Open Access
ARTICLE
Hydroelectric and Hydrogen Storage Systems for Electric Energy Produced from Renewable Energy Sources
1 Department of Engineering, Università della Campania “Luigi Vanvitelli”, Aversa (CE), 81031, Italy
2 Laboratory of Electronics Treatment Information, Mechanic and Energetic, University of Ibn Tofail, Kenitra, 14000, Morocco
* Corresponding Author: Saif Serag. Email:
(This article belongs to the Special Issue: Materials and Energy an Updated Image for 2023)
Energy Engineering 2024, 121(10), 2719-2741. https://doi.org/10.32604/ee.2024.054424
Received 27 May 2024; Accepted 30 July 2024; Issue published 11 September 2024
Abstract
Renewable energy sources are essential for mitigating the greenhouse effect and supplying energy to resource-scarce regions. However, their intermittent nature necessitates efficient storage solutions to enhance system efficiency and manage energy costs. This paper investigates renewable and clean storage systems, specifically examining the storage of electricity generated from renewable sources using hydropower plants and hydrogen, both of which are highly efficient and promising for future energy production and storage. The study utilizes extensive literature data to analyze the impact of various parameters on the cost per kWh of electricity production in hybrid renewable systems incorporating hydropower and hydrogen storage plants. Results indicate that these hybrid systems can store electricity efficiently and cost-effectively, with production costs ranging from 0.126 to 0.3 $/kWh for renewable-hydropower systems and 0.118 to 0.42 $/kWh for renewable-hydrogen systems, with expected cost reductions over the next decade due to technological advancements and increased market adoption. The novelty of this study lies in its comprehensive comparison of hybrid renewable systems integrating hydropower and hydrogen storage, providing detailed cost analysis and future projections. It identifies key parameters influencing the cost and efficiency of these systems, offering insights into optimizing storage solutions for renewable energy. Moreover, this research underscores the potential of hybrid systems to reduce dependency on fossil fuels, particularly during peak demand periods, and emphasizes the importance of seasonal and geographic considerations in selecting energy sources. The study highlights the importance of policy support and investment in hybrid renewable systems and calls for further research into optimizing these systems for different seasonal and geographic conditions. Overall, the integration of renewable energy sources with hydropower and hydrogen storage offers a promising pathway to a sustainable, economical, and resilient energy future.Graphic Abstract
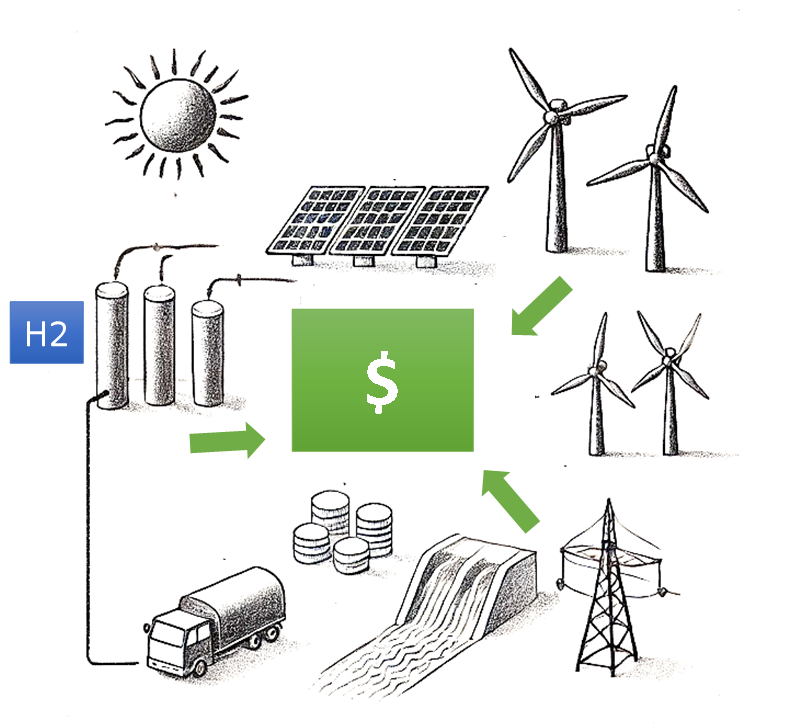
Keywords
Nomenclature
C0e | Capital Costs for LCOE, Capex |
C0s | Capital Costs for LCOS, Capex |
CRF | Capital Recovery Factor |
Edis | Electricity Discharged |
Et | Electricity Production |
Ft | Fuel Expenditure |
HHV | Higher Heating Value |
i | Discount Rate |
Levelized Cost of Energy | |
LCOS | Levelized Cost of Storage |
LHV | Low Heating Value |
Mte | Operational Costs for LCOE, Opex |
Mts | Operational Costs for LCOS, Opex |
O&M | Operation and Maintenance |
N | Lifetime of the Project |
PEM | Proton Exchange Membrane |
RES | Renewable Energy Sources |
Rt | Charging Cost |
t | Time |
World is facing several dramatic challenges. Among them, two can be considered significant: first is securing energy supplies to meet different regions’ needs, especially with the consistent increase in demand, the large increase in population and the increase in services dependent on energy supplies, such as health services, means of transportation, communications, entertainment, etc. The second is to limit the use of fossil energy sources causing climate change, as several problems have emerged as a result of energy production using fossil fuels, like air, soil and water pollution, global warming, ozone hole expansion, and other problems that have dire consequences for Earth and future generations [1]. The best solutions to these two challenges are the production of energy from renewable sources such as wind, solar, tidal energy sources, biomass and other sustainable, renewable, and natural sources that are clean and environmentally friendly. Storage systems represent the most important devices for renewable energy sources (RES for short) to ensure the continuity of energy production, especially since they are unstable and change with time and seasons. Storage systems also guarantee solving the problem of instability and variation in energy production and ensure improving the quality of energy production and supply, integrating renewable resources and reducing their negative impact, which has direct consequences on the environment and the transition to a carbon-free future [2].
Hydro pump systems accounted for the largest share of global energy storage, comprising approximately 92% in 2020. This dominance highlights the established nature and reliability of pumped hydro storage in energy management. Following hydro pump systems, electrochemical storage systems constituted 5.9% of the global energy storage. Within this category, lithium-ion batteries were the most prevalent, representing 90% of electrochemical storage systems. The widespread use of lithium-ion batteries underscores their efficiency and declining costs, making them a popular choice for energy storage solutions. Other storage technologies, such as flywheels, compressed air energy storage (CAES), and thermal storage, collectively made up the smallest percentages of the global energy storage landscape, indicating the relatively nascent stage and lower adoption rates of these alternatives [3].
The economic study of energy production and storage projects represents a crucial aspect for planning and deploying such systems, as it prompts investors and decision makers to take decisions regarding investments, deployment and distribution, which in turn limits carbon emissions, climate change mitigation and air pollution, in addition to giving a future vision for the decrease in the costs of renewable energy projects and their storage.
Unfortunately, few data are available on the economic cost of storage projects, which makes it difficult to predict future cost decrease. Therefore, a realistic study of the costs of energy production and storage must be given away from exaggerations. This would result either in lower costs because of increased production and storage efficiency or in higher costs, as a result of higher raw materials costs, capital costs, and higher demand in the future [4].
The cost of energy production projects can be determined through the calculation of the levelized cost of energy (LCOE) and the levelized cost of storage (LCOS). This process involves a comprehensive analysis of the total costs associated with the project. These costs include capital expenditures (Capex), which cover the initial investment required to build the project, and operating and maintenance expenditures (Opex), which are the ongoing costs needed to keep the project running efficiently. Additionally, other parameters that influence the project’s overall cost, such as financing, insurance, and regulatory compliance, must be considered. For calculate the LCOE and LCOS, these total costs are divided by the total amount of energy produced and stored over the entire operational lifespan of the project. This division accounts for the efficiency of the energy production and storage systems and the actual quantity of energy generated and stored. By taking into account both the initial and ongoing costs, as well as the energy output, the LCOE and LCOS provide a standardized metric that allows for the comparison of the economic viability of different energy projects. This metric is crucial for investors and policymakers as it helps to identify the most cost-effective energy solutions, ensuring that resources are allocated efficiently to achieve sustainable energy goals [5,6].
The technological development that took place in the last decade, represented by an increase in the efficiency of generators and a decrease in Capex and Opex costs, led to a decrease in the costs of various energy projects. During the last years, renewable energy projects witnessed remarkable changes in the produced energy cost, LCOE. Fig. 1 shows the difference in LCOE between 2010 and 2020 for some renewable energy sources, considering min, max, and average values.
Figure 1: Comparison of global weighted average LCOE for some renewable energy sources in 2010 and 2020. Adapted from references [7–10]
Over the past decade, the average cost of onshore wind projects has decreased significantly, dropping from $0.086 to $0.063 per kWh. This represents a cost reduction of approximately 27%, highlighting the advancements and economies of scale achieved in this sector. Offshore wind projects experienced an even greater decrease in costs, with prices falling from $0.16 to $0.11 per kWh, which is a substantial reduction of 32%. These reductions underscore the growing efficiency and competitiveness of wind energy in the global market. Solar energy has also seen remarkable cost declines. The average costs of photovoltaic (PV) projects have decreased dramatically, from $0.38 to $0.068 per kWh, which accounts for a reduction of more than 82%. This significant drop reflects improvements in solar panel technology, manufacturing processes, and deployment strategies. Concentrated Solar Power (CSP) projects, which include technologies such as power towers and Stirling dishes, have also benefited from technological advancements and economies of scale. The average cost for CSP projects has fallen from $0.35 to $0.18 per kWh, a reduction of around 50%. Hydropower plants, which are among the simplest and most cost-effective energy production methods, have witnessed a slight increase in their levelized cost of energy (LCOE). The average cost rose from $0.037 to $0.047 per kWh, an increase of approximately 20%. Despite this increase, hydropower remains the most cost-efficient energy production method with the lowest LCOE among all renewable energy sources. Tidal energy costs have decreased slightly, from $0.018 to $0.017 per kWh, which is a modest reduction of 5.6%. This indicates a gradual improvement in the efficiency and viability of tidal energy technologies. Biomass plants have also seen a decrease in costs, with the average cost dropping from $0.078 to $0.066 per kWh, marking a reduction of around 16%. These reductions in biomass costs reflect advancements in biomass conversion technologies and better resource management practices. Overall, these cost trends demonstrate significant progress in making renewable energy sources more affordable and competitive with traditional fossil fuels. The continuous decline in the cost of wind, solar, and biomass energy highlights the potential for these renewable sources to play a major role in the global transition to a sustainable and low-carbon energy future [7–10].
Energy storage systems are essential for the stability and efficiency of renewable energy sources, but many have significant environmental drawbacks. The extraction of materials like lithium and cobalt, vital for most battery technologies, often leads to environmental degradation and human rights issues. Mining activities can result in habitat destruction, soil and water contamination, and high carbon emissions. Therefore, developing environmentally friendly energy storage solutions is becoming increasingly important. The cost of storing electricity is expected to decrease significantly, especially for lithium-ion batteries. These batteries are favored for their efficiency, energy density, and declining costs. However, for other storage systems to remain competitive, they must achieve similar performance improvements and cost reductions [11].
Hydropower remains a valuable solution for long-term and large-scale energy storage. Pumped hydro storage, in particular, can store large amounts of energy and release it as needed, supporting the electrical grid during high demand or low renewable generation periods. Despite its potential, hydropower faces geographical and environmental constraints [12].
Hydrogen is increasingly being recognized as a vital component for energy storage due to its versatility and environmental benefits. As an energy carrier, hydrogen can store excess energy produced from renewable sources like wind and solar, which are intermittent by nature. This stored energy can then be used to generate electricity when demand is high or supply from renewables is low. Hydrogen storage systems offer long-term storage capabilities, making them an effective solution for balancing the energy grid and enhancing the reliability of renewable energy systems. Additionally, hydrogen can be used in fuel cells to power vehicles and industries, contributing to a reduction in greenhouse gas emissions and supporting the transition to a sustainable, low-carbon energy future [13].
In summary, the diversity of energy storage systems presents both opportunities and challenges. While many current technologies have environmental impacts, ongoing research is crucial to develop more sustainable solutions. As energy storage costs decline, the focus must remain on enhancing environmental sustainability to achieve a truly resilient and sustainable energy future.
In this paper, a comprehensive technical and economic study is presented to evaluate the levelized cost of energy (LCOE) and the levelized cost of storage (LCOS) for energy derived from renewable sources. The focus is on two sustainable and environmentally friendly storage methods: hydropower systems and hydrogen production and storage. The study involves a detailed analysis of the costs associated with each component of these systems. By reviewing the capital expenditures (Capex), operating and maintenance costs (Opex), and other relevant expenses for each system part, the total cost is calculated when these storage systems are integrated with renewable energy sources to form hybrid systems.
The findings demonstrate that producing and storing energy using these systems can be economically viable. The cost analysis shows that these hybrid systems can achieve competitive prices in the global energy markets, making them attractive options for large-scale energy production and storage. Furthermore, the use of hydropower and hydrogen storage not only supports the stability and reliability of renewable energy sources but also contributes to a reduction in greenhouse gas emissions and environmental impact.
The paper underscores the importance of investing in sustainable storage solutions to complement renewable energy production. By proving that these systems can be cost-effective and environmentally friendly, the study provides valuable insights for policymakers, investors, and industry stakeholders looking to transition to a more sustainable and resilient energy future.
To obtain the different costs of the storage systems coupled with the energy renewable systems two main parameters are employed in the investigation, namely, levelized cost of energy (LCOE) and levelized cost of storage (LCOS) [6,12].
where C0e and C0s is the investment expenditures or Capex, Mte and Mts the annual operations and maintenance costs for energy production and storage or Opex, Rt charging cost, Ft the fuel expenditures per year, Et the electricity produced per year, Edis the electricity discharged per year, i the discount rate, and N the operating lifetime of the project.
Capital Recovery Factor (CRF) is one of the important parameters for calculating the LCOE, as it has a direct impact on the investment cost, where calculated to determine annual cash payments series that can be paid monthly, annually or in any other regular period, which is given by Eq. (3) [14].
CRF is actually affected by several factors that directly influence the discount rate such as initial investment size, return rate or profitability, consistency and cash flows, which may lead to a slowdown in the recovery of investment costs. Other factors such as inflation, operating expenses, and taxes also negatively affect the speed of capital recovery, as increased inflation affects the real value of capital, and increased operating expenses and taxes may reduce the cash funds available for capital recovery.
Capacity factor, CF, is considered an important factor that directly affects the amount of energy produced for any project, given by Eq. (4).
where Time is equal to 8760 h/year.
Fig. 2 shows the details of LCOE calculation, showing arrangement and effect of various parameters on total cost calculation and total energy calculation. The investment cost can be obtained by calculating the CRF, according to interest rate and project lifetime, by summing the capital cost to the cost of operation and maintenance and other added costs, and by knowing the amount of energy that can be produced during the lifetime of the investment, LCOE can be calculated, assuming that the energy production is the same each year.
Figure 2: Detailed flow chart for calculating LCOE
Studies indicate an increase in electricity generation by hydropower plant capacity by 2% in 2019 due to the increase in the level of water and rain, in addition to the significant expansion of capacities. Hydropower is produced in 150 countries [15], where pumped storage hydropower totaled 1.5 GW of the new additions in capacity. Most of this was in China (1.2 GW), with Israel also commissioning the 300 MW Mount Gilboa project under an innovative financing model, Major projects completed in 2020 included the 2.1 GW Lauca facility in Angola, the 1.8 GW Jixi pumped storage facility in China and the Ilisu (1.2 GW) and Lower Kaleköy (0.5 GW) projects in Turkey. The single biggest increase in capacity was in China, where Wudongde project put eight of its 12 units online, adding 6.8 GW to the Chinese grid, the remainder is expected to be commissioned in 2021 [16].
Fig. 3 shows the method used to apply the storage system for electricity by hydropower storage, where a part of the electric energy produced from renewable energy sources (wind, solar, tidal, and biomass) is used in off-peak period to operate the pump in order to push the water to a high dam. Providing water in the dam until the period of peak demand for electricity, when the reservoir gate is opened to pass water through turbines, which in turn generates electricity, and the hydropower system is integrated with the network of renewable energy sources.
Figure 3: Mechanism of renewable–hydropower system operation
Dam height and the amount of water flow plays an important role in increasing the production capacity, as the production capacity increases as storage dam height increases, which in turn increases the amount of flow and increases the production capacity. Fig. 4 shows the amount of production capacity that can be applied at different head and flow together with the typical range of operations for the hydraulic turbines that used for energy production [17].
Figure 4: Hydropower capacities according to flow and head. Reprinted from reference [17]
Hydropower storage cost can be calculated in a detailed and unrelated with main energy sources that needs to supply energy to charge the dam’s water, then by combine it with renewable sources as a hybrid system, thus, the total electricity production cost by renewable sources and hydropower as storage system, can be calculated.
Hydropower stations cost changes according to producing companies, capacities, dam heights, area and capacity. By following up on changes in cost over the past years, it was found that hydropower system capital costs ranged between 1415 and 10,000 $/kW [18,19], with an average value of around 5800 $/kW, cost in specific cases can reach up to 34,000 $/kW [20], as in the Westville project with a low head (3.35 m). In fact, there is no significant decrease in prices, but an interesting relationship exists with the production capacity and head. Fig. 5 shows the resulting decreases in average capital cost as a function of the head and the production capacity. It is observed that for small capacities (up to 50 kW) the reduction of the specific Capex with increasing the head is significant for low heads, up to 60 m, then the values remain nearly constant. Whereas for greater capacity plants, larger than 500 kW, the reduction of the specific Capex is obtained for all Head values. Smaller projects have slightly higher investment costs and are expected to have higher average costs as the specific electromechanical costs (per kW) can be very high and affect the total costs.
Figure 5: Hydropower investment specific cost ($/kW) dependence on head (m)
There are other influential costs such as operation and maintenance (O&M), which amount to between 1.6% and 6.5% of Capex, then capacity factor and discount rate also play a key role in attaining the LCOE value. Fig. 6 shows LCOE for different values of the discount rate, equal to 3%, 7%, 10%, and different specific investment cost, 1000, 2000, 3000, and 6000 $/kW [17].
Figure 6: LCOE as a function of the specific investment cost and discount rate for hydropower
O&M includes several services, such measurement and verification services, system uptime and production optimization, reliability-based maintenance, preventive, predictive and reactive maintenance [21]. The Capex details can be listed as follows: the reservoir accounts for 26%–32% of the total expenditure, the tunnel between 5% and 14%, the powerhouse between 30% and 33%, including devices and shafts. As for construction and engineering, 7%–15% and the owner cost 14%–23%, as shown in Table 1.
Table 2 shows details of total cost of the hydropower project, in which the costs are obtained according to the production capacity (Less 1 MW, 1–10 MW, greater than 10 MW), showing the range of investment costs, maintenance and operation costs, lifetime, efficiency and electrical capacity factor.
Hydrogen is a chemical element with symbol H with a gas density of 0.09 g/L at 0°C and 1.013 bar of pressure, which means 14 times lighter than air, and it has a great ability to spread outside the atmosphere [24]; however, it exists in combination with other elements. While hydrogen is not a primary source of energy, it becomes an attractive energy carrier when separated from other elements using alternative energy sources. Hydrogen is a clean energy vector for future energy, especially for energy storage and transmission purposes [25]. The storage capacity of hydrogen energy is excellent because that each kilogram of hydrogen contains approximately 39.4 and 33.6 kWh based on the higher heating value (HHV) and low heating value (LHV), respectively [26]. The main advantages of using hydrogen for power supply include that it is a sustainable, clean, and environmentally friendly source. It can be stored and transported to different places, and combustion product is mostly just water [27].
In this research, the methods of hydrogen production are investigated by using renewable sources of energy, such as wind energy, solar energy, tidal energy, and biomass, by using a Proton Exchange Membrane (PEM) because hydrogen production using this method is purer, and the simplest and cheapest manners to store hydrogen. Of course, hydrogen could be transported from one place to another, and there are different ways to transport hydrogen in its various phases solid, liquid, and gaseous, so different transportation costs are investigated, then will study the cost of generating electricity from hydrogen using fuel cell technology, as in Fig. 7.
Figure 7: Mechanism of renewable hydrogen system operation
4.1 Hydrogen Production by PEM
There are three systems of hydrogen production by electrolysis; alkaline electrolyzer, anion exchange membrane, and proton exchange membrane (PEM), which is the subject of this research. PEM is distinguished from other methods in that the hydrogen produced has the highest purity around 99.9999% and can be applied to medium to small projects between 0.2 and 1200 kW [27–29].
PEM works applying continuous electric current to the electrodes immersed in the cell and water is spilt into oxygen and hydrogen so that the oxygen ions are attracted towards anode while the hydrogen is towards cathode. PEM operates under a temperature between 70°C and 80°C and with an efficiency of 65% to 90% [30,31].
A decrease in the capital cost occurred during the past years as the cost in 2010 was 2900–4400 $/kW, and decreased in 2020 until it reached 900–1600 $/kW, and expect a reduction in the capital cost by 2030, when it would reach 400–700 $/kW, While the percentage of operation and maintenance cost is 1.5%–5% or 15 to 45 $/kW per year, PEM life span ranged 7 to 10 years, or approximately 50,000 to 70,000 h, as shown in Table 3.
4.2 Hydrogen Storage and Transportation
Technology recently has developed significantly to ensure the quality, safety and security of storage and meet the requirements of cost and performance, and it has varied so that hydrogen can be stored in its three phases, solid, liquid and gaseous, but hydrogen storage methods can be divided into two main parts: The first is the physical storage method and includes compressed gas 350–700 bar, coolant, and liquid hydrogen. The second method is a chemical method, the adsorption method, which is the storage of hydrogen on the surfaces of solid materials, and the absorption method in which hydrogen molecules are stored inside the solid materials [32]. Hydrogen transfer depends on several factors, most notably the hydrogen phase (liquid, solid, gaseous), distance travelled and hydrogen amount to be transported. The most important means used to store and transport hydrogen and potential costs of each method will be reviewed separately.
This is the most common method for storing hydrogen in cylindrical steel tanks, for increasing the density of gaseous hydrogen, it is cooled to lower temperatures, in addition require high pressures between 350 and 700 bar which entail density between 35 and 65 kg/m3. These tanks are commercially available, safe, high-performance, and do not require any internal heat exchanger, have also low weight, despite their large size compared to other methods. Tank cost used for Hydrogen Compressed gas storage is between 500 and 600 $/kg [33,34].
Compressed gas storage method is considered more economical than others, as the storage costs in cylinders ranges from 0.21 to 0.6 $/kg, and more than 4.25 $/kg for pipes for one day, but for larger periods which range from 30 days, the costs can be assessed from 1 to 5 $/kg, as for the pipes be more than 7 $/kg [35], while maintenance and operating costs range from 0.1 to 0.5 $/kg, and other costs 0.1 to 0.2 $/kg [3]. In 2020 the capital costs have reduced to around 0.2 $/kg for 30-day storage, while there are no significant differences in other costs.
Underground compressed gas storage technology considers appropriate and best for large quantities if hydrogen needs to be stored for long periods. The capital costs for them are estimated from 2 to 11 $/kg, which is a low value if compared with steel tanks storage method because this method enables hydrogen preservation for several months and with higher efficiency [36,37].
Compressed gas can be transported either by tube trailers or by pipelines or pressurized cylinders. High pressure is applied to the gas between 180 and 500 bar in order to increase the carrying capacity of the tanks or cylinders. Cylinders are a very expensive technology to deal with because their carrying capacity is low. As for tube trailers, which are composed of several steel cylinders, they have a capacity between 36 and 460 kg of hydrogen [38].
Transportation costs by tube trailer vary according to the applied pressure and delivery distance, however, these costs can be reduced by lower capital costs. The costs of a trailer containing between 180 and 460 kg range from 100,000 to 360,000 $. Pipes costs changes according to pipes diameters and quality, where costs range between 2500 and 6000 $/km for small diameters of 5 cm and gradually increase according to diameter and reach 1,200,000 $/km for large diameters that approach 50 cm. Generally, Gaseous Hydrogen transportation costs are between 0.39 and 1.2 $/kg [39,40].
Hydrogen can also be stored as a cooled liquid at a temperature lower than −253°C, or stored as a component in other liquids such as NaBH4 solutions, saturated organic liquids, or anhydrous ammonia, where liquid hydrogen has a density of 70.8 kg/m3 at a pressure of 13 bar, and when it reaches a temperature of −240°C, it begins to change its phase from gas to liquid at critical point, anyway, liquefaction is an energy intensive process and about 40%–45% of hydrogen LHV is used for the process [33]. Hydrogen is stored in refrigerated vessels, also known as coolers. These vessels are double-walled with insulation between the walls and this insulation is important to reduce heat flow incoming from environment. Liquid nitrogen is also used as an inert to further reduce heat transfer. Liquid hydrogen has a high storage density at relatively low pressure, thus its stored energy is much greater than compressed hydrogen. For this reason, ultra-isolated tanks must be used, to avoid risks of boiling off [41,42]. Liquid hydrogen can be transported by tank trucks under conditions that prevent liquid hydrogen from boiling by making shields of liquid nitrogen to cool the outer wall of the liquid hydrogen container to reduce heat transfer. These tanks have large carrying capacity between 360 and 4300 kg of liquid hydrogen. Liquefied hydrogen can also be transported by Railcars with greater capacities, estimated between 2300 and 9100 kg, and sea ships for long distances so that the barge can carry 21,200 to 700,000 kg of liquid hydrogen. Liquid hydrogen can be transported by tubes, but these tubes should be very well insulated [39].
The transportation of liquid hydrogen is approximately 3.5–4 times more expensive than transporting liquefied natural gas. This is due to boiling losses, which account for 10%–20% and can even reach 50% of its transportation capital cost. The costs for transporting liquefied hydrogen vary from 1.8 to 2.1 $/kg, and these values may triple for very long distances [39].
Researchers are currently investigating an alternative storage option for fuel cell vehicles. Their research are mainly based on the composition of a material containing a large amount of hydrogen. In principle, there are two ways to store hydrogen in a solid material, which differ in the way hydrogen bonds to metals, so the storage situation according to two mechanisms can be chemisorption or physisorption.
Materials in which hydrogen is chemisorbed, such as magnesium hydride, release the hydrogen at temperatures well above of the room temperature, around 550–600 K. The hydrogen is stored by exposing the material to hydrogen also at elevated temperature and pressure. In contrast, materials on which hydrogen is physiosorbed, such as carbon nanotubes, release hydrogen at room temperatures. Further, only very low temperatures, of the order of 77 K, are required to store hydrogen within these materials. Both types of materials, however, require energy either to heat the material to release the hydrogen or to cool the material to store the hydrogen [41,42].
Chemisorption:
This method relies on the formation of a chemical bond between metal and hydrogen atoms. It involves breaking the bond of hydrogen molecules, resulting in new individual hydrogen atoms. These atoms form a strong bond with the solid substance through chemical reactions, leading to hydrogen absorption, like the process observed in metal hydrides. For chemical removal, molecular hydrogen dissociation is necessary, storing hydrogen as individual atoms. To facilitate this dissociation, transition metals can be added to the material, as the electrons of transition metals play a crucial role in breaking the hydrogen bond [42].
Physisorption:
H2 molecules are adsorbed on the surface of the solid material by physical attraction alone, as no new bonds are formed due to the presence of strong Van der Waals forces that lead to hydrogen absorption such as porous carbon materials, where they have weak bonds and the energy required is relatively low to release the stored hydrogen. Hydrogen-adsorbed materials depend on their surface area for hydrogen storage and tend to have low volumetric capacities. However, they are generally based on the element carbon [43].
Attention has been drawn to the storage of hydrogen into carbon nanotubes, nanofibers, and more recently, microporous carbons which are considered very promising due to their low molar mass and chemical stability. In large quantities it is relatively inexpensive [43]. This technology is still very expensive and reaches more than 10 $/kg, in addition to the problems of hydrogen release, which makes it difficult to reach the actual and economic application of this technology.
Metal hydrides can be used for solid hydrogen transport by absorbing hydrogen molecules with a metal hydride, then loading the entire container onto a truck or railcar for transport to the customers site where it can be exchanged for an empty hydride container or used as a conventional tanker [39].
For transportation of hydrogen using metal hydrides, the major cost is the capital expense of buying the metal hydride, once filled the hydride containers can be shipped like any other piece of freight, with charges depending on the distance and weight.
Fuel cells that operate by cathode, anode and proton exchange membrane are one of the best ways to electricity production from hydrogen with high efficiency from 40% to 60% at temperatures up to 800°C. There is a big difference between the prices of fuel cells according to the manufacturers, as costs range between 1150 and 8500 $/kW [34], and the price of electricity produced from these cells differed according to their efficiency and capital costs, as the LCOE amounted to between 0.07 and 0.3 $/kWh. If the year 2020 is taken into account, the cost of producing hydrogen by fuel cells is about 4 to 5 $/kg. Table 3 shows the technical and economic details of production, storage, transportation and use of hydrogen. All details about hydrogen production were given by use PEM method, also, the economic details for storing hydrogen in gaseous state were given, which is the easiest and cheapest way to store hydrogen. In addition, transporting hydrogen methods in different ways and their costs were all according to the sizes available in labor market, finally, cost details of electricity production by using fuel cells.
Using the technical and economic information and using Eqs. (1) and (2), LCOE and LCOS values can be calculated for each major component in hydrogen or hydroelectric storage projects, taking into account the direct and significant impact of LCOE of the various sources used in storage process. For this reason, the study is limited to calculating energy storage costs production of renewable sources, namely wind, solar, tidal and biomass.
Hydroelectric energy storage systems are characterized by several features, such as their high efficiency and long lifespan, which makes them qualified to be among the best, most efficient and economical storage systems compared to many renewable systems.
By examining the details of each component and calculating the LCOE for projects in various regions over the past decade, the following costs were found. For projects in North America, Europe, China, and many other Asian countries, reservoir costs are between 0.008 and 0.0187 $/kWh, with an average of 0.015 $/kWh, while the tunnel cost is between 0.015 and 0.005 $/kWh, and the energy house is the most important and expensive component, with a cost between 0.009 and 0.029 $/kWh, on average, it reaches 0.022 $/kWh, As for engineering and construction, it consumes costs between $0.0033 and 0.085 $/kWh. Ownership costs range from 0.0042 to 0.013 $/kWh, while any other costs bear low rates of approximately 0.009 $/kWh. By collecting all various components cost, it was found that the total cost is between 0.044 and 0.1 $/kWh, which is the cheapest cost considering any renewable energy source, and this cost is expected to decrease during the coming years, thanks to the continuous development of technologies that may increase project efficiency and the energy production, of course reducing produced energy costs. Fig. 8 shows all component costs of the hydropower project, giving a clear visualization of difference in costs range for different parts, and it also gives overall value range of LCOE.
Figure 8: Cost details of the components of hydropower project
When using the hydropower project as a storage system for renewable energy sources or so-called hybrid systems these systems are not expensive. Fig. 9 shows LCOE values for all renewable energy sources that can use the hydropower system for energy storage. The figure shows that the hybrid systems integrated with wind, solar, biomass and hydropower storage, can attain an average LCOE between 0.1 and 0.18 $/kWh, which is the cheapest value for that.
Figure 9: LCOE values for renewable energy sources with hydropower storage system (Larger triangles represent the most probable values)
The LCOE value may increase slightly if combined with Tidal power because its cost is slightly high, but that high value of tidal power drops clearly to more than 20% when combined with low-cost sources such as a hybrid system with multiple sources (source-source-Hydropower storage), where the average decreased from 0.27 to 0.2 $/kWh, which is also low compared to the significant increase in the cost of electricity in all countries, especially during peak time.
Since the hydrogen production process goes through several stages, it is natural to calculate all the components which affect the LCOE. The cost of water splitting into hydrogen and oxygen is the highest cost, ranging from 0.013 to 0.021 $/kWh, with an average approaching 0.017 $/kWh, followed by storage in the gaseous state, which is also considered a high cost, ranging from 0.01 to 0.018 $/kWh, while the cost of fuel cells is close to 0.01 to 0.012 $/kWh. Other costs may reach an average of 0.0035 and sometimes rise to 0.004 $/kWh. It should be noted that some projects require the transfer of hydrogen from one region to another, others are decentralized production and consumption so that they do not need to transport hydrogen and increase costs. Thus, the costs of transportation are also high, as they include storage and cooling costs in tankers, containers, and pipes. Therefore, the transportation cost must be calculated and the necessary costs for the production, storage, and use of transported hydrogen and non-transported hydrogen used directly after storage. Transportation costs have been found to be between 0.009 and 0.014 $/kWh, with an average of 0.012. Thus, the total costs of energy produced from hydrogen without transportation ranges from 0.04 to 0.054 $/kWh, with an average of 0.047 $/kWh. As for transportation, the cost may increase to 0.05 to 0. 068 $/kWh, with an average of 0.06 $/kWh, as shown in Fig. 10.
Figure 10: Cost details of the components of hydrogen project
LCOE resulting from hydrogen storage varies according to the type of energy source used to produce and store hydrogen. Fig. 11 shows the value of LCOE resulting from the hybrid renewable energy systems used to produce energy through the hydrogen storage system, that it appears that the cost of energy production from integrating the wind with the hydrogen storage system ranges between 0.118 and 0.224 $/kWh and it appears that the most likely average is between 0.13 and 0.15 $/kWh (the most probable averages appear as larger triangles), while solar-hydrogen is between 0.125 and 0.275 $/kWh, with an average of 0.18 $/kWh. Biomass-hydrogen system is close to the previous systems, as It has values between 0.125 and 0.225 $/kWh, with an average of about 0.16 $/kWh, and Tidal system is considered the largest value of LCOE, reaching between 0.245 and 0.425 $/kWh, with an average of 0.31 $/kWh. As for the multi-source hybrid systems tow renewable sources-hydrogen storage system, it is better merging a low-cost system with a high-cost system works to balance the cost and avoid the high costs of some renewable sources, however, it is crucial to consider the specific characteristics of the project intended to meet the energy requirements of a given region. This involves studying the allocation of the total project cost in suitable proportions relative to the potential resources in the region. Subsequently, each energy source would cover a specific percentage of the energy needs based on its efficiency. It is noted that solar-wind and biomass system associated with the hydrogen storage system show low values between 0.121 and 0.261 $/kWh, while when these sources are combined with tides, they present values between 0.181 and 0.347 $/kWh, with an average approaching 0.245 $/kWh, which is an appropriate and reasonable cost for use, especially when comparing its cost to the cost of energy at peak use.
Figure 11: LCOE values for renewable energy sources with hydrogen storage system (Larger triangles represent the most probable values)
The cost of hydrogen produced from renewable sources can also be calculated so that the value per kilogram of hydrogen can also be known according to the type of source. Fig. 12 shows the cost of hydrogen produced from various renewable energy sources, as it turns out that the cost of hydrogen may decrease or increase according to the type of energy source used for the production process. At the minimum, the value of hydrogen produced from renewable sources reaches 4.65 $/kg, and the upper limit varies according to the type of source. It ranges from 6.7 to 13 $/kg.
Figure 12: Cost of hydrogen production from renewable energy sources
This study demonstrates that using renewable energy sources combined with storage methods such as hydropower and hydrogen systems is not only viable but also economically competitive compared to the anticipated high future costs of fossil fuel-based energy, particularly during peak consumption periods. The investigation provides accurate calculations for the Levelized Cost of Electricity (LCOE) when using renewable sources, hydropower, and hydrogen.
For hydropower storage systems, the LCOS ranges from $0.044 to $0.1 per kWh. When integrated with renewable energy sources in hybrid systems, the total LCOE ranges from $0.08 to $0.28 per kWh. This combination results in a cost-effective and integrated renewable system, making it a viable solution for large-scale energy storage and supply.
Hydrogen storage systems have an LCOS ranging between $0.04 and $0.054 per kWh without transportation. Including transportation, the cost increases to between $0.05 and $0.068 per kWh. When combined with renewable energy sources, the total LCOE ranges from $0.118 to $0.425 per kWh. This cost is competitive, especially if suitable sources for hydrogen production and storage are selected.
The efficiency and cost-effectiveness of hybrid renewable energy systems can be enhanced through the appropriate selection of integrated renewable sources. This involves considering the efficiency and availability of sources across different seasons, optimizing for both performance and economic viability.
Finally, the study proves that producing and storing energy using hydropower and hydrogen systems can be affordable and competitive in the global energy markets. These hybrid systems not only support the stability and reliability of renewable energy but also contribute to reducing greenhouse gas emissions and minimizing environmental impact. This research provides valuable insights for policymakers, investors, and industry stakeholders looking to transition to a more sustainable and resilient energy future.
Implications and Future Directions
• Scalability and Integration: The findings suggest that scalable and integrated renewable energy systems with effective storage solutions can significantly reduce dependency on fossil fuels, particularly during peak demand periods.
• Policy and Investment: Policymakers and investors should consider supporting the development and deployment of hybrid renewable energy systems, emphasizing the integration of hydropower and hydrogen storage to achieve economic and environmental benefits.
• Technological Advancements: Continued research and development in storage technologies, particularly in improving the efficiency and reducing the costs of hydrogen production and storage, are crucial for the future of renewable energy systems.
• Seasonal and Geographic Optimization: Further studies should explore the optimal combinations of renewable sources tailored to specific geographic and seasonal conditions, maximizing the overall efficiency and cost-effectiveness of hybrid systems.
Acknowledgement: I would like to express my sincere gratitude to my main supervisor, Biagio Morrone, for his invaluable guidance, support, and expertise throughout this research. I am also deeply thankful to my second supervisor, Adil Echchelh, for his feedback and supervision. Their combined mentorship has been instrumental in the successful completion of this work. Lastly, I extend my appreciation to my family and friends for their unwavering encouragement and understanding during this project.
Funding Statement: The authors received no specific funding for this study.
Author Contributions: Saif Serag was responsible for data collection, analysis, application, drawing, and formulating the conclusions of this study. Biagio Morrone contributed through review, modification, amendment, supervision, and final approval of the manuscript. Adil Echchelh provided supervision and approval of the work. All authors reviewed the results and approved the final version of the manuscript.
Availability of Data and Materials: The authors ensure the authenticity and validity of the materials and data in the article.
Ethics Approval: Not applicable.
Conflicts of Interest: The authors declare that they have no conflicts of interest to report regarding the present study.
References
1. P. A. Owusum and S. Asumadu-Sarkodie, “A review of renewable energy sources, sustainability issues and climate change mitigation,” Cogent Eng., vol. 3, no. 1, pp. 1–14, Apr. 2016. doi: 10.1080/23311916.2016.1167990. [Google Scholar] [CrossRef]
2. M. Y. Worku, “Recent advances in energy storage systems for renewable source grid integration: A comprehensive review,” Sustainability, vol. 14, no. 10, pp. 5985, May 2022. doi: 10.3390/su14105985. [Google Scholar] [CrossRef]
3. CNESA, “Global energy storage market analysis—2020,” Nov. 27, 2020. Accessed: Nov. 17, 2020. [Online]. Available: http://en.cnesa.org/latest-news/2020/11/17/cnesa-global-energy-storage-market-analysis2020q3-summary [Google Scholar]
4. W. He, M. King, X. Luo, M. Dooner, D. Li and J. Wang, “Technologies and economics of electric energy storages in power systems: Review and perspective,” Adv. Appl. Energy, vol. 4, no. 4, Nov. 2021, Art. no. 100060. doi: 10.1016/j.adapen.2021.100060. [Google Scholar] [CrossRef]
5. CFI Team, “Levelized Cost of Energy (LCOEthe average total cost of a project per unit of total electricity generated,” Jul. 2022. Accessed: Jul. 23, 2024. [Online]. Available: https://corporatefinanceinstitute.com/resources/valuation/levelized-cost-of-energy-lcoe/ [Google Scholar]
6. S. Serag and A. Echchelh, “Technical and economic study for electricity production by concentrated solar energy and hydrogen storage,” Technol. Econ. Smart Grids Sustain. Energy, vol. 7, no. 1, pp. 1–30, Aug. 2022. doi: 10.1007/s40866-022-00154-x. [Google Scholar] [CrossRef]
7. R. P. Sheam and Y. K. Ramgolam, “Applied levelized cost of electricity for energy technologies in a small island developing state: A case study in Mauritius,” Renew. Energy, vol. 132, no. 7, pp. 1415–1424, Mar. 2019. doi: 10.1016/j.renene.2018.09.021. [Google Scholar] [CrossRef]
8. D. Timmons, J. M. Harris, and B. Roach, The Economics of Renewable Energy. Global Development and Environment Institute, Tufts University, 2020. Accessed: Jul. 23, 2024. [Online]. Available: https://www.bu.edu/eci/files/2019/06/RenewableEnergyEcon.pdf [Google Scholar]
9. IRENA, Renewable Power Generation Costs in 2019. Abu Dhabi: International Renewable Energy Agency (IRENA2020. Accessed: Jul. 23, 2024. [Online]. Available: https://www.irena.org/publications/2020/Jun/Renewable-Power-Costs-in-2019 [Google Scholar]
10. C. Kost, S. Shammugam, V. Jülch, T. Nguyen, and T. Schlegl, “Levelized cost of electricity-renewable energy technologies,” 2018. Accessed: Jul. 23, 2024. [Online]. Available: https://www.ise.fraunhofer.de/content/dam/ise/en/documents/publications/studies/EN2018_Fraunhofer-ISE_LCOE_Renewable_Energy_Technologies.pdf [Google Scholar]
11. C. M. Costa, J. C. Barbosa, R. Gonçalves, H. Castro, F. J. D. Ampo and S. L-Méndez, “Recycling and environmental issues of lithium-ion batteries: Advances, challenges and opportunities,” Energy Storage Mater., vol. 37, no. 12, pp. 1–97, May 2021. doi: 10.1016/j.ensm.2021.02.032. [Google Scholar] [CrossRef]
12. O. Schmidt, S. Melchior, A. Hawkes, and I. Staffell, “Projecting the future levelized cost of electricity storage technologies,” Joule, vol. 3, no. 1, pp. 81–100, Jan. 2019. doi: 10.1016/j.joule.2018.12.008. [Google Scholar] [CrossRef]
13. International Energy Agency (IEA“The future of hydrogen: Seizing today’s opportunities,” Jun. 2021. Accessed: Jul. 23, 2024. [Online]. Available: https://www.iea.org/reports/the-future-of-hydrogen [Google Scholar]
14. S. Serag and A. Echchelh, “Technical and economic evaluation of electricity generation and storage using renewable energy sources on Socotra Island, Yemen,” Iraqi J. Sci., vol. 64, no. 6, pp. 2809–2842, Jun. 2023. doi: 10.24996/ijs.2023.64.6.14. [Google Scholar] [CrossRef]
15. R. B. Alley, “EARTH 104: Earth and the environment (Development),” 2021. Accessed: Jul. 23, 2024. [Online]. Available: https://www.e-education.psu.edu/earth104/node/1060 [Google Scholar]
16. Hydro Review, “Despite growth, hydropower installation needs to increase further, IHA says,” Nov. 6, 2021. Accessed: Nov. 6, 2023. [Online]. Available: https://www.hydroreview.com/hydro-industry-news/despite-growth-hydropower-installation-needs-to-increase-further-iha-says/#gref [Google Scholar]
17. IRENA, “Renewable energy technologies: Cost analysis series (Hydropower),” vol. 1, no. 3/5, Jun. 2012. Accessed: Jul. 23, 2024. [Online]. Available: https://www.irena.org/-/media/Files/IRENA/Agency/Publication/2012/RE_Technologies_Cost_Analysis-HYDROPOWER.pdf [Google Scholar]
18. D. A. Harpman, “Exploring the economic value of hydropower in the interconnected electricity system, United States Department of the Interior Bureau of Reclamation,” 2006. Accessed: Jul. 23, 2024. [Online]. Available: https://www.usbr.gov [Google Scholar]
19. G. Oladosu, L. George, and J. Wells, 2020 Cost Analysis of Hydropower Options at Non-Powered Dams. Oak Ridge, Oak Ridge National Laboratory, Mar. 2021, TN 37831-6283. Accessed: Jul. 23, 2024. [Online]. Available: https://info.ornl.gov/sites/publications/Files/Pub145012 [Google Scholar]
20. K. Mongird, V. Viswanathan, J. Alam, C. Vartanian, and V. Sprenkle, “Grid energy storage technology cost and performance assessment,” Dec. 2020. Accessed: Jul. 23, 2024. [Online]. Available: https://www.pnnl.gov [Google Scholar]
21. N. Gregus, “What is operations and maintenance,” 2022. Accessed: May 4, 2022. [Online]. Available: https://goenergylink.com [Google Scholar]
22. P. Lako, Hydropower. IEA, ETSAP Technology Brief, May 2021. Accessed: Jul. 23, 2024. [Online]. Available: https://iea-etsap.org/E-TechDS/PDF/E06-hydropower-GS-gct_ADfina_gs.pdf [Google Scholar]
23. D. Gielen et al., Renewable Power Generation Costs in 2020. Abu Dhabi: International Renewable Energy Agency, Jun. 2021. Accessed: Jul. 23, 2024. [Online]. Available: www.irena.org [Google Scholar]
24. E. Tzimas, C. Filiou, S. D. Peteves, and J. B. Veyret, “Hydrogen storage: State-of-the-art and future perspective,” in Institute for Energy Petten, Netherlands: European Communities, 2003. Accessed: Dec. 16, 2022. [Online]. Available: http://europa.eu.int/ [Google Scholar]
25. U. Y. Qazi, “Future of hydrogen as an alternative fuel for next-generation industrial applications; Challenges and expected opportunities,” Energies, vol. 15, no. 13, pp. 1–40, Jun. 2022. doi: 10.3390/en15134741. [Google Scholar] [CrossRef]
26. G. Bristowe and A. Smallbone, “The key techno-economic and manufacturing drivers for reducing the cost of power-to-gas and a hydrogen-enabled energy system,” Hydrogen, vol. 2, no. 3, pp. 273–300, Jun. 2021. doi: 10.3390/hydrogen2030015. [Google Scholar] [CrossRef]
27. A. Züttel, “Hydrogen storage methods,” Naturwissenschaften, vol. 91, no. 4, pp. 157–172, Mar. 2004. doi: 10.1007/s00114-004-0516-x. [Google Scholar] [PubMed] [CrossRef]
28. H. A. Miller et al., “Green hydrogen from anion exchange membrane water electrolysis: A review of recent developments in critical materials and operating conditions,” Sustain. Energy Fuels, vol. 4, no. 5, pp. 2114–2133, Mar. 2020. doi: 10.1039/C9SE01240K. [Google Scholar] [CrossRef]
29. Z. Luo, Y. Hu, H. Xu, D. Gao, and W. Li, “Cost-economic analysis of hydrogen for China’s fuel cell transportation field,” Energies, vol. 13, no. 24, pp. 6522, Dec. 2020. doi: 10.3390/en13246522. [Google Scholar] [CrossRef]
30. S. S. Kumar and V. Himabindu, “Hydrogen production by PEM water electrolysis–A review,” Mat. Sci. Energy Technol., vol. 2, no. 3, pp. 442–454, Dec. 2019. doi: 10.1016/j.mset.2019.03.002. [Google Scholar] [CrossRef]
31. E. H. Taibi, Blanco, R. Miranda, IRENA, Green Hydrogen Cost Reduction: Scaling up Electrolysers to Meet the 1.5°C Climate Goal. Abu Dhabi: International Renewable Energy Agency, Dec. 2020. Accessed: Jul. 23, 2024. [Online]. Available: www.irena.org/publications [Google Scholar]
32. J. B. David, H. Cassidy, H. K. Jennie Moton, and D. Daniel, Final Report: Hydrogen Storage System Cost Analysis. USA: U.S. Department of Energy Office of Scientific and Technical Information, Sep. 30, 2016. doi: 10.2172/1343975. [Google Scholar] [CrossRef]
33. Gupta, Hydrogen Storage, Distribution and Cleaning. SWEDEN: Degree Project in Chemical Science and Engineering, Second Cycle, 30 Credits Stockholm, Jul. 2021. Accessed: Jul. 23, 2024. [Online]. Available: https://www.diva-portal.org [Google Scholar]
34. Team Vskills, “Hydrogen storage,” Accessed: Aug. 23, 2021. [Online]. Available: https://www.vskills.in/certification/tutorial/hydrogen-storage/ [Google Scholar]
35. C. E. G. Padro and V. Putsche, “Survey of the economics of hydrogen technologies,” NREL/TP-570-27079, 12212. Sep. 1999. Accessed: Jul. 23, 2024. [Online]. Available: https://www.nrel.gov/docs/fy99osti/27079.pdf [Google Scholar]
36. E. A. Harvego, J. E. James O’Brien, and M. G. McKellar, “System evaluations and life-cycle cost analyses for high-temperature electrolysis hydrogen production facilities,” INL/EXT-12-25968, 1047199, May 2012. Accessed: Jul. 23, 2024. [Online]. Available: https://inldigitallibrary.inl.gov/sites/sti/sti/5436986.pdf [Google Scholar]
37. N. Dopffel, NORCE Norwegian Research Centre, Bridging the Gap to a sustainable Future: Underground Hydrogen Storage. Norce, May 10, 2021. Accessed: Aug. 23, 2021. [Online]. Available: https://www.norceresearch.no/en/news/bridging-the-gap-to-a-sustainable-future-underground-hydrogen-storage [Google Scholar]
38. G. W. Mair, S. Thomas, B. Schalau, and B. Wang, “Safety criteria for the transport of hydrogen in permanently mounted composite pressure vessels,” Int. J. Hydrogen Energy, vol. 46, no. 23, pp. 12577–12593, Mar. 2021. doi: 10.1016/j.ijhydene.2020.07.268. [Google Scholar] [CrossRef]
39. W. A. Amos, “Costs of storing and transporting hydrogen,” NREL/TP-570-25106, ON: DE00006574, 6574, Nov. 1998. Accessed: Jul. 23, 2024. [Online]. Available: https://www.nrel.gov/docs/fy99osti/25106.pdf [Google Scholar]
40. C. Yang and J. Ogden, “Determining the lowest-cost hydrogen delivery mode,” Int. J. Hydrogen Energy, vol. 32, no. 2, pp. 268–286, Jul. 2006. doi: 10.1016/j.ijhydene.2006.05.009. [Google Scholar] [CrossRef]
41. B. Moore, Grand View Research, “Hydrogen storage industry trends & opportunities,” 2021. Accessed: Jul. 23, 2024. [Online]. Available: https://www.grandviewresearch.com/research-insights/hydrogen-storage-market-insights-size-trends [Google Scholar]
42. R. Krishna, E. Titus, M. Salimian, O. Okhay, S. Rajendran and A. Rajkumar, “Hydrogen storage for energy application,” in J. Liu (Ed.Hydrogen Storage, IntechOpen Journals, Sep. 5, 2012. https://www.intechopen.com/chapters/38711 [Google Scholar]
43. M. Mohan, V. K. Sharma, E. A. Kumar, and V. Gayathri, “Hydrogen storage in carbon materials—A review,” Energy Storage, vol. 1, no. 2, pp. e35, Mar. 2019. doi: 10.1002/est2.35. [Google Scholar] [CrossRef]
44. L. Lachell, “Fuel cells: What they are and how they work,” 2022. Accessed: Dec. 14, 2022. [Online]. Available: https://www.plugpower.com/fuel-cells-what-they-are-and-how-they-work/ [Google Scholar]
45. J. Vickers, D. Peterson, and K. Randolph, “Cost of electrolytic hydrogen production with existing technology,” 2023. Accessed: Sep. 22, 2020. [Online]. Available: https://www.hydrogen.energy.gov [Google Scholar]
46. J. Hinkley, J. Hayward, R. McNaughton, and R. Gillespie, Cost Assessment of Hydrogen Production from PV and Electrolysis. Australia: CSIRO, 2016. Accessed: Mar. 21, 2021. [Online]. Available: www.csiro.au [Google Scholar]
47. J. Yates et al., “Techno-economic analysis of hydrogen electrolysis from off-grid stand-alone photovoltaics incorporating uncertainty analysis,” Cell Rep. Phy. Sci., vol. 1, no. 10, Oct. 2020, Art. no. 100209. doi: 10.1016/j.xcrp.2020.100209. [Google Scholar] [CrossRef]
48. J. Davies, F. Dolci, and E. Weidner, “Historical analysis of FCH 2 JU electrolyser projects, European commission,” 2021. Accessed: Apr. 14, 2021. [Online]. Available: https://publications.jrc.ec.europa.eu/repository/handle/JRC121704 [Google Scholar]
49. D. J. Jovan and G. Dolanc, “Can green hydrogen production be economically viable under current market conditions,” Energies, vol. 13, no. 24, pp. 6599, Dec. 2020. doi: 10.3390/en13246599. [Google Scholar] [CrossRef]
50. K. McMurphy, T. Benjamin, A. Wilson, Battelle Memorial Institute, “Manufacturing cost analysis of PEM fuel cell systems for 5- and 10-kW backup power applications,” Oct. 2021. Accessed: Jul. 23, 2024. [Online]. Available: https://www.energy.gov [Google Scholar]
Cite This Article
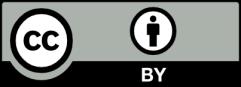
This work is licensed under a Creative Commons Attribution 4.0 International License , which permits unrestricted use, distribution, and reproduction in any medium, provided the original work is properly cited.