondary frequency control service requirements are relatively high, the minimum length of 2 h and 45 min, the advantages of an electrochemical ESS are challenging to play, so the primary frequency control on the speed of response and accuracy of the requirements of the strict, more favorable to give play to the advantages of electrochemical energy storage frequency control. Therefore, one-time frequency regulation requires rigid response speed and precision, which is more conducive to utilizing the benefits of electrochemical energy storage frequency regulation. The typical provinces of China’s independent energy storage participation in the auxiliary service market revenue are shown in Table 4.
In addition to the above model, the fire storage joint frequency regulation has become one of the first commercialization areas in the energy storage industry. Fire storage joint frequency control refers to the thermal power plant through the addition of energy storage to enhance the thermal power plant’s regulation performance, to make up for the disadvantage of the slow climbing speed of thermal power units, to achieve the flexibility of thermal power units to transform. The primary sources of revenue of the project include secondary frequency control compensation and reduction of appraisal fees, with secondary frequency control compensation being the primary source. The National Energy Administration previously issued the Implementing Rules for the Management of Grid-Connected Operation of Power Plants and other documents, which established a compensation mechanism for the thermal storage and transfer project. With the recent increase in electrochemical energy storage frequency regulation projects, thermal power plant configuration electrochemical energy storage to provide frequency regulation and other auxiliary services has become one of the highest economic energy storage application modes. But compared with electric energy regulation, frequency regulation additional service market space is small, a large number of energy storage technology influx into the frequency regulation market is bound to frequency regulation prices caused by a more significant impact. In Guangdong Province, for example, the secondary frequency control compensation revenue includes frequency control capacity revenue, and frequency control mileage revenue 2 parts. Early commissioning of the projected revenue is higher, the short-term cost recovery, but with the increase in the project and the emergence of new market rules (September 01 2020 from the implementation of the new market rules, on the K value of the open (m+1) square root), the market clearing price significantly lower, most of the late commissioning of the project can not recover costs. From a short-term perspective, the revenue will also be more secure, some of the larger installed capacity, their regulatory solid capacity of thermal power units in the energy storage configuration of the transformation. From a long-term perspective, with the top-level design, supporting mechanisms continue to improve, fire storage and joint regulation in the future will also be expanded to a frequency regulation market, thus further broadening the profitability channels, revenue space will be more precise.
The spot market mechanism is the basis for market players such as grid-side energy storage power plants to participate in trading of electric energy and auxiliary services. Based on the practical experience of domestic and international power markets, the spot market involved consists of the day-ahead electric energy market, the day-ahead additional service market, and the intraday balancing market [59,60]. The bidding guidance strategy for energy storage to participate in the day-ahead-real-time two-stage, multi-scenario application of the electricity spot market has not yet been fully formed. Still, there are relevant studies in the literature. Kazemi et al. [61] proposes a risk-based bidding strategy for energy storage participation in the day-ahead power market, the rotating reserve market, and the intraday balancing need to optimize the offer decision considering the market price and reserve demand uncertainty. Moreno et al. [62] and Akhavan-Hejazi et al. [63] studied the operation of energy storage in the combined day-ahead electric energy and ancillary services market. It used probability distribution and multi-scenario generation to simulate the impact of uncertainty in the real-time market and optimize the bidding decision scheme, respectively. Based on the above spot market mechanism, the grid-side energy storage plant spot market trading process shown in Fig. 4 is obtained by considering the electric energy and auxiliary service transactions from the two-time scales of day-ahead and intraday.
Figure 4: Grid-side energy storage plant spot market trading process
Before the day of operation, energy storage power stations are required to submit to the trading center not only the declared charging and discharging power quantity and announced price for each time slot; they are also required to submit the stated capacity and displayed price for upward/downward adjustment for each time slot. The trading center will clear the market for electric energy in the previous day based on the information of the electric energy offer received within the specified time, and get the last price electricity for each time slot on the operation day as well as the bidding status of each market participant; and clear the market for auxiliary services in the previous day based on the information of additional service offer, and get the clearing price of other services for each time slot on the operation day (upward adjustment of the clearing price of the service and downward adjustment of the clearing price of the service) as well as the winning status of the bidding status of each market participant. Based on the winning bids in the previous day’s market, the energy storage power station arranges the charging and discharging power for each time slot on the operation day. During the day, the trading center performs intraday balancing market clearing based on the net load deviation. It obtains real-time tariffs for each period and upward/downward capacity calls. The storage plant adjusts the charging and discharging power for each period based on the regulation capacity called up in the intraday balancing market.
3.1.4 Quotation Strategy Model
The structure of the two-tier trading decision model with the energy storage plant as the main body of the strategy is shown in Fig. 5.
Figure 5: Structure of the trading decision model
The upper layer model is the energy storage plant trading decision model, which generates offers for various spot market trading types. Based on the spot market trading process of grid-side energy storage power plants in Section 3.1.2, to maximize the revenue from their participation in the day-ahead energy market, day-ahead auxiliary service market and intraday balancing market; based on the day-ahead energy market clearing price (day-ahead price), day-ahead additional service market clearing price (up/down service clearing price) and intraday balancing market clearing price (real-time price); and on the premise of meeting the storage system operation constraints, the trading decision model of energy storage power plants is constructed to optimize the offer strategy of storage power plants. On the premise of meeting the conditions on the operation of the ESS, the trading decision model of the energy storage power station is constructed to optimize the grid-side energy storage power station offer strategy, including the declared charging and discharging power quantity and said price for each time slot in the previous day’s energy market bidding and the stated upward/downward adjustment announced capacity and said worth for each time slot in the last day’s auxiliary service market bidding. It also transmits to the lower level the energy storage plant’s offer for each period of electric energy and additional services.
The lower model is the spot market clearing model, which contains two parts: the joint clearing of the day-ahead energy market and auxiliary services market, and the clearing of the intraday balancing market. In the day-ahead collective clearing, due to the wind power output is not controllable as well as China’s current wind power guaranteed purchase policy, wind farms do not participate in the day-ahead electric energy market offer, but the wind farms’ day-ahead predicted output as a “negative load”, superimposed to get the net load of the system. To maximize social benefits, based on the offer strategy of energy storage power plant, thermal power unit generation offer and upward/downward adjustment offer, netload demand offer and upward/downward adjustment demand offer, and under the premise of meeting the constraints of the power system operation and market transaction constraints, we construct a joint clearing model of the day-ahead electricity energy market and auxiliary services market. Through the model clearing, we obtain the winning bidding capacity of charging and discharging and upward/downward adjustment of energy storage plant, as well as the day-ahead tariff and the upward/downward adjustment of service clearing price. In the intraday balancing clearing, to minimize the system balancing cost, the intraday balancing market clearing model is constructed based on the upward/downward adjusted capacity and electric energy quotes won by each market participant, and taking into account the wind abandonment quotes and load shedding sections to meet the system net load deviation. Through the model clearing, we get the calling situation of energy storage’s upward/downward bidding capacity and the real-time electricity price. The lower-level market clearing results are fed back to the upper level for calculating the actual revenue of the energy storage plant.
3.2 Functions of Energy Storage in Power Systems
The main function of ESS in the generation, grid and demand side is shown in Fig. 6.
Figure 6: Main functions of ESS in the generation, grid and demand side
Wind power, photovoltaic and other renewable energy development is rapid. Still, due to the mismatch between resource-rich places and power consumption, technical factors, institutional barriers and other factors, the renewable energy consumption needs to be solved by the industry’s development problems. The construction of ESS in large ground power stations can store renewable energy capacity when wind and light resources are sufficient, and transmit it out when wind and light resources are insufficient, thus effectively alleviating the phenomenon of renewable energy power stations “abandoning wind, abandoning light and restricting electricity”, and improving the self-consumption capacity of renewable energy power stations. On this basis, through the intelligent control system with the wind farm operation strategy can promote the renewable energy power plant and power grid friendly interaction, improve the ability of the grid to accept renewable energy and the overall quality of operation, on the other hand, supporting the energy storage to enhance their utilization needs to meet the regulation needs of more participating subjects [64].
As weather and time factors affect renewable energy power generation output, the output size changes quickly and fluctuates wildly. As wind power and photovoltaic grid-connected capacity grow, their instability and other problems must be solved urgently. The construction of an ESS in renewable energy farms can help to suppress the fluctuation of renewable energy power generation when the external environment changes, stabilize the output level, smooth the power generation curve, and meet the renewable energy power assessment. To improve the economy and scheduling flexibility of renewable energy farms on the power generation side, Li et al. [65] relied on the complementarity of CCHP units and virtual power plants to various energy sources. It proposes a two-layer robust game model to cope with the uncertainty of power generation from renewable energy farms, and to smooth the output volatility of the farms, to improve the grid-friendly capability of renewable energy farms. Shi et al. [66] analyzed the fluctuation characteristics of wind power output from both time and frequency domains. The degree of fluctuation is extracted and shown as a quantization index (QI). Based on the QI clustering, the wind power scenario with the most significant power fluctuation is selected as the “worst performance”, based on which the scheduling timeframe and energy storage capacity and charging/discharging power can be determined. Roy et al. [67] took a hybrid ESS composed of batteries and ultracapacitors as the research object. It uses a multilayer perceptron artificial neural network for pre-temporal wind speed prediction, and an adaptive neuro-fuzzy inference system to design the state of charge (SOC) controller for energy storage, to accurately estimate the grid reference power in a single dispatch cycle, and to ensure that the ESS accomplishes the following wind farm operation instructions for each dispatch cycle and leave a certain capacity margin. In addition, the particle swarm optimization technique is used to optimize the life cycle cost of the ESS based on its discharge depth level, and thus minimize the cost of the dispatchable solution for the wind storage system.
The construction of ESS on the grid side can have a peak shifting capacity twice as much as its own installed capacity. After scale configuration, it can provide efficient peak shaving and valley filling services, realize load shaping and load transfer, which is equivalent to the improvement of the load characteristics for the grid, and effectively alleviate the pressure of grid peak shifting. Xie et al. [68] proposed to present a blockchain-based grid-side energy storage market-oriented trading model and mechanism, combining existing policies and market rules, carrying out research on energy storage participation in auxiliary services, and designing a market mechanism for energy storage participation in peak shifting and frequency regulation additional services to improve the flexibility of the power system and promote clean energy consumption. In addition, as a fast and flexible solution, grid-side energy storage has been increasingly deployed to facilitate the penetration of renewable energy. Zhang et al. [69] proposed a new two-level optimization model for grid-side energy storage, which considers the operation of grid-side energy storage under the uncertainty induced by fluctuating wind power generation. The lower level uses an accurate battery cycle life model to simulate the long-term process of grid-side energy storage. In contrast, the upper level performs marginal economic utility analysis and storage capacity optimization to achieve the optimal capacity allocation of energy storage. Bence et al. [70] proposed the concept of local market and the corresponding dynamic tariff system, considering the interaction between local and retail markets and the network estimation state, and establishes an over-the-grid fee trading platform to facilitate P2P energy trading for all market participants.
Integrated energy systems with flexible operation and dispatchable performance are the primary trends of current energy development. As the system generally distributed power generation occupies a large proportion, but distributed photovoltaic or decentralized wind power is intermittent and fluctuating. Therefore, the installation of the ESS in the integrated energy system can maximize the use of distributed renewable energy, reduce the diesel generator running time, improve the reliability of the power supply and reduce the environmental pollution at the same time, the ESS can be played to obtain the maximum economic returns. Sun et al. [71] built a flexible multi-energy sharing platform, integrates different electricity, heat and natural gas storage resources scales in a comprehensive energy system, and designs an interaction mechanism between hybrid energy storage and renewable energy power stations. Wang et al. [60] established a battery-sharing model at the park level, and to improve the utilization rate of energy storage, Bukhsh et al. [72] integrated the energy storage of small-scale commercial and domestic users within the community for sharing and, in turn, pools the information to the higher-level coordinator for unified dispatch management. When the user-side energy storage participates in demand response, on the one hand, it can gain revenue through the difference in electricity prices. On the other hand, it can also obtain demand response policy subsidies. In [73], part of the energy storage is used to respond to the tariff to reduce the cost of electricity, and the other part is used to provide services to the grid to realize the dynamic allocation of energy storage resources. At the same time, the participation of user-side energy storage in demand response can alleviate the government’s financial pressure, and by guiding user-side energy storage to participate in peak-shaving demand response in summer and valley-filling demand response in winter, it can massively reduce the country’s investment expenditures on power plants. Yan et al. [74] proposed a stepwise multi-price multi-time demand side response (DSM) model. Second, energy-based and power-based energy storage devices are used together. Supercapacitor devices are used to stabilize the fluctuations of distributed power sources. Lithium iron phosphate batteries are used to reduce the load shortage rate. Line losses are also considered in the optimization objective. The location and capacity of hybrid energy storage devices are discussed. Finally, time-of-day tariff strategy is considered, power compensation cost for interruptible loads is calculated, and DSM strategy is introduced to realize joint planning with ESS.
4 Smart Grid Energy Storage Scheduling Architecture
4.1 Structure of the Provinces and Territories
In the previous scheduling of energy storage in the context of source load network storage, a high proportion of renewable energy access was considered [75,76], flexible resource utilization [77–79], and energy storage overcharging/discharging [80,81], without considering the use of multi-level collaborative scheduling in different provinces and regions to improve management efficiency, economy, and stability. The application scenario of the proposed ``source-load-network-storage" [82] is shown in Fig. 7. The framework can comprehensively perceive the characteristics and response characteristics of adjustable loads, such as distributed power sources, based on big data, clustering analysis and other advanced applications deployed on the provincial regulator cloud. It further identifies the complete power supply paths of necessary users, analyzes the redundancy degree of power supply points at each voltage level, and evaluates the reliability and risk of power supply. spatial and temporal characteristics and response characteristics of adjustable loads such as energy storage and electric vehicles. In the application scenario of source-network-load-storage interaction, the national regulator can directly control the large-capacity ESS with 1000 kV access; the sub-regulator can directly control the medium-capacity ESS with 500 kV access; the provincial regulator can directly control the small-capacity ESS with 220 kV access; and the local regulator can manage the user-side aggregated energy storage, a specific capacity of the grid-side energy storage, and wind-scenic ancillary storage with an access voltage level of 110 kV.
Figure 7: Interactive application scenario of source-network-load-storage
Utilizing the source-network-load-storage active cooperative control application deployed in the provincial regulator’s cloud, it obtains the control demands for the power market, grid peaking, and overloading of internal sections in the local regulator’s spot market system. It realizes the safe and stable operation of the grid frequency and inner compartments, as well as the smooth execution of the results of the market clearing using the active power control of the new type of regulating resources, such as the wind, light, and water, the energy storage, and the adjustable loads, throughout the entire network. Then, based on the leading distribution integrated AGC control objectives and the primary distribution network maintenance data, it calculates the regulation strategy and plan for the source network, load and storage, and sends these optimized control objectives to each local control system. According to the system operation demand, power market trading varieties and communication resources, the control objectives on different time scales are formulated, which can cover ultra-short-term (5 min to multiple hours in the future, with 5 min granularity), short-term (1~8 days in the future, with 15 min granularity), and medium-long-term (1 month to 1 year in the future, with 1 h granularity).
The main grid application of the local regulator carries out load-assisted decision analysis based on the cloud data and realizes the issuance of adjustable capacity regulation strategies for local power plants, power-side energy storage, large industrial users, etc., by load control, and interacts with the distribution grid application module with information such as adjustable resource control strategies. Combined with the various types of aggregated resources of source network, load and storage provided by the distribution grid application, the grid operation mode is optimized according to the current and future operation status of the grid, and the calculated strategy is sent down to the distribution grid application, which carries out control by the distribution grid application.
The ground distribution grid application builds load-side resource aggregation modeling, monitoring, and control functions, and realizes monitoring, analysis, and control functions for distributed power sources, charging piles, controllable loads, and other equipment. The distribution grid application aggregates and optimizes the control of different types and regions of controllable load resources to solve the problem of large number and variety of controllable load resources and small capacity of some single units. Finally, the cloud-based and local integrated main and distribution network optimization control objectives and strategies complete the source network, load and storage co-optimization and flexible control, thus realizing the integrated primary and distribution management in a wide area region.
4.2 Models of Energy Storage Participation
The model of energy storage participation in national sub-provincial and local dispatch refers to the use of energy storage technologies for the storage and release of electrical energy in the power system to balance the difference between supply and demand, stabilize grid operation, provide dispatch flexibility, and support a high percentage of renewable energy penetration. Energy storage participation can occur at the national level, the regional level, or even at the provincial level or smaller. The following are some standard models of energy storage participation in national sub-provincial and local dispatch:
(1) Frequency regulation and AGC [83]: Energy storage can achieve frequency regulation through rapid charging and discharging to stabilize the grid frequency. AGC system adjusts the output of generating units according to the changes in grid frequency, while energy storage can respond quickly to frequency fluctuations and support frequency regulation.
(2) Power balance: Energy storage can achieve harmony between power supply and demand according to the load changes in the power system and fluctuations in renewable energy, storing renewable energy when it generates excess power, and then releasing power when demand peaks, realizing the balance between power supply and demand.
(3) Large-scale energy storage power station [84]: Within the scope of the country’s sub-provinces and provinces, large-scale energy storage power stations can be constructed, such as Battery ESS or pumped storage power stations, as central energy storage devices to provide the power grid with Long-term energy storage capacity and flexible scheduling capacity for the power grid.
(4) Distributed energy storage system [85]: Distributed energy storage system installed in power users or small areas can provide local power scheduling and stability, improve the power system’s energy utilization efficiency and the power supply’s quality.
(5) Market-based operation [86]: The participation of energy storage in the scheduling of the national and provincial provinces and territories can also be realized through the market mechanism. Market-based operation incorporates the value of energy storage resources into the electricity market, and the storage and release of electricity through market transactions to achieve economic efficiency and system optimization.
The scheduling and operation architecture of energy storage devices is shown in Fig. 8 [87–89]. Firstly, each unit’s characteristic parameters and output status, the offer data of each team, and the peaking performance index are inputted to the trading market. Each energy storage participates in each market according to the following order, after which the instructions are sent to each energy storage power station through the dispatch center, and finally the power-side, grid-side and user-side energy storage acts. Intraday power balancing is realized using the intraday balancing market to invoke the upward/downward capacity determined in the day-ahead auxiliary service market to meet the net load deviation that may occur during actual operation. Day-ahead power balancing is organized in response to fluctuating changes in net load to achieve day-ahead power balancing. To cope with net load deviations, the Day-ahead Ancillary Services Market is organized for upward/downward capacity trading to provide regulation capacity for intra-day operations. Organize the intraday balancing market during the day to achieve intraday power balance by calling the regulation capacity determined in the day-ahead auxiliary service market based on the net load deviation during actual operation.
Figure 8: Dispatch process with ESS
The dispatch process with energy storage participation is shown in Fig. 8. Before the day of operation, energy storage power stations are required to submit to the trading center not only the declared charging and discharging power quantity and announced price for each time slot; they are also required to submit the stated capacity and displayed price for upward/downward adjustment for each time slot. The trading center will clear the market for electric energy in the previous day based on the information of electric energy offer received within the specified time, and get the last price electricity for each time slot on the operation day as well as the bidding status of each market participant; and clear the market for auxiliary services in the previous day based on the information of additional service offer, and get the clearing price of other services for each time slot on the operation day (upward adjustment of the clearing price of the service and downward adjustment of the clearing price of the service) as well as the winning status of the bidding status of each market participant. Based on the winning bids in the previous day’s market, the energy storage power station arranges the charging and discharging power for each time slot on the operation day. During the day, the trading center performs intraday balancing market clearing based on the net load deviation. It obtains real-time tariffs for each period and upward/downward capacity calls. The storage plant adjusts the charging and discharging power for each period based on the regulation capacity in the intraday balancing market. Finally the intraday balancing market releases the final dispatch initiative to the energy storage.
One of the power side energy storage is through the wind power, photovoltaic power station configuration of energy storage, based on the power station power prediction and storage charge and discharge scheduling, can be smooth control of renewable energy power generation, reduce the instantaneous power changes, reduce the impact on the grid. When the electricity load is low, the renewable energy generation surplus, energy storage power station can store more power in time, reduce the abandoned wind and abandoned light rate, and in the high electricity load, the stored power grid improves the renewable energy generation and consumption problems. The grid-side energy storage frequency regulation has higher accuracy, and its output power matches the automatic generation control (AGC) command to a high degree. Hence, the frequency regulation effect is better. The ESS is installed in the upstream of the grid-side line. When the line is blocked, the power that cannot be delivered can be stored in the energy storage equipment, and when the line load is less than the line capacity, the ESS will discharge to the line. Energy storage can be an alternative to upgrading or building new transmission and distribution equipment. Energy storage on the consumer side can be categorized into industrial, commercial, and household energy storage, generally used with distributed photovoltaics. Distributed photovoltaic power generation refers to facilities constructed in the vicinity of users and characterized by balanced regulation in the distribution system in the form of self-generation and self-consumption by users and on-grid access to surplus power.
The scheduling of energy storage is divided into horizontal and vertical management. Horizontally, it is divided into grid-side energy storage, power-side energy storage and user-side energy storage, which are categorized by application scenarios and accessed to the grid with a specific capacity. Vertically, it is divided into four levels, namely, national regulation, sub-regulation, provincial regulation and local regulation, where energy storage is accessed according to the specified voltage level, and then uniformly deployed by the dispatch center. In the future, the grid company will have a more precise definition of the capacity and voltage level of energy storage access, and better and more flexible control of its participation in the process of auxiliary services.
In terms of timing, it is possible to match the periods required to complete different closed-loop control actions according to the control timing requirements of grid auxiliary services to achieve initial synergy [90]. Further, for multiple control modes that meet the exact timing requirements, a collaboration mechanism that fully considers the comprehensive decision-making of various factors needs to be established, such as when a local fault generates intra-day hourly regulation and control requirements, power supply augmentation, grid operation adjustment, demand response, virtual power plant, energy storage invocation, etc., are optional. How to make a comprehensive decision based on the power balance, grid operation constraints, economy, environmental protection and other factors to realize the optimal scheduling of multi-mode combinations is a critical technology that needs to be studied in the new type of power system.
This review summarizes the current research status of energy storage market-oriented scheduling and operation strategy in the context of smart grid and the exploration of energy storage operation model and market-oriented incentive mechanism. It analyzes the dispatching and management requirements for energy storage by the national, provincial, and local regulators. It summarizes the energy storage regulation and operation management mode based on the “national, provincial, and local” multilevel coordination, management system architecture, and critical technologies in the interactive source-load, network, and storage scenario. In addition, a two-layer trading decision model with storage power station as the primary strategy for energy storage power station to participate in spot market trading and the basic operation model of energy storage in various scenarios such as power generation side, grid side, and user side are also analyzed, which can optimize the bidding strategy of energy storage to participate in the day-ahead market (including the electric energy market and the auxiliary service market) and the intraday balancing market, and obtain the charging and discharging behaviors and corresponding offer strategies to maximize the benefits of energy storage, and effectively motivate the energy storage equipment to participate in the day-ahead market (including the electric energy market and the auxiliary service market). The charging and discharging behaviors and corresponding bidding strategies that maximize the energy storage revenue can be obtained to effectively incentivize energy storage equipment’s cost recovery.
Acknowledgement: None.
Funding Statement: Funded by the North China Branch of State Grid Corporation of China, Contract No. SGNC0000BGWT2310175.
Author Contributions: The authors confirm contribution to the paper as follows: study design and draft manuscript preparation: Li Deng, Jiafei Huan, Wei Wang, Weitao Zhang, Yuan Huang, Yue Xiang; collection and analysis of policies and data: Liangbin Xie, Lun Dong, Jingrong Guo, Zhongping Li. All authors reviewed the results and approved the final version of the manuscript.
Availability of Data and Materials: Data will be made available on request.
Conflicts of Interest: The authors declare that they have no conflicts of interest to report regarding the present study.
References
1. Liu, J., Tang, Q., Su, Y., Li, T., Wang, Y. et al. (2021). Economic analysis of solid oxide fuel cell and its role in carbon peak, carbon neutralization process. 2021 4th International Conference on Energy, Electrical and Power Engineering, pp. 115–119. Chongqing, China. [Google Scholar]
2. Wang, Y., Wu, Z., Song, X., Duan, H., Tan, H. (2022). Calculation and emission reduction effect evaluation model of wind power lifetime carbon emission under carbon neutralization target. 2022 IEEE 6th Conference on Energy Internet and Energy System Integration, pp. 2182–2186. Chengdu, China. [Google Scholar]
3. Bebortta, S., Senapati, D., Panigrahi, C. R., Pati, B. (2022). An adaptive modeling and performance evaluation framework for edge-enabled green IoT systems. IEEE Transactions on Green Communications and Networking, 6, 836–844. 10.1109/TGCN.2021.3127487. [Google Scholar] [CrossRef]
4. Wu, X., You, L., Wu, R., Zhang, Q., Liang, K. (2022). Management and control of load clusters for ancillary services using internet of electric loads based on cloud-edge-end distributed computing. IEEE Internet of Things Journal, 9, 18267–18279.10.1109/JIOT.2022.3156954. [Google Scholar] [CrossRef]
5. Xie, J., Zheng, Y., Pan, X., Zheng, Y., Zhang, L. et al. (2021). A short-term optimal scheduling model for wind-solar-hydro hybrid generation system with cascade hydropower considering regulation reserve and spinning reserve requirements. IEEE Access, 9, 10765–10777. 10.1109/ACCESS.2021.3049280. [Google Scholar] [CrossRef]
6. Yang, R., Jin, J., Zhou, Q., Zhang, M., Liang, S. et al. (2023). Superconducting magnetic energy storage integrated current-source DC/DC converter for voltage stabilization and power regulation in DFIG-based DC power systems. Journal of Modern Power Systems and Clean Energy, 11, 1356–1369. 10.35833/MPCE.2022.000051. [Google Scholar] [CrossRef]
7. Liu, W., Liu, Y., Wu, L. (2023). Model predictive control based voltage regulation strategy using wind farm as black-start source. IEEE Transactions on Sustainable Energy, 14, 1122–1134. 10.1109/TSTE.2023.3238523. [Google Scholar] [CrossRef]
8. Massa, L., Tucci, C. L., Afuah, A. (2017). Acritical assessment of business model research. Academy of Management Annals, 11, 73–104. [Google Scholar]
9. Aravind, R. K., Gireesh, S. (2021). Role of policy in developing business models for battery storage deployment: Hawaii case study. Energy Policy, 159, 112605. 10.1016/j.enpol.2021.112605. [Google Scholar] [CrossRef]
10. Ririka, Y., Akihisa, M. (2023). We combined third-party ownership and aggregation business model for adopting rooftop solar PV-battery systems: Implications from the case of Miyakojima Island. Japan Energy policy, 173, 113392. 10.1016/j.enpol.2021.112605. [Google Scholar] [CrossRef]
11. Zhang, M., Yang, X. N. (2021). Administrative framework barriers to energy storage development in China. Renewable and Sustainable Energy Reviews, 148, 111297. 10.1016/j.enpol.2022.113392. [Google Scholar] [CrossRef]
12. Opathella, C., Elkasrawy, A., Mohamed, A. A., Venkatesh, B. (2018). A novel capacity market model with energy storage. IEEE Transactions on Smart Grid, 10, 5283–5293. 10.1109/TSG.2018.2879876. [Google Scholar] [CrossRef]
13. Faruhaan, A., Go, Y. (2021). Energy storage sizing and enhanced dispatch strategy with temperature and safety considerations: A techno-economic analysis. Energy Storage, 3, e260. 10.1002/est2.260. [Google Scholar] [CrossRef]
14. Li, B., Wang, H., Tan, Z. (2022). Capacity optimization of hybrid energy storage system for flexible islanded microgrid based on real-time price-based demand response. International Journal of Electrical Power and Energy Systems, 136, 107581. 10.1016/j.ijepes.2021.107581. [Google Scholar] [CrossRef]
15. Lindner, M., Peper, J., Offermann, N., Biele, C., Teodosic, M. et al. (2023). Operation strategies of battery energy storage systems for preventive and curative congestion management in transmission grids. IET Generation Transmission and Distribution, 17, 589–603. 10.1049/gtd2.12739. [Google Scholar] [CrossRef]
16. Wang, Q., Zhang, X., Xu, D. (2023). Source-load scenario generation based on weakly supervised adversarial learning and its data-driven application in energy storage capacity sizing. IEEE Transactions on Sustainable Energy, 14, 1918–1932. 10.1109/TSTE.2023.3258929. [Google Scholar] [CrossRef]
17. Abomazid, A. M., El-Taweel, N. A., Farag, H. E. Z. (2022). Optimal energy management of hydrogen energy facility using integrated battery energy storage and solar photovoltaic systems. IEEE Transactions on Sustainable Energy, 13, 1457–1468. 10.1109/TSTE.2022.3161891. [Google Scholar] [CrossRef]
18. Jiang, X., Jin, Y., Zheng, X., Hu, G., Zeng, Q. (2020). Optimal configuration of grid-side battery energy storage system under power marketization. Applied Energy, 272, 115242. 10.1016/j.apenergy.2020.115242. [Google Scholar] [CrossRef]
19. Gao, T., Liang, L., Liu, K., Xiong, D., Lin, Z. et al. (2021). Field exploration and analysis of power grid side battery energy storage system. IEEE Access, 9, 63213–63218. 10.1109/ACCESS.2021.3054620. [Google Scholar] [CrossRef]
20. Sun, E., Shi, J., Zhang, L., Ji, H., Zhang, Q. et al. (2023). An investigation of battery energy storage aided wind-coal integrated energy system. Energy Engineering, 120, 1583–1602. 10.32604/ee.2023.027790. [Google Scholar] [CrossRef]
21. Wang, Q., Zhang, X., Yi, C., Li, Z., Xu, D. (2022). A novel shared energy storage planning method considering the correlation of renewable uncertainties on the supply side. IEEE Transactions on Sustainable Energy, 13, 2051–2063. 10.1109/TSTE.2022.3179837. [Google Scholar] [CrossRef]
22. Wang, Y., Liu, J. (2022). Optimal capacity allocation of wind-light-water multi-energy complementary capacity based on improved multi-objective optimization algorithm. Frontiers in Energy Research, 10, 1115769. 10.3389/fenrg.2022.1115769. [Google Scholar] [CrossRef]
23. Nguyen, H. K., Song, J. B., Han, Z. (2015). Distributed demand side management with energy storage in smart grid. IEEE Transactions on Parallel and Distributed Systems, 26, 3346–3357. 10.1109/TPDS.2014.2372781. [Google Scholar] [CrossRef]
24. Mediwaththe, C. P., Blackhall, L. (2021). Network-aware demand-side management framework with a community energy storage system considering voltage constraints. IEEE Transactions on Power Systems, 36, 1229–1238. 10.1109/TPWRS.2020.3015218. [Google Scholar] [CrossRef]
25. Kebede, A. A., Coosemans, T., Messagie, M., Jemal, T., Behabtu, H. A. et al. (2021). Techno-economic analysis of lithium-ion and lead-acid batteries in stationary energy storage application. Journal of Energy Storage, 40, 102748. 10.1016/j.est.2021.102748. [Google Scholar] [CrossRef]
26. Dufo-López, R., Lujano-Rojas, J. M., Bernal-Agustín, J. L. (2014). Comparison of different lead-acid battery lifetime prediction models for use in simulation of stand-alone photovoltaic systems. Applied Energy, 115, 242–253. 10.1016/j.apenergy.2013.11.021. [Google Scholar] [CrossRef]
27. Zou, C. F., Zhang, L., Hu, X. S., Wang, Z., Wik, T. et al. (2018). A review of fractional-order techniques applied to lithium-ion batteries, lead-acid batteries, and supercapacitors. Journal of Power Sources, 390, 286–296. 10.1016/j.jpowsour.2018.04.033. [Google Scholar] [CrossRef]
28. Kabir, M. M., Demirocak, D. E. (2017). Degradation mechanisms in li-ion batteries: A state-of-the-art review. International Journal of Energy Research, 41, 1963–1986. 10.1002/er.3762. [Google Scholar] [CrossRef]
29. Shahjalal, M., Roy, P. K., Shams, T., Fly, A., Chowdhury, J. I. et al. (2022). A review on second-life of li-ion batteries: Prospects, challenges, and issues. Energy, 241, 122881. 10.1016/j.energy.2021.122881. [Google Scholar] [CrossRef]
30. Liu, Y. Y., Shi, H. D., Wu, Z. S. (2023). Recent status, key strategies and challenging perspectives of fast-charging graphite anodes for lithium-ion batteries. Energy & Environmental Science, 16, 4834–4871. 10.1039/D3EE02213G. [Google Scholar] [CrossRef]
31. Eng, A. Y. S., Kumar, V., Zhang, Y. W., Luo, J., Wang, W. et al. (2021). Room-temperature sodium-sulfur batteries and beyond: Realizing practical high energy systems through anode, cathode, and electrolyte engineering. Advanced Energy Materials, 11, 2003493. 10.1002/aenm.202003493. [Google Scholar] [CrossRef]
32. Zhang, B. W., Sheng, T., Liu, Y. D., Wang, Y. X., Zhang, L. et al. (2018). Atomic cobalt as an efficient electrocatalyst in sulfur cathodes for superior room-temperature sodium-sulfur batteries. Nature Communications, 9, 4082. 10.1038/s41467-018-06144-x [Google Scholar] [PubMed] [CrossRef]
33. Zhang, B. W., Sheng, T., Wang, Y. X., Chou, S., Davey, K. et al. (2019). Long-life room-temperature sodium-sulfur batteries by transition-metal-nanocluster-sulfur interactions. Angewandte Chemie-International Edition, 58, 1484–1488. 10.1002/anie.201811080 [Google Scholar] [PubMed] [CrossRef]
34. Huang, S. P., Lu, Y. J. (2022). Numerical parametric investigation of nonaqueous vanadium redox flow batteries. Batteries, 8, 75. 10.3390/batteries8080075. [Google Scholar] [CrossRef]
35. Fang, J. Y., Tang, Y., Li, H. C., Li, X. (2018). A battery/ultracapacitor hybrid energy storage system for implementing the power management of virtual synchronous generators. IEEE Transactions on Power Electronics, 33(4), 2820–2824. 10.1109/TPEL.2017.2759256. [Google Scholar] [CrossRef]
36. Shen, J. Y., Dusmez, S., Khaligh, A. (2014). Optimization of sizing and battery cycle life in battery/ultracapacitor hybrid energy storage systems for electric vehicle applications. IEEE Transactions on Industrial Informatics, 10(4), 2112–2121. 10.1109/TII.2014.2334233. [Google Scholar] [CrossRef]
37. Chen, X. Y., Xie, Q., Bian, X., Shen, B. Y. (2022). Energy-saving superconducting magnetic energy storage (SMES) based interline DC dynamic voltage restorer. CSEE Journal of Power and Energy Systems, 8(1), 238–248. 10.17775/CSEEJPES.2020.05440. [Google Scholar] [CrossRef]
38. Ma, T., Yang, H. X., Lu, L. (2014). Feasibility study and economic analysis of pumped hydro storage and battery storage for a renewable energy powered island. Energy Conversion and Management, 79, 387–397. 10.1016/j.enconman.2013.12.047. [Google Scholar] [CrossRef]
39. Ma, T., Yang, H. X., Lu, L., Peng, J. (2014). Technical feasibility study on a standalone hybrid solar-wind system with pumped hydro storage for a remote island in Hong Kong. Renewable Energy, 69, 7–15. 10.1016/j.renene.2014.03.028. [Google Scholar] [CrossRef]
40. Razmi, A. R., Soltani, M., Ardehali, A., Gharali, K., Dusseault, M. B. et al. (2021). Design, thermodynamic, and wind assessments of a compressed air energy storage (CAES) integrated with two adjacent wind farms: A case study at Abhar and Kahak sites, Iran. Energy, 221, 119902. 10.1016/j.energy.2021.119902. [Google Scholar] [CrossRef]
41. Wang, W., Yuan, B. Q., Sun, Q., Wennersten, R. (2022). Application of energy storage in integrated energy systems–A solution to fluctuation and uncertainty of renewable energy. Journal of Energy Storage, 52, 104812. 10.1016/j.est.2022.104812. [Google Scholar] [CrossRef]
42. Xu, Y. H., Zhang, H. G., Yang, F. B., Tong, L., Yang, Y. et al. (2021). Experimental study on small power generation energy storage device based on pneumatic motor and compressed air. Energy Conversion and Management, 234, 113949. 10.1016/j.enconman.2021.113949. [Google Scholar] [CrossRef]
43. Lombardi, P., Schwabe, F. (2017). Sharing economy as a new business model for energy storage systems. Applied Energy, 188, 485–496. 10.1016/j.apenergy.2016.12.016. [Google Scholar] [CrossRef]
44. Lu, Y., Xiang, X., Huang, Y., Yu, B., Weng, L. et al. (2023). Deep reinforcement learning based optimal scheduling of active distribution system considering distributed generation, energy storage and flexible load. Energy, 271, 127087. 10.1016/j.energy.2023.127087. [Google Scholar] [CrossRef]
45. Muhyaddin, R., Yusuf, A., Khaled, S., Dadfar, S., Khaki, M. (2023). Optimal operation and stochastic scheduling of renewable energy of a microgrid with optimal sizing of battery energy storage considering cost reduction. Journal of Energy Storage, 59, 106475. 10.1016/j.est.2022.106475. [Google Scholar] [CrossRef]
46. Zheng, S., Huang, G., Lai, A. C. (2021). Techno-economic performance analysis of synergistic energy sharing strategies for grid-connected prosumers with distributed battery storages. Renewable Energy, 178, 1261–1278. 10.1016/j.renene.2021.06.100. [Google Scholar] [CrossRef]
47. Dai, R., Esmaeilbeigi, R., Charkhgard, H. (2021). The utilization of shared energy storage in energy systems: A Comprehensive review. IEEE Transactions on Smart Grid, 12(4), 3163–3174. 10.1109/TSG.2021.3061619. [Google Scholar] [CrossRef]
48. Liu, Y., He, Q., Shi, X., Zhang, Q., An, X. (2023). Energy storage in China: Development progress and business model. Journal of Energy Storage, 72, 108240. 10.1016/j.est.2023.108240. [Google Scholar] [CrossRef]
49. Lu, Y., Guo, Z., Gu, Y., Xu, M., Liu, T. (2023). Analysis of new energy storage policies and business models in China and abroad. Energy Storage Science and Technology, 12(9), 3019. 10.19799/j.cnki.2095-4239.2023.0276. [Google Scholar] [CrossRef]
50. Zhang, C., Ding, N., Yin, F., Sha, Q., Zhang, J. et al. (2022). Overview of new energy storage application scenarios and business models. Distributed Energy Resources, 7(1), 54–62. [Google Scholar]
51. Mongird, K., Viswanathan, V., Balducci, P., Alam, J., Fotedar, V. et al. (2020). An evaluation of energy storage cost and performance characteristics. Energies, 13(13), 3307. 10.3390/en13133307. [Google Scholar] [CrossRef]
52. Zakeri, B., Syri, S. (2015). Electrical energy storage systems: A comparative life cycle cost analysis. Renewable and Sustainable Energy Reviews, 42, 569–596. 10.1016/j.rser.2014.10.011. [Google Scholar] [CrossRef]
53. Padmanabhan, N., Ahmed, M., Bhattacharya, K. (2019). Battery energy storage systems in energy and reserve markets. IEEE Transactions on Power Systems, 35(1), 215–226. 10.1109/TPWRS.2019.2936131. [Google Scholar] [CrossRef]
54. Mongird, K., Viswanathan, V., Balducci, P., Alam, J., Vanshika, F. et al. (2020). An evaluation of energy storage cost and performance characteristics. Energies, 13(13), 3307. 10.3390/en13133307. [Google Scholar] [CrossRef]
55. Ketter, W., Collins, J., Reddy, P. P., Flath, C., Weerdt, M. (2011). The Power Trading Agent Competition (No. ERS-2011-027-LIS). ERIM Report Series Research in Management. http://hdl.handle.net/1765/30683 [Google Scholar]
56. Shang, J. C., Bai, H., Yang, M., Wang, T., Li, Q. et al. (2020). Grid-side energy storage system day-ahead bidding strategy based on two-level decision in spot market. 2020 IEEE 3rd Student Conference on Electrical Machines and Systems (SCEMS), pp. 668–673. Jinan, China. [Google Scholar]
57. You, D., Zhang, G., Song, S., Liu, H., Cai, Y. et al. (2023). Analysis on participation strategy of independent energy storage station in electricity spot market. 2023 8th Asia Conference on Power and Electrical Engineering (ACPEE), pp. 1004–1008. Tianjin, China. [Google Scholar]
58. Zhang, S., Yang, Y., Chang, X., Zhao, J., Fan, R. et al. (2019). Calculation and analysis of energy storage demand in shanxi power grid. In: 2019 IEEE Innovative Smart Grid Technologies-Asia (ISGT Asia), pp. 2510–2515. [Google Scholar]
59. Zhang, C., Yan, W. (2019). Spot market mechanism design for the electricity market in china considering the impact of a contract market. Energies, 12(6), 1064. 10.3390/en12061064. [Google Scholar] [CrossRef]
60. Wang, Q., Zhang, C., Ding, Y., Xydis, G., Wang, J. et al. (2015). Review of real-time electricity markets for integrating distributed energy resources and demand response. Applied Energy, 138, 695–706. 10.1016/j.apenergy.2014.10.048. [Google Scholar] [CrossRef]
61. Kazemi, M., Zareipour, H., Amjady, N., Rosehart, W. D., Ehsan, M. (2017). Operation scheduling of battery storage systems in joint energy and ancillary services markets. IEEE Transactions on Sustainable Energy, 8(4), 1726–1735. 10.1109/TSTE.2017.2706563. [Google Scholar] [CrossRef]
62. Moreno, R., Moreira, R., Strbac, G. (2015). A MILP model for optimizing multi-service portfolios of distributed energy storage. Applied Energy, 137, 554–566. 10.1016/j.apenergy.2014.08.080. [Google Scholar] [CrossRef]
63. Akhavan-Hejazi, H., Mohsenian-Rad, H. (2014). Optimal operation of independent storage systems in energy and reserve markets with high wind penetration. IEEE Transactions on Smart Grid, 5(2), 1088–1097. 10.1109/TSG.2013.2273800. [Google Scholar] [CrossRef]
64. Kalathil, D., Wu, C., Poolla, K., Varaiya, P. (2019). The sharing economy for the electricity storage. IEEE Transactions on Smart Grid, 10(1), 556–567. 10.1109/TSG.2017.2748519. [Google Scholar] [CrossRef]
65. Li, X., Wang, W., Wang, H. (2021). A novel bi-level robust game model to optimize a regionally integrated energy system with large-scale centralized renewable-energy sources in Western China. Energy, 228, 120513.10.1016/j.energy.2021.120513. [Google Scholar] [CrossRef]
66. Shi, J., Lee, W. J., Liu, X. (2017). Generation scheduling optimization of wind-energy storage system based on wind power output fluctuation features. IEEE Transactions on Industry Applications, 54(1), 10–17. 10.1109/TIA.2017.2754978. [Google Scholar] [CrossRef]
67. Roy, P., Liao, Y., He, J. (2023). Economic dispatch for grid-connected wind power with battery-supercapacitor hybrid energy storage system. IEEE Transactions on Industry Applications, 59(1), 1118–1128. 10.1109/TIA.2022.3203663. [Google Scholar] [CrossRef]
68. Xie, Y., Lee, Y., Chang, X., Yin, X., Zheng, H. (2022). Research on the transaction mode and mechanism of grid-side shared energy storage market based on blockchain. Energy Report, 8(2), 224–229. 10.1016/j.egyr.2021.11.044. [Google Scholar] [CrossRef]
69. Zhang, Y., Su, Y., Wang, Z., Liu, F., Li, C. (2021). Cycle-life-aware optimal sizing of grid-side battery energy storage. IEEE Access, 9, 20179–20190. 10.1109/ACCESS.2021.3054860. [Google Scholar] [CrossRef]
70. Bence, S., Dániel, P. D. (2023). Local electricity market design utilizing network state dependent dynamic network usage tariff. IEEE Access, 11, 19247–19258. 10.1109/ACCESS.2023.3249113. [Google Scholar] [CrossRef]
71. Sun, L., Qiu, J., Han, X., Yin, X., Dong, Z. Y. (2020). Capacity and energy sharing platform with hybrid energy storage system: An example of hospitality industry. Applied Energy, 280(4), 115897. 10.1016/j.apenergy.2020.115897. [Google Scholar] [CrossRef]
72. Bukhsh, R., Javaid, N., Abbasi, R. A., Fatima, A., Akbar, M. et al. (2019). An efficient fog as a power economy sharing service. IEEE Access, 7, 185012–185027. 10.1109/ACCESS.2019.2924533. [Google Scholar] [CrossRef]
73. Wang, Z., Gu, C., Li, F. (2018). Flexible operation of shared energy storage at households to facilitate PV penetration. Renewable Energy, 116, 438–446. 10.1016/j.renene.2017.10.005. [Google Scholar] [CrossRef]
74. Yan, N., Zhang, B., Li, W., Ma, S. (2019). Hybrid Energy storage capacity allocation method for active distribution network considering demand side response. IEEE Transactions on Applied Superconductivity, 29(2), 1–4. 10.1109/TASC.2018.2889860. [Google Scholar] [CrossRef]
75. Li, M., Tian, Y., Zhang, H., Zhang, N. (2023). The source-load-storage coordination and optimal dispatch from the high proportion of distributed photovoltaic connected to power grids. Journal of Engineering Research, in press. 10.1016/j.jer.2023.10.042. [Google Scholar] [CrossRef]
76. Ho, W. S., Macchietto, S., Lim, J. S., Hashim, H., Muis, Z. A. et al. (2016). Optimal scheduling of energy storage for renewable energy distributed energy generation system. Renewable and Sustainable Energy Reviews, 58, 1100–1107. 10.1016/j.rser.2015.12.097. [Google Scholar] [CrossRef]
77. Sun, B., Jing, R., Zeng, Y., Li, Y., Chen, J. et al. (2023). Distributed optimal dispatching method for intelligent distribution network considering effective interaction of source-network-load-storage flexible resources. Energy Reports, 9, 148–162. 10.1016/j.egyr.2022.11.178. [Google Scholar] [CrossRef]
78. Ranamuka, D., Muttaqi, K. M., Sutanto, D. (2020). Flexible AC power flow control in distribution systems by coordinated control of distributed solar-PV and battery energy storage units. IEEE Transactions on Sustainable Energy, 11(4), 2054–2062. 10.1109/tste.2019.2935479. [Google Scholar] [CrossRef]
79. Guo, T., Guo, Q., Huang, L., Guo, H., Lu, Y. et al. (2023). Microgrid source-network-load-storage master-slave game optimization method considering the energy storage overcharge/overdischarge risk. Energy, 282, 128897. 10.1016/j.energy.2023.128897. [Google Scholar] [CrossRef]
80. Wang, C., Zhang, X., Xiong, H., Guo, C. (2023). Distributed shared energy storage scheduling based on optimal operating interval in generation-side. Sustainable Energy, Grids and Networks, 34, 101026. 10.1016/j.segan.2023.101026. [Google Scholar] [CrossRef]
81. Ding, Y., Xu, Q., Huang, Y. (2020). Optimal sizing of user-side energy storage considering demand management and scheduling cycle. Electric Power Systems Research, 184, 106284. 10.1016/j.epsr.2020.106284. [Google Scholar] [CrossRef]
82. Shen, W., Zeng, B., Zeng, M. (2023). Multi-timescale rolling optimization dispatch method for integrated energy system with hybrid energy storage system. Energy, 283, 129006. 10.1016/j.energy.2023.129006. [Google Scholar] [CrossRef]
83. Guo, M., Zheng, J., Mei, F., Sha, H., Gao, A. et al. (2023). Double-layer AGC frequency regulation control method considering operating economic cost and energy storage SOC consistency. International Journal of Electrical Power & Energy Systems, 145, 108704. 10.1016/j.ijepes.2022.108704. [Google Scholar] [CrossRef]
84. Li, J., Liang, Z., Xu, S. (2022). Research on modeling and grid connection stability of large-scale cluster energy storage power station based on digital mirroring. Energy Reports, 8, 584–596. 10.1016/j.egyr.2022.02.234. [Google Scholar] [CrossRef]
85. Xia, Y., Xu, Q., Chen, L., Du, P. (2022). The flexible roles of distributed energy storages in peer-to-peer transactive energy market: A state-of-the-art review. Applied Energy, 327, 120085. 10.1016/j.apenergy.2022.120085. [Google Scholar] [CrossRef]
86. Xiao, J., He, G., Fan, S., Li, Z. (2022). Substitute energy price market mechanism for renewable energy power system with generalized energy storage. Substitute energy price market mechanism for renewable energy power system with generalized energy storage. Applied Energy, 328, 120219. 10.1016/j.apenergy.2022.120219. [Google Scholar] [CrossRef]
87. Khojasteh, M., Faria, P., Vale, Z. (2022). Scheduling of battery energy storages in the joint energy and reserve markets based on the static frequency of power system. Journal of Energy Storage, 49, 104115. 10.1016/j.est.2022.104115. [Google Scholar] [CrossRef]
88. Khojasteh, M., Faria, P., Vale, Z. (2021). Energy-constrained model for scheduling of battery storage systems in joint energy and ancillary service markets based on the energy throughput concept. International Journal of Electrical Power & Energy Systems, 133, 107213. 10.1016/j.ijepes.2021.107213. [Google Scholar] [CrossRef]
89. Xiao, Y., Wu, W., Wang, X., Qu, Y., Li, J. (2023). Economic potentials of energy storage technologies in electricity markets with renewables. Energy Storage and Saving, 2, 370–391. 10.1016/j.enss.2022.10.004. [Google Scholar] [CrossRef]
90. Ma, B., Zhang, L., Yu, H., Zou, B., Wang, W. et al. (2023). End-cloud collaboration method enables accurate state of health and remaining useful life online estimation in lithium-ion batteries. Journal of Energy Chemistry, 82, 1–17. 10.1016/j.jechem.2023.02.052. [Google Scholar] [CrossRef]
Cite This Article
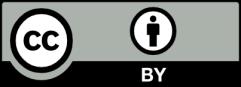
This work is licensed under a Creative Commons Attribution 4.0 International License , which permits unrestricted use, distribution, and reproduction in any medium, provided the original work is properly cited.