Open Access
ARTICLE
Mitigating Carbon Emissions: A Comprehensive Analysis of Transitioning to Hydrogen-Powered Plants in Japan’s Energy Landscape Post-Fukushima
1 Department of Mechanical Engineering Education, Universitas Sebelas Maret, Sukoharjo, 57161, Indonesia
2 International Institute for Carbon-Neutral Reserch (WPI-I2CNER), Kyushu University, Fukuoka, 819-0395, Japan
3 Postgraduate of Mechanical Engineering, Universitas Sebelas Maret, Surakarta, 57126, Indonesia
4 Energy and Society Laboratory, Department of Mechanical Engineering Education, Universitas Sebelas Maret, Sukoharjo, 57161, Indonesia
5 Department of Metallurgical and Material Engineering, Universitas Indonesia, Depok, 16424, Indonesia
* Corresponding Author: Nugroho Agung Pambudi. Email:
(This article belongs to the Special Issue: Innovative Energy Systems Management under the Goals of Carbon Peaking and Carbon Neutrality)
Energy Engineering 2024, 121(5), 1143-1159. https://doi.org/10.32604/ee.2024.047555
Received 09 November 2023; Accepted 28 February 2024; Issue published 30 April 2024
Abstract
One of the impacts of the Fukushima disaster was the shutdown of all nuclear power plants in Japan, reaching zero production in 2015. In response, the country started importing more fossil energy including coal, oil, and natural gas to fill the energy gap. However, this led to a significant increase in carbon emissions, hindering the efforts to reduce its carbon footprint. In the current situation, Japan is actively working to balance its energy requirements with environmental considerations, including the utilization of hydrogen fuel. Therefore, this paper aims to explore the feasibility and implications of using hydrogen power plants as a means to reduce emissions, and this analysis will be conducted using the energy modeling of the MARKAL-TIMES Japan framework. The hydrogen scenario (HS) is assumed with the extensive integration of hydrogen into the power generation sector, supported by a hydrogen import scheme. Additionally, this scenario will be compared with the Business as Usual (BAU) scenario. The results showed that the generation capacities of the BAU and HS scenarios have significantly different primary energy supplies. The BAU scenario is highly dependent on fossil fuels, while the HS scenario integrates hydrogen contribution along with an increase in renewable energy, reaching a peak contribution of 2,160 PJ in 2050. In the HS scenario, the target of reducing CO₂ emissions by 80% is achieved through significant hydrogen penetration. By 2050, the total CO₂ emissions are estimated to be 939 million tons for the BAU scenario and 261 million tons for the Hydrogen scenario. In addition, the contribution of hydrogen to electricity generation is expected to be 153 TWh, smaller than PV and wind power.Keywords
Nomenclature
Carbon dioxide | |
BAU | Business as Usual |
CCS | Carbon Capture and Storage |
ETSAP | Energy Technology Systems Analysis Programmed |
GHG | Greenhouse Gases |
ICE | Internal Combustion Engine |
IEA | International Energy Agency |
IGCC | Integrated Gasification Combined Cycle |
JAEA | Japan Atomic Energy Agency |
LHV | Lower Heating Value |
LPG | Liquefied Petroleum Gas |
MARKAL | MARKet and Allocation |
MCH | Methyl Cyclohexane |
NOx | Nitrogen oxides |
PV | Photovoltaics |
RES | Reference Energy System |
TIMES | Integrated MARKAL-EFOM1 System |
UN | United Nations |
Before the Fukushima disaster, Japan showed exemplary efficiency and reliability in its energy sector. The power generation facilities were managed with exceptional steadiness, ensuring a continuous and stable supply of electricity to meet the increasing demands of both its population and industrial sectors. However, after the disaster, all nuclear power plants were temporarily shut down, reaching zero production in 2015. In response, Japan began importing more coal, oil, and natural gas to fill this energy gap, resulting in a significant increase in carbon emissions, undermining the government’s efforts to reduce the carbon footprint.
Currently, Japan is actively working to balance its energy requirements with environmental concerns. The reintroduction of nuclear power is being carried out gradually, yet the future of the nation’s power generation sector continues to be a subject of intense debate. The government is considering several options: A gradual phase-out of nuclear energy, extending the operational life of existing power plants, or investing in new nuclear power plants to maintain or even increase nuclear power generation capacity. These policies aim to achieve a balance between the growing energy demand, environmental safety, and commitment to reducing greenhouse gas emissions.
In response to global environmental challenges, Japan has established an ambitious target of reducing emissions by 80% by 2050, a goal that aligns with the United Nations (UN) overarching aim to lower greenhouse gas (GHG) emissions worldwide. The UN’s strategy includes a substantial cut in total GHG emissions, targeting a reduction from 1.1 million tons to below 1 million tons in the Business-as-Usual scenario (BAU), under 0.9 million tons in the Innovation scenario, and approaching 0.8 million tons in the Intensification scenario, all within the decade from 2020 to 2030 [1]. This ambitious initiative calls for the expansion of renewable energy sources, such as solar photovoltaics (PV) and wind energy, complemented by the development of more innovative and efficient technologies.
Significant advancements have been made in the renewable energy sector, particularly since 2011, with solar power capacity showing a marked increase. By the end of 2016, the cumulative PV capacity had reached 42,040 MW, which included 153 MW for off-grid systems and 41,879 MW for grid-connected PV systems [2]. Additionally, the wind energy sector, currently at a capacity of 3.2 GW, is anticipated to undergo a substantial increase, reaching 36.2 GW by 2030. However, the expansion of wind energy faces potential limitations due to the constrained grid capacity, the structure of the current electricity market, and operational practices that have limited the integration of wind energy projects into the grid [3].
One of the key strategies identified for reducing emissions in Japan involves the utilization of hydrogen fuel. This approach is backed by the government’s commitment to a hydrogen society program, which emphasizes hydrogen’s significant role in both residential energy consumption and transportation sectors. The concept of a hydrogen-powered society is progressively gaining momentum. This could involve the establishment of hydrogen power plants, where pure or mixed hydrogen fuel is burned within a Brayton cycle power plant. This process generates electricity for power generation. Notably, when the hydrogen used for combustion is sourced from renewable energy sources or is combined with Carbon Capture and Storage (CCS) technology, this method can produce energy with zero emissions. Such an approach holds the potential to make a substantial impact on reducing emissions [4].
In light of these developments, this paper aims to explore the feasibility and implications of constructing hydrogen power plants to reduce emissions. This will be analyzed using the MARKAL-TIMES Japan framework. The hydrogen scenario envisions a widespread integration of hydrogen into the power generation sector, supported by a hydrogen import scheme. Additionally, this scenario will be evaluated against the Business as Usual (BAU) scenario as shown in Fig. 1. This comparative analysis is crucial to understand the variations in emissions levels between these two approaches and to gauge the effectiveness of hydrogen power plants in achieving Japan’s emission reduction goals.
Figure 1: Emission reduction scenarios in Japan [1]
Hydrogen has the potential to offer cost-effective, financially viable, socially beneficial, and energetically efficient solutions to address issues related to the continuously increasing global energy demand, including global warming [5]. Furthermore, it is a fundamental component of the energy transition [6], playing significant roles [7], such as:
• Integration of large-scale renewable energy.
• Easy, reliable, safe, clean, and affordable energy access for all sectors and regions.
• Highly resilient energy systems.
• Integration into multigeneration systems to offer a variety of valuable products.
• Environmentally friendly transportation through fuel cells and hydrogen-powered internal combustion engines.
• Cleaner energy source for industries, residential applications, buildings, etc.
• Environmentally friendly heating, cooling, drying, and power for all energy sectors.
• Eco-friendly industrial raw materials.
Based on this explanation, hydrogen has the potential to provide clean, efficient, reliable, and affordable solutions areas of application with significant social benefits [8]. Moreover, hydrogen can facilitate the widespread adoption and full integration of renewable energy sources, making a significant step towards greening the energy system [9,10].
The low-carbon hydrogen plays a significant role in the energy transition to address some energy challenges, serving as an indirect greenhouse gas with its heating impact often neglected. However, the heating effect of hydrogen released into the atmosphere is short-lived, lasting only a few decades compared to fossil fuels [11].
In light of these developments, this paper aims to explore the feasibility and implications of constructing hydrogen power plants to reduce emissions. This will be analyzed using the MARKAL-TIMES Japan framework. The hydrogen scenario envisions a widespread integration of hydrogen into the power generation sector, supported by a hydrogen import scheme. Additionally, this scenario will be evaluated against the Business as Usual (BAU) scenario. This comparative analysis is crucial to understand the variations in emissions levels between these two approaches and to gauge the effectiveness of hydrogen power plants in achieving Japan’s emission reduction goals.
Several countries worldwide have adopted the use of hydrogen as an alternative energy source. However, in Europe, the potential for green hydrogen faces limitations due to the insufficient expansion of renewable energy-based power plants. Consequently, many European countries are exploring the option of importing green hydrogen to address this challenge. The future viability of hydrogen as an energy source is heavily dependent on strong support from existing policy frameworks. To minimize risks associated with hydrogen infrastructure investment and advocate for the promotion of green hydrogen, Europe needs to formulate a clear and realistic long-term implementation strategy. In this context, it is important to recognize that transitioning to a sustainable energy system incorporates a spectrum of supply technologies, storage options, and demand-side technologies [12].
Some European Union countries have strategically adopted hydrogen in response to the conflict in Ukraine and the collective effort to reduce carbon emissions, aiming for independence from Russian gas supplies. A study conducted by Hanto et al. [13] investigated the impact of hydrogen blending in existing gas pipeline infrastructure on the European energy system up to the year 2050. In this context, hydrogen blending sensitivity factors were integrated into the Global Energy System Model (GENeSYS-MOD). Consequently, the result shows that European hydrogen demand is inelastic and constrained by high costs and specific applications. The incorporation of 100% blending increases hydrogen production in Europe by about 0.17% in 2050 compared to no blending. Additionally, blending significantly influences hydrogen production and trade at the regional level, particularly for countries using existing natural gas pipelines. Despite stable hydrogen production and demand under different sensitivity variations, there are significant differences at the national level. The study model describes Norway’s crucial role as a hydrogen exporter, projecting an increase from 44.0 to 105.9 TWh in 2050. This value is consistent with the elevated blending proportion, leveraging existing gas pipelines. Meanwhile, Turkey and Spain maintain their positions as important exporters with marginal export decreases. France, Germany, and Italy arise as the primary hydrogen importers in 2050, with Germany witnessing increased imports primarily due to rising hydrogen exports from Norway. These results show how blending proportions and geographic proximity shape the dynamics of hydrogen trade in Europe.
Aditiya et al. [14] explored the potential for countries in the Asia-Pacific region to integrate hydrogen energy into their energy portfolios. Furthermore, the study evaluated their domestic energy capacity (DECP index), social components (HPI index), national economic strength (GDPI index), and progress in research and development (RDEI index). Current hydrogen strategies show that Japan, South Korea, Australia, and New Zealand have the potential to lead in establishing the Asia-Pacific Hydrogen Valley. Simultaneous increases in RDEI and HPI values signify significant progress in hydrogen research and development with low social uncertainty toward energy transition scenarios. Singapore and Brunei Darussalam, with the highest GDPI values, have the greatest potential to financially support the Asia-Pacific Hydrogen Valley. Meanwhile, countries with substantial energy capacities, such as Brunei Darussalam and Australia, enjoy a competitive advantage in providing energy resources. Indonesia, Malaysia, Thailand, and Vietnam are identified as the next potential energy suppliers based on their current capacity in energy production per unit. Countries with low operational costs and geographical advantages, including the Philippines, Indonesia, Vietnam, and Thailand, become viable candidates for hydrogen energy infrastructure and transit hubs in the Asia-Pacific Hydrogen Valley supply chain.
3.1 Japanese Power Plant Potential
Until now, Japan’s dependence on fossil energy was significant reaching 87.4% with a renewable energy mix of 57.1% hydro, 32.6% Solar PV, 5.8% wind, 3.8% biomass, and 0.7% geothermal until 2020 [15]. The main purpose of using hydrogen, which is harvested from other energy conversions, is to improve the renewable energy consumption that is commonly used today [16]. Therefore, it drastically reduces the use of fossil fuels and increases energy availability as well as the independence ratio. However, preliminary studies have shown that Japan’s energy independence is still low with a continuous decrease in rate due to an increase in the amount of fossil energy imported. In 2010, the energy independence was 20.3%, which drastically decreased to 11.3% in 2020 [17].
The use of this hydrogen energy provides the potential for a more significant reduction in
The hydrogen society in Japan is planned to be implemented by 2050 when hydrogen will be the main fuel for transportation and residential use. Hydrogen can be obtained from natural gas, heavy oils, coal, naphtha, biomass, and water [22]. Hydrogen is more environmentally friendly and contributes to the worldwide sustainable development targets [16]. In transportation, hydrogen is a promising fuel because of three advantages: GHG emissions reduction, energy security, and reduction of local air pollution [23]. The main challenge facing hydrogen fuel production is its high cost, and because the hydrogen production process requires many resources, the infrastructure is still under development.
In the current situation, fuel cells for personal and industrial use have been released onto the market. Fuel cells for homes have been available since 2009, and fuel cells for cars since 2015. Continued improvements and cost reduction will bring hydrogen into the mainstream. By 2020, the price of hydrogen has the potential to be cost-competitive with that of fuels for hybrid vehicles, and Japan plans to popularize hydrogen in connection with the 2020 Tokyo Olympic Games by using hydrogen fuel cells in buses and other public services [24]. By 2025, fuel cell vehicles will be competitively priced with hybrid cars. In homes, Japan is pursuing subsidy policies towards the installation of 1.4 M Ene-Farm fuel cells by 2020 and 5.3 M by 2030 [25].
The introduction of a hydrogen society in Japan will require several adaptations to the current infrastructure, along with further development of technologies, many of which are already underway. For example, hydrogen fueling stations will be distributed throughout the country. At first, supplies of hydrogen will need to be imported from overseas, but domestic power plants are also planned. Central control centers with advanced computer systems, and cumulative server batteries will also play their part in managing power generation, supply, and demand.
An essential key to achieving a “hydrogen society” is reducing the cost of hydrogen fuel and related technology throughout the entire value chain. This strategy places a significant emphasis on lowering hydrogen production and procurement costs. The main objective is to achieve an 80% cost reduction by around 2050, making hydrogen fuel competitive with natural gas. In 2018, the cost was around 100 yen/Nm3, with a long-term projection to decrease to about 20 yen/Nm3 (from approximately $0.90/Nm3 to $0.17/Nm3). Japan also emphasizes that hydrogen should be carbon-free after 2050 [26].
Fossil fuel production methods often result in high emissions, which require a combination of Carbon Capture and Storage (CCS). In the advanced stages of decarbonization, several components such as biomass, hydrogen, and CCS become the main energy options, contributing approximately 48.2%–70.5% to the total decarbonization potential by 2050 [27].
Hydrogen fuel can also be used in the power generation sector to replace fossil fuels. Within this sector, hydrogen can be utilized in fired and firing technology. The specific energy of the lower heating value (LHV) is approximately 120.21 MJ/kg, which is higher than natural gas or any liquid fuels such as crude oil, gasoline, diesel, naphtha, and propane [28]. This gives the potential for hydrogen to be a highly efficient, abundant, flexible, and clean power source.
Investigation of the pure hydrogen burning and its mixture at the small scale of an internal combustion engine (ICE) has been researched. It is often mixed with gasoline, ethanol, and diesel oil. This combination reduces emissions and improves efficiency. Furthermore, the application of Hydrogen in Brayton cycles can be used in hydrogen-fired and co-combustion power generation. However, the challenges include the cost and Nitrogen oxides (NOx). Research indicated that pure hydrogen burning increases the level of NOx. Details about NOx resultant from gas combustion are presented in Chiesa et al. [29]. The paper illustrates the relationship between the NOx emissions at stoichiometric flame temperature placed in a gas turbine with a pressure of 12–16 bars. It is recommended that the temperature and emission levels for NOx should be below 2600 K with a respective NOx quantity of about 200 ppm. Any increase in hydrogen gas leads to an increase in flame temperature, which results in higher levels of NOx emissions. The recommended temperature in the gas turbine should be approximately 2300 K for pure hydrogen resulting in a NOx concentration of 50 ppm. NOx emissions have been the major challenge facing the production and use of hydrogen fuel in turbine-based generation of electricity.
To derive a potential future Japanese energy system solution by introducing hydrogen into the system, the MARKAL-TIMES code, originally developed by the IEA is employed. MARKAL is an optimization model developed by the Energy Technology Systems Analysis Program (ETSAP) from IEA [30]. Fig. 2 demonstrates the MARKAL-TIMES code generation framework employed in this study.
Figure 2: Markal-Times’ code generation framework [30]
The MARKAL-TIMES method has the advantage of a high level of technical detail and is used to study the entire energy sector at a regional, national, or global scale [31]. Statistically, this method provides advantages for multivariate analysis, so that it can analyze complex variables [32]. It is very popular in modeling carbon emission reduction nowadays. In Europe, especially in France, the TIMES method is used for assessing GHG mitigation and associated costs in the biofuel sector [18]. As in Denmark, it is used for energy system models in the transportation sector [33,34]. In the UK, this method is used to determine the effect of heating technologies on households to achieve the UK’s 2050 greenhouse gas reduction targets [35,36]. As for Asia, especially Japan, this method is used in industrial carbon capture storage (CSS) [37]. Meanwhile, in China, the TIMES method is used in the building sector [38]. A comparative study between China and America was also carried out on carbon reduction in the transportation sector [39]. Furthermore, more extensive research on a global scale also uses this method to reduce carbon in indirect life-cycle carbon dioxide emissions [40] and decrease the rate of increase in global temperature below 2°C [41].
4.1 MARKAL-TIMES Japan Framework
To run the MARKAL-TIMES code generator, a specific energy framework model is required. The Japanese framework was developed by the Japan Atomic Energy Agency (JAEA). The framework was later updated by Kurosawa & Hagiwara, from the Institute of Applied Energy, detailing demand estimates and primary energy supply pricing [42]. Before this framework can be utilized, a reference energy system is required, to describe the initial energy system. Fig. 3 outlines the key factors of the reference energy system.
Figure 3: Reference energy system in the Japanese energy model [42]
4.2 Reference Energy System (RES)
A reference energy system (RES) has been developed as a network representation of how energy is extracted, distributed, and utilized from source to end-use activity in Japan. Each link in the network represents a step for which data such as efficiency, environmental impact, and cost may be specified. The RES can be updated annually and changed to reflect different levels of energy demand, different sources of energy, and the impact of new technologies. Japan’s RES begins with energy resources, which consist of renewables, imported uranium, and imported or domestic fossil fuels. Fuels such as coal and crude oil can be further defined as coking coal, steam coal, anthracite, liquefied petroleum gas (LPG), kerosene, jet fuel, naphtha, gasoline, and heavy fuel. Next in the network are the conversion technologies: Renewable power plants, nuclear power plants, LNG-fired or coal power plants, and oil-fired thermal plants. All energy resources and conversion technologies lead to the same output, electricity, which is transmitted to meet demand in all sectors including commercial, residential, transportation, industrial, and storage.
In this paper, we are focusing on a hydrogen society model. Therefore, the hydrogen flow in the Japanese energy system should be developed to incorporate the RES system as presented in Fig. 4. It illustrates the potential hydrogen flow scenario within the Japanese energy model from production through to end-use. Hydrogen will be produced overseas and imported to Japan. In anticipation of this, Kawasaki Heavy Industries Ltd., and the Iwatani Corporation have partnered with Kobe City to build a liquefied hydrogen import hub [43]. Hydrogen can be transported in liquid form or can be chemically carried using methylcyclohexane (MCH) for example Binding hydrogen with a chemical storage material can increase its density, making it easier to transport in large volumes.
Figure 4: Hydrogen flow in Japan energy model [42]
Other possibilities include liquid, compressed, or slush hydrogen which can be stored in cryogenic tanks, and large quantities can be stored underground in caverns, salt domes, or depleted oil and natural gas fields. The model uses these pure hydrogen pathways with the assumption no
For chemical carriers using MCH, hydrogen is used in natural gas-hydrogen co-combustion plants. It may be initiated in 2030, and there will be no hydrogen import at that time. We presume that the price of hydrogen will be 35 JPY/NM3 [44] which will remain fixed from 2030–2050. Natural gas fuel is supplied in this firing plant. In the BAU scenario, there are no co-combustion plants, only LNG combined cycle plants, using natural gas as a fuel.
Hydrogen is also dispensed from refueling stations for use in hydrogen fuel cell automobiles. The Ministry of Economy, Trade, and Industry (METI) of Japan has targeted 40,000 hydrogen fuel-cell vehicles by 2020, supplied by 160 refueling stations. Currently, more than 135 stations are in operation in Japan until 2020 [45].
As presented in Table 1, two scenarios are investigated: BAU and Hydrogen. Only for the hydrogen scenario is the 80% emission reduction target applied in 2050, reducing emissions to approximately 261 million tons of
The BAU and hydrogen scenario generation capacities are shown in Fig. 5. Each scenario has a significantly different primary energy supply. Since there is no hydrogen import in the BAU scenario, fossil fuel will be the main contributor until 2050. Meanwhile, in the hydrogen scenario, the contribution due to hydrogen begins in 2025 with a small amount. By 2030 the hydrogen contribution will increase to 43 PJ. By 2050 the hydrogen contribution peaks at 2,160 PJ. The fossil fuel contribution in this scenario has been largely replaced by hydrogen fuel and renewable energy. Renewable energy constitutes a large portion of 2050, contributing 8,991 PJ. The total primary energy supply overall is not significantly different between the scenarios. The BAU scenario has a total primary energy supply of 15,643 PJ, while the hydrogen scenario has a slightly lower total supply of 14,903 PJ.
Figure 5: Primary energy supply in BAU (left) and hydrogen scenario (right)
The total primary energy supply for both scenarios is not significantly different but has an impact on the national energy system. This result is consistent with results from a case study in Korea, showing that the hydrogen supply chain scenario will significantly impact the primary energy portfolio in the national energy system [46]. Consequently, policymakers, business actors, and engineers need to carefully consider changes in economic and technological structures to support the implementation of this hydrogen scenario. Sgarbossa et al. [47] proposed that hydrogen supply should be diversified, such as centralized production with renewable energy, including wind and solar energy, or using electricity generated from large-scale hydropower plants. Based on a case study in China addressing challenges in developing the hydrogen supply chain, high construction costs, and technological challenges, Ren et al. [48] suggested active participation in international cooperation.
Fig. 6 shows the emissions produced in each scenario’s energy system within the five sectors of power generation, industry, residential, transportation, and energy conversion. The green bar shows the amount of
Figure 6: Emission reductions before (left) and after the hydrogen scenario is applied (right)
In the BAU scenario, there is no emissions reduction target. The development of the energy system is determined by the cost and the market without any policy driver. Meanwhile, the policy driver behind the hydrogen scenario to reduce 80% of
The implementation of hydrogen use shows that carbon reduction occurs when balanced with efforts to promote environmentally friendly renewable energy and reduce fossil energy usage. The scenario of increased renewable energy use is further explained in Fig. 7. Research by Ren et al. in China found an increased adverse effect in the transportation sector due to the use of hydrogen derived from fossil energy [49]. This occurs because hydrogen from fossil fuels generates greenhouse gas emissions cycles two to three times higher than internal combustion engines. Consequently, Boretti [50] conducted a case study in Australia, focusing on the use of natural gas and coal for hydrogen production. The result suggested the need for Carbon Capture Storage (CCS) to achieve carbon emission reduction targets. Zou et al. [51] also stated that solar, wind, hydropower, nuclear, and hydrogen energy are the main forces of new energy, assisting the power sector in achieving low carbon emissions. However, an Australian study conducted by Milani et al. [52] showed that hydrogen production methods using fossil fuel cracking through steam methane reforming (SMR) or coal gasification integrated with CCS are cheaper than electrolysis methods.
Figure 7: Total electricity generation in TWh in base (left) and hydrogen scenario (right)
5.3 Electricity Generation Supply
As previously described, the sharp decline of power generation in the hydrogen scenario is due to hydrogen power plants, based on imported hydrogen; both in the form of co-fired and co-firing plants. Both types of power plants are assumed to be deployed by 2025, as shown in Fig. 7. With zero emissions, the two types of power plants will replace fossil and nuclear generation. Power generation from hydrogen by 2050 is estimated at 153 TWh. This is less than the contribution from PV and wind power at 492 and 330 TWh, respectively.
Under the BAU scenario, fossil fuel is still the mainstay of the system, predominantly coal. Oil and gas will phase out along with nuclear power. MARKAL-TIMES is more likely to choose coal because these fuels are the cheapest. Coal itself has two technological options by the set-up of the Japanese framework, namely conventional pulverized coal, and integrated gasification combined cycle (IGCC) with low emissions.
The increased use of renewable energy in the hydrogen scenario compared to the Business-As-Usual (BAU) benefits Japan regarding energy independence. Ji et al. [53] stated that Japan is still significantly dependent on OPEC oil imports for a long time, accounting for 80% of total imports. Therefore, political uncertainty and oil trade policies in OPEC countries have a significant impact on the oil import security of the country. Wang et al. [54] found that China and Japan had high trade dependencies with Turkmenistan, Kazakhstan, and Uzbekistan in importing natural gas. Moreover, Japanese trading companies have dependencies on coal-fossil energy sources in Australia and Indonesia [55]. Therefore, Trencher et al. [56] recommended various policy paths and institutional reform measures to promote the deployment of renewable energy sources while reducing dependence on coal.
Apart from reducing dependence on fossil energy in the hydrogen scenario, Japan benefits from the use of locally sourced renewable energy. Cheng et al. [57] identified renewable energy potential in Japan with a result of 4,000 GW of solar PV potential and over 2,000 GW [57]. With these combinations, the power plants represent an annual generation of more than 13,000 TWh, which is 14 times larger than the current generation. Furthermore, Wu et al. [58] found that the total technical bioenergy in Japan can reach 3.43 to 3.78 EJ/year in the 21st century, equivalent to 17.3%–19.1% of the current primary energy supply of the country. Gao et al. [59] discovered that the willingness to pay (WTP) of the Japanese public for renewable energy continuously increased from 2015 to 2030, between approximately 1000 and 2400 JPY/(household·month).
The conclusions and policy implications of this paper can be summarized as follows:
• Hydrogen-based energy is one of the strategies that could be adopted in Japan to reduce emission levels. With an 80% emission reduction target, it is necessary to make radical changes in the future energy system through the adoption of new technologies.
• A comparison of the BAU energy supply scenario and the hydrogen scenario shows significant differences through to 2050. Under a BAU scenario, which does not have an emissions reduction target, GHG reductions occur because of a decreasing demand of 27%. This reduction is insufficient to achieve the goals of the Kyoto Protocol. Under the hydrogen scenario, 80% emissions reduction can be achieved by 2050 with an overall GHG emission level of 261 million tons of
• The power generation sector accounts for a large percentage of emission reductions in the hydrogen scenario, some 93%. Meanwhile, residential and transportation uses of hydrogen account for contributions of approximately 87% and 88% in each sector. While the overall target of 80% across all sectors is met in the hydrogen scenario, the industrial sector only reduces intra-sector emissions by 43%.
• The nature of the Japanese energy system heavily influences where the best reductions can be made, and this is a strong indicator for encouraging a majority effort in the power generation, transport, and residential sectors to engender the future hydrogen economy in Japan.
Based on the results, discussions, and conclusions, the research shows that policymakers are required to consider the hydrogen scenario by supporting regulations to achieve the Paris Agreement targets. Several stakeholders including businesses, engineers, and researchers also need to provide support for financing business sectors, infrastructure, and supporting technologies to ensure the optimal implementation of the hydrogen scenario. The continuous increase in WTP of the Japanese public towards renewable energy, as found by Gao et al. [59] is a positive sentiment for supporting the implementation of the hydrogen scenario that should not be ignored.
Acknowledgement: The authors are grateful to Universitas Sebelas Maret, Indonesia, and LPPM for their support of this study. Additionally, the authors extend their appreciation to the Ministry of Education, Culture, Research, and Technology.
Funding Statement: This research received no specific grant.
Author Contributions: The authors confirm contribution to the paper as follows: Study conception and design: Nugroho Agung Pambudi; data collection and validation: Alfan Sarifudin; analysis and interpretation of results: Andrew Chapman; draft manuscript preparation: Desita Kamila Ulfa, Iksan Riva Nanda. All authors reviewed the results and approved the final version of the manuscript.
Availability of Data and Materials: The data that support the findings of this study are available from the corresponding author, NAP, upon reasonable request.
Conflicts of Interest: The authors declare that they have no conflicts of interest to report regarding the present study.
References
1. United Nations Secretariat, United Nations Secretariat Climate Action Plan 2020–2030. Switzerland: UN Geneva, 2019. Accessed: Jan. 01, 2024. [Online]. Available: https://www.un.org/management/sites/www.un.org.management/files/united-nations-secretariat-climate-action-plan.pdf [Google Scholar]
2. L. Fang, H. H. Xu, and S. C. Wang, “National survey report of PV power applications in China 2014,” Accessed: Jan. 01, 2024, 2014. [Online]. Available: https://iea-pvps.org/national_survey/national-survey-report-of-pv-power-applications-in-china-2014/ [Google Scholar]
3. E. Mizuno, “Overview of wind energy policy and development in Japan,” Renew. Sustain. Energy Rev., vol. 40, pp. 999–1018, 2014. [Google Scholar]
4. A. J. Chapman, T. Fraser, and K. Itaoka, “Hydrogen import pathway comparison framework incorporating cost and social preference: Case studies from Australia to Japan,” Int. J. Energy Res., vol. 41, no. 14, pp. 2374–2391, 2017. [Google Scholar]
5. S. Dutta, “A review on production, storage of hydrogen and its utilization as an energy resource,” J. Ind. Eng. Chem., vol. 20, no. 4, pp. 1148–1156, 2014. doi: 10.1016/j.jiec.2013.07.037. [Google Scholar] [CrossRef]
6. F. Zhang, P. Zhao, M. Niu, and J. Maddy, “The survey of key technologies in hydrogen energy storage,” Int. J. Hydrogen. Energy, vol. 41, no. 33, pp. 14535–14552, 2016. doi: 10.1016/j.ijhydene.2016.05.293. [Google Scholar] [CrossRef]
7. C. Acar and I. Dincer, “Review and evaluation of hydrogen production options for better environment,” J. Clean. Prod., vol. 218, pp. 835–849, 2019. [Google Scholar]
8. Y. Kalinci, A. Hepbasli, and I. Dincer, “Techno-economic analysis of a stand-alone hybrid renewable energy system with hydrogen production and storage options,” Int. J. Hydrogen. Energy, vol. 40, no. 24, pp. 7652–7664, 2015. doi: 10.1016/j.ijhydene.2014.10.147. [Google Scholar] [CrossRef]
9. G. Cipriani et al., “Perspective on hydrogen energy carrier and its automotive applications,” Int. J. Hydrogen. Energy., vol. 39, no. 16, pp. 8482–8494, 2014. doi: 10.1016/j.ijhydene.2014.03.174. [Google Scholar] [CrossRef]
10. A. K. Singh, S. Singh, and A. Kumar, “Hydrogen energy future with formic acid: A renewable chemical hydrogen storage system,” Catal. Sci. Technol., vol. 6, no. 1, pp. 12–40, 2016. [Google Scholar]
11. I. B. Ocko and S. P. Hamburg, “Climate consequences of hydrogen emissions,” Atmos. Chem. Phys., vol. 22, no. 14, pp. 9349–9368, 2022. [Google Scholar]
12. A. Ajanovic, M. Sayer, and R. Haas, “On the future relevance of green hydrogen in Europe,” Appl. Energy, vol. 358, pp. 122586, 2024. [Google Scholar]
13. J. Hanto et al., “Assessing the implications of hydrogen blending on the european energy system towards 2050,” Adv. Appl. Energy, vol. 13, pp. 100161, 2023. [Google Scholar]
14. H. B. Aditiya and M. Aziz, “Prospect of hydrogen energy in Asia-Pacific: A perspective review on techno-socio-economy nexus,” Int. J. Hydrogen. Energy, vol. 46, no. 71, pp. 35027–35056, 2021. [Google Scholar]
15. T. Wakiyama and A. Kuriyama, “Assessment of renewable energy expansion potential and its implications on reforming Japan’s electricity system,” Energy Policy, vol. 115, pp. 302–316, 2018. doi: 10.1016/j.enpol.2018.01.024. [Google Scholar] [CrossRef]
16. N. A. Pambudi, K. Itaoka, A. Kurosawa, and N. Yamakawa, “Impact of hydrogen fuel for CO2 emission reduction in power generation sector in Japan,” Energy Proced., vol. 105, pp. 3075–3082, 2017. doi: 10.1016/j.egypro.2017.03.642. [Google Scholar] [CrossRef]
17. Agency for Natural Resource and Energy, “Japan’s Energy, 2023,” Accessed: Feb. 18, 2024, 2023. [Online]. Available: https://www.enecho.meti.go.jp/en/category/brochures/pdf/japan_energy_2022.pdf [Google Scholar]
18. P. Hugues, E. Assoumou, and N. Maizi, “Assessing GHG mitigation and associated cost of French biofuel sector: Insights from a TIMES model,” Energy, vol. 113, pp. 288–300, 2016. doi: 10.1016/j.energy.2016.06.146. [Google Scholar] [CrossRef]
19. M. Moinuddin and A. Kuriyama, “Japan 2050 low carbon navigator: Possible application for assessing climate policy impacts,” Energy Strateg. Rev., vol. 26, pp. 100384, 2019. [Google Scholar]
20. P. A. Kharecha and M. Sato, “Implications of energy and CO2 emission changes in Japan and Germany after the Fukushima accident,” Energy Policy, vol. 132, pp. 647–653, 2019. [Google Scholar]
21. C. Oltra, R. Sala, S. Germán, and S. López-Asensio, “Trust perceptions among residents surrounding nuclear power plants: A descriptive and explanatory study,” Prog. Nucl. Energy, vol. 113, pp. 1–6, 2019. doi: 10.1016/j.pnucene.2018.12.012. [Google Scholar] [CrossRef]
22. H. Balat and E. Kirtay, “Hydrogen from biomass-present scenario and future prospects,” Int. J. Hydrogen. Energy, vol. 35, no. 14, pp. 7416–7426, 2010. doi: 10.1016/j.ijhydene.2010.04.137. [Google Scholar] [CrossRef]
23. S. Singh et al., “Hydrogen: A sustainable fuel for future of the transport sector,” Renew. Sustain. Energy Rev., vol. 51, pp. 623–633, 2015. doi: 10.1016/j.rser.2015.06.040. [Google Scholar] [CrossRef]
24. S. Matsuda and H. Kubota, “The feasibility of a hydrogen society,” Glob. J. Res. Eng., vol. 16, no. 3, pp. 23–28, 2016. [Google Scholar]
25. A. Maruta, “Japan’s ENE-FARM programme,” Accessed: Jan. 01, 2024, 2016. [Online]. Available: https://docplayer.net/83759173-Japan-s-ene-farm-programme.html [Google Scholar]
26. M. Nagashima, “Japan’s hydrogen strategy and its economic and geopolitical implications,” Accessed: Jan. 01, 2024, 2018. [Online]. Available: https://www.ifri.org/en/publications/etudes-de-lifri/japans-hydrogen-strategy-and-its-economic-and-geopolitical-implications [Google Scholar]
27. Y. Li et al., “Energy transition roadmap towards net-zero communities: A case study in Japan,” Sustain. Cities Soc., vol. 100, pp. 105045, 2024. doi: 10.1016/j.scs.2023.105045. [Google Scholar] [CrossRef]
28. C. J. Cleveland, “Handbook of energy: v.1: Diagrams, charts, and tables,” Choice Rev. Online, vol. 51, no. 7, pp. 51-3860, 2014. doi: 10.5860/choice.51-3860. [Google Scholar] [CrossRef]
29. P. Chiesa, G. Lozza, and L. Mazzocchi, “Using hydrogen as gas turbine fuel,” J. Eng. Gas Turbine. Power, vol. 127, no. 1, pp. 73–80, 2005. doi: 10.1115/1.1787513. [Google Scholar] [CrossRef]
30. The Energy Technology Systems Analysis Program (ETSAPGitHub-etsap-TIMES_TIMES_model at v4.5.3. 2019. Accessed: Jan. 01, 2024. [Online]. Available: https://github.com/etsap-TIMES/TIMES_model/tree/v4.5.3 [Google Scholar]
31. A. S. R. Subramanian, T. Gundersen, and T. A. Adams, “Modeling and simulation of energy systems: A review,” Process., vol. 6, no. 12, pp. 238, 2018. doi: 10.3390/PR6120238. [Google Scholar] [CrossRef]
32. International Energy Agency (IEA“Joint studies for new and mitigated,” Accessed: Jan. 01, 2024, 2011. [Online]. Available: https://iea-etsap.org/index.php/applications/other [Google Scholar]
33. J. Tattini, M. Gargiulo, and K. Karlsson, “Reaching carbon neutral transport sector in Denmark–evidence from the incorporation of modal shift into the TIMES energy system modeling framework,” Energy Policy, vol. 113, pp. 571–583, 2018. doi: 10.1016/j.enpol.2017.11.013. [Google Scholar] [CrossRef]
34. J. Tattini et al., “Improving the representation of modal choice into bottom-up optimization energy system models–The MoCho-TIMES model,” Appl. Energy, vol. 212, pp. 265–282, 2018. doi: 10.1016/j.apenergy.2017.12.050. [Google Scholar] [CrossRef]
35. P. H. Li, I. Keppo, and N. Strachan, “Incorporating homeowners’ preferences of heating technologies in the UK TIMES model,” Energy, vol. 148, pp. 716–727, 2018. [Google Scholar]
36. J. M. Cayla and N. Maïzi, “Integrating household behavior and heterogeneity into the TIMES-households model,” Appl. Energy, vol. 139, pp. 56–67, 2015. [Google Scholar]
37. N. A. Pambudi, K. Itaoka, A. Chapman, A. Kurosawa, and E. Kato, “Industrial carbon capture storage (CSS) model using times-Japan framework,” Energy Proced., vol. 142, pp. 2525–2533, 2017. doi: 10.1016/j.egypro.2017.12.193. [Google Scholar] [CrossRef]
38. J. Shi, W. Chen, and X. Yin, “Modelling building’s decarbonization with application of China TIMES model,” Appl. Energy, vol. 162, pp. 1303–1312, 2016. doi: 10.1016/j.apenergy.2015.06.056. [Google Scholar] [CrossRef]
39. H. Zhang, W. Chen, and W. Huang, “TIMES modelling of transport sector in China and USA: Comparisons from a decarbonization perspective,” Appl. Energy, vol. 162, pp. 1505–1514, 2016. doi: 10.1016/j.apenergy.2015.08.124. [Google Scholar] [CrossRef]
40. W. McDowall, B. Solano Rodriguez, A. Usubiaga, and J. Acosta Fernández, “Is the optimal decarbonization pathway influenced by indirect emissions? Incorporating indirect life-cycle carbon dioxide emissions into a European TIMES model,” J. Clean. Prod., vol. 170, pp. 260–268, 2018. doi: 10.1016/j.jclepro.2017.09.132. [Google Scholar] [CrossRef]
41. W. Huang, W. Chen, and G. Anandarajah, “The role of technology diffusion in a decarbonizing world to limit global warming to well below 2°C: An assessment with application of global TIMES model,” Appl. Energy, vol. 208, pp. 291–301, 2017. doi: 10.1016/j.apenergy.2017.10.040. [Google Scholar] [CrossRef]
42. A. Kurosawa and N. Hagiwara, “Long term energy system analysis of Japan after March 11, 2011,” in Third IAEE Asian Conf., Kyoto, Japan, 2012, pp. 20–22. [Google Scholar]
43. L. Maths and S. Eriksson, Report: Hydrogen Technology Market in Japan. 2016. Accessed: Jan. 01, 2024. [Online]. Available: https://www.eu-japan.eu/eubusinessinjapan/library/publication/report-hydrogen-technology-market-japan [Google Scholar]
44. E. Kato and A. Kurosawa, “Evaluation of Japanese energy system toward 2050 with TIMES-Japan–deep decarbonization pathways,” Energy Proced., vol. 158, pp. 4141–4146, 2019. doi: 10.1016/j.egypro.2019.01.818. [Google Scholar] [CrossRef]
45. M. Genovese and P. Fragiacomo, “Hydrogen refueling station: Overview of the technological status and research enhancement,” J. Energy Storage, vol. 61, pp. 106758, 2023. doi: 10.1016/j.est.2023.106758. [Google Scholar] [CrossRef]
46. J. Choi, D. G. Choi, and S. Y. Park, “Analysis of effects of the hydrogen supply chain on the Korean energy system,” Int. J. Hydrogen. Energy, vol. 47, no. 52, pp. 21908–21922, 2022. doi: 10.1016/j.ijhydene.2022.05.033. [Google Scholar] [CrossRef]
47. F. Sgarbossa, S. Arena, O. Tang, and M. Peron, “Renewable hydrogen supply chains: A planning matrix and an agenda for future research,” Int. J. Prod. Econ., vol. 255, pp. 108674, 2023. doi: 10.1016/j.ijpe.2022.108674. [Google Scholar] [CrossRef]
48. X. Ren, L. Dong, D. Xu, and B. Hu, “Challenges towards hydrogen economy in China,” Int. J. Hydrogen. Energy, vol. 45, no. 59, pp. 34326–34345, 2020. doi: 10.1016/j.ijhydene.2020.01.163. [Google Scholar] [CrossRef]
49. L. Ren, S. Zhou, and X. Ou, “Life-cycle energy consumption and greenhouse-gas emissions of hydrogen supply chains for fuel-cell vehicles in China,” Energy, vol. 209, pp. 118482, 2020. doi: 10.1016/j.energy.2020.118482. [Google Scholar] [CrossRef]
50. A. Boretti, “Production of hydrogen for export from wind and solar energy, natural gas, and coal in Australia,” Int. J. Hydrogen. Energy, vol. 45, no. 7, pp. 3899–3904, 2020. doi: 10.1016/j.ijhydene.2019.12.080. [Google Scholar] [CrossRef]
51. C. N. Zuo et al., “The role of new energy in carbon neutral,” Petrol. Explor. Dev., vol. 48, no. 2, pp. 480–491, 2021. [Google Scholar]
52. D. Milani, A. Kiani, and R. McNaughton, “Renewable-powered hydrogen economy from Australia’s perspective,” Int. J. Hydrogen. Energy, vol. 45, no. 46, pp. 24125–24145, 2020. doi: 10.1016/j.ijhydene.2020.06.041. [Google Scholar] [CrossRef]
53. Q. Ji, H. Y. Zhang, and D. Zhang, “The impact of OPEC on East Asian oil import security: A multidimensional analysis,” Energy Policy, vol. 126, pp. 99–107, 2019. doi: 10.1016/j.enpol.2018.11.019. [Google Scholar] [CrossRef]
54. W. Wang, L. W. Fan, and P. Zhou, “Evolution of global fossil fuel trade dependencies,” Energy, vol. 238, pp. 121924, 2022. doi: 10.1016/j.energy.2021.121924. [Google Scholar] [CrossRef]
55. G. Trencher, C. Downie, K. Hasegawa, and J. Asuka, “Divestment trends in Japan’s international coal businesses,” Renew. Sustain. Energy Rev., vol. 124, pp. 109779, 2020. doi: 10.1016/j.rser.2020.109779. [Google Scholar] [CrossRef]
56. G. Trencher, N. Healy, K. Hasegawa, and J. Asuka, “Discursive resistance to phasing out coal-ired electricity: Narratives in Japan’s coal regime,” Energy Policy, vol. 132, pp. 782–796, 2019. doi: 10.1016/j.enpol.2019.06.020. [Google Scholar] [CrossRef]
57. C. Cheng, A. Blakers, M. Stocks, and B. Lu, “100% renewable energy in Japan,” Energy Convers. Manage., vol. 255, pp. 115299, 2022. doi: 10.1016/j.enconman.2022.115299. [Google Scholar] [CrossRef]
58. W. Wu, T. Hasegawa, S. Fujimori, K. Takahashi, and K. Oshiro, “Assessment of bioenergy potential and associated costs in Japan for the 21st century,” Renew. Energy, vol. 162, pp. 308–321, 2020. doi: 10.1016/j.renene.2020.08.015. [Google Scholar] [CrossRef]
59. L. Gao, Y. Hiruta, and S. Ashina, “Promoting renewable energy through willingness to pay for transition to a low carbon society in Japan,” Renew. Energy, vol. 162, pp. 818–830, 2020. doi: 10.1016/j.renene.2020.08.049. [Google Scholar] [CrossRef]
Cite This Article
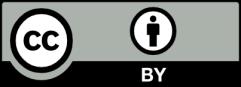
This work is licensed under a Creative Commons Attribution 4.0 International License , which permits unrestricted use, distribution, and reproduction in any medium, provided the original work is properly cited.