Open Access
ARTICLE
Hybrid Energy Systems and the Logic of Their Service-Dominant Implementation: Screening the Pathway to Improve Results
1 National Technical University of Ukraine “Kyiv Polytechnic Institute”, Kyiv, 03056, Ukraine
2 Institute of General Energy of NAS of Ukraine, Kyiv, 03150, Ukraine
3 Interregional Academy of Personnel Management, Kyiv, 02000, Ukraine
* Corresponding Author: Oleksandr Novoseltsev. Email:
Energy Engineering 2023, 120(6), 1307-1323. https://doi.org/10.32604/ee.2023.025863
Received 02 August 2022; Accepted 14 March 2023; Issue published 03 April 2023
Abstract
The transition of the global economy to a low-carbon development path has led to dramatic changes in the organization and functioning of energy markets around the world, where hybrid energy systems (HESs) are one of the decisive active agents. At the same time, a number of problems facing the modern HESs are primarily due to the stochastic nature of the renewable energy they use, require further profound changes not only in the technologies they use and how they manage them, necessary to meet the needs of end consumers and interact with the unified energy system, but also to preserve the ability of the environment to self-heal. In order to make the process of changes more efficient and eco-deep, the article proposes to use and discusses the approach based on service-dominant (SD) logic, which opens up new opportunities for solving the problems of HESs. First of all through: the implementation of closer service interaction with other participants in the energy markets, as well as with the environment; a systemically organized process of transforming the “product” economic activity of HESs into a service-dominant one; developing the generalized and engineering models for solving the problems of optimizing the technical and economic indicators of HESs, operation in steady-state and transient modes. The calculations confirm the effectiveness of the proposed approach and its ability to reduce the average daily costs for the system as a whole by 14.7% compared to the costs with a uniform distribution of power between the modules.Keywords
The transition of the world economy to low-carbon development, driven mainly by pollution from the burning of fossil fuels, has caused fundamental changes in the organization and functioning of energy markets. These markets that were centralized decades ago, mainly operating with conventional types of energy resources, are now becoming decarbonized and decentralized with active involvement of prosumers in the processes of energy production and consumption. Today’s energy markets are shifting to a real-time model, taking into account the widespread use of solar and wind power plants, energy storages, electric vehicles, etc., based on smart technologies and virtual organization management. On the one hand, this creates more problems with ensuring the reliability and stability of the operation of energy systems, and on the other hand—opens up more opportunities for energy efficiency (EE) and renewable energy sources (RES).
There are three closely interconnected directions (design solutions) for solving of the above problems, which are currently being implemented through: increasing the flexibility of the centralized energy system; carrying out its decentralization; and implementation of hybrid energy systems (HESs). But what is already clear today is that: the new format (type) of a centralized energy system should be based on a modular structure with deep regulation of the generated energy; the decentralized energy system should consolidate local prosumers; and HESs—integrates different types of clean conventional and renewable generation. It is also clear that:
• Each type of these systems, taken (considered) separately, has its own advantages and disadvantages, and their consolidation should open up new opportunities for using synergistic effects;
• None of the types of energy systems being considered for implementation should be inferior to others in terms of energy efficiency, ecology and energy security;
• All energy systems selected for implementation must be integrated, coordinated and balanced as subsystems within the framework of unified (united, bulk, also) energy system.
Here it is necessary to emphasize that the decision-making process for choosing the optimal structure and operation parameters (modes) of the unified energy system (UES) is a process of multi-level, multi-objective optimization taking into account the specifics of the scale, location, connectivity, proximity, flexibility and manageability of the selected subsystems, as well as their differences in energy, economic and ecological characteristics [1,2].
Reviews of problematic issues containing more than 160 references covering most aspects of the comparative analysis of centralized and decentralized energy systems operating in electricity markets can be found, for example, in [3]. In our analysis, we single out hybrid power systems as an important class of UES, characterized by wide possibilities for using different types of power plants operating on different types of energy sources, including, as a rule, RES. In addition to traditional power plants, these can be energy storage devices such as pumped hydroelectric power plants, compressed air systems, hydrogen systems, super capacitors, etc., thermal energy sources (biogas plants, solar thermal collectors) and organic biofuel sources (e.g., diesel generators) that act as backup power. Given the renewable nature of the RES and the ever-increasing prices for oil and natural gas, HESs with renewable sources are already successfully competing with traditional ones today [4].
Autonomous (i.e., not connected to a centralized network) energy systems that use only certain types of renewable energy (solar, wind, etc.), in real time conditions of variability of weather/climate, do not always meet the requirements of consumers for the reliability and quality of the generated energy. Obviously, a system that combines several types of renewable energy, especially with other types of clean energy, becomes more efficient in terms of price, reliability and productivity. Such a system, referred to as a hybrid system, is becoming the fastest growing element of energy markets worldwide [5,6].
Hybridization makes it possible to improve the technical and economic parameters of energy systems with RES; however, implies the optimal choice of energy sources, technologies and modes of their operation, which ultimately determines the methodology for designing such systems, including the solution of complementary tasks of dispatching and control.
One of the modern directions for the development of HESs is microgrids, which are self-sufficient power systems that serve discrete geographical areas, providing energy to nearby consumers [7,8]. This allows, firstly, to overcome the inefficiency of centralized grids, which have large losses during transmission and distribution—up to 8%–15%, and secondly, to disconnect from the central grid at any time and operate autonomously using a microgrid controller that manages available energy resources in order to achieve the goals set by microgrid customers in cases of unforeseen power outages, scheduled repairs or for other reasons. For its part, autonomous mode requires an independent solution of a whole range of issues at the consumption level; first of all, this is the quality of electricity generation, its excess losses, as well as billing problems [9,10].
Despite the advantages of microgrids systems, it should be emphasized that their complete autonomy and the rejection of the services of a UES are unrealistic and/or unprofitable, since such systems need at least qualified management, even not to mention the advantages of energy exchange between them.
Thus, for the efficient operation of microgrid systems as a self-sufficient part of a UES, an exchange of energy flows with the levels of transmission and distribution of a unified system will be required, that can be realized using new approaches to the organization and management of the system, based on smart information technologies [2]. One of these approaches is based on the principles of building so-called transactive energy systems, which, in contrast to the traditional hierarchical approach, promote a network environment. This makes transactive energy systems more interoperable and flexible due to the wide possibilities of direct interaction of each node of such a network with others (also between levels of the unified system), thereby improving the efficiency and reliability of the system, increasing customer satisfaction and reducing energy costs, etc. [11–13]. The requirements for developing an effective mechanism for transaction management, taking into account various market scenarios and pricing mechanisms, focused on meeting the needs of a consumer, are discussed in detail in [14]. Summing up the above analysis, it should be noted that the difficulties of introducing hybrid energy systems are associated not only with the variability of renewable energy, but also with the urgent need to develop a business paradigm that opens up new, more competitive forms and rules for the organization and operation of HESs in the energy products and services markets. One of the promising innovative approaches to solving this complex problem analysed in our work is service-dominant (SD) logic, but today it has been developed in detail at the level of descriptive explanation [15], which in our case requires its formalization in order to make numerically sound decisions.
Thus, the main objective of the work is to develop a methodological framework and a multi-level model based on the SD approach to improve the competitiveness of HESs due to their performance-based interaction with all subcontractors in the markets of energy products and services, as well as with end-users.
The advantages of the proposed approach to improving the competitiveness of HESs are that the economic interest of subcontractors will not be in the provision of marketable products and services, but in the final results of their utilization by consumers. This obliges subcontractors not only to supply high-quality products, but also to guarantee the effectiveness and efficiency (energy, economic, environmental) of its operation by providing services within the period stipulated by the performance contract.
To achieve the objective, the following sequence of two main steps was applied. The first step is to analyze the main capabilities of the SD approach in order to identify methodological requirements that can be reconciled with the task of increasing the competitiveness of HESs in the markets of energy products and services and as a result, clarify the stages of their implementation within the framework of performance management. At the second step, a multilevel model is constructed that formalizes the identified competitive capabilities by a system of generalized equations with their subsequent adaptation for engineering calculations and analysis.
The novelty of this work is that:
• At the methodological level, a systemically organized process of transforming the “product” economic activity of HESs into a service-dominant one is proposed. Achievements also include identifying potential performers and developing a continuous improvement management cycle to drive such transformation.
• At the generalized model level, the problem of optimizing a complex energy system, taking into account the energy, economic and environmental aspects of its operation is formalized on the basis of the set-theoretic approach. A system of corresponding target functions is developed;
• At the level of an engineering model, an algorithm is developed for numerical solving the problems of optimizing the technical and economic indicators of HESs operation, covering the tasks of minimizing costs in the transmission and distribution of electricity, optimizing power take-off from generators, and mitigating the negative impact of transients when the load changes.
The practical implications of this work are to provide designers as well as practicing engineers with a methodological framework for improving the competitiveness of HESs by making more informed, performance-based decisions about the actions to be taken to improve it.
2 The Logic of Service-Dominant Approach
In the last decade, the service-dominant (SD) logic [16] has become increasingly in demand as a means of creating innovative solutions in which manufacturing and service companies pool their resources with retail customers to encourage the efficient production and sale of products and services while reducing business costs. This means that consumers become full participants in the SD market in the production and use of goods and services [17,18]. Very closed to the SD logic is the concept of product-service systems (PSS) [18,19]. Their mutually agreed integration (denote it as a SD-approach) contributes to a closer and longer-term relationship, which means better mutual understanding and more profitable commodity-money transactions, including in the implementation of EE and RES projects [20–22].
Among other advantages of the SD-approach in the implementation of EE and RES projects, the following two should be highlighted. First, result orientation, when the synergy of interaction between manufacturing and service companies with customers is aimed at effectively meeting the final needs of consumers in lighting, heating, cooling, etc. Second, the focus on demand management (low cost planning, integrated resource management, etc.) originated in the US energy sector in order to introduce more cost-effective services to reduce energy demand than to increase generating capacity.
The energy services market within the framework of the SD-approach is actually determined by a systemically organized energy community of participants united by a network structure of interconnections, which, depending on the level of their integration, can cover both the national and local levels and even the level of specific energy-intensive equipment.
Considering the complexity and a significant number of geographically dispersed and, as a rule, legally independent participants, the organization of such energy communities in practice has become possible only on the basis of smart information platforms created in a virtual (cloud) environment and functioning in conditions of competitive interaction between participants [23,24].
Here we should state that from the point of view of management theory, such a community is a complex socio-technical system that unites business entities acting in energy sector (e.g., energy cooperatives, microgrids, prosumers, etc.), each of which produces (uses and/or provides) separate, but systemically consistent with all other entities, products and/or services, which together form a single business solution for end users. The organization of a waste-free cycle in managing the flow of energy, material, financial and economic resources through waste disposal by these entities increases their energy efficiency and expands the spheres of using RES.
Considered within the framework of the SD-approach, such a socio-technical system can be displayed as a set of sub-blocks, each of which reflects the business activity of participants (energy communities) in the primary resource markets, materials and products (both renewable and non-renewable), energy services, secondary resources, materials and products of their processing, as well as in the production market, which rightfully occupies a central place in such frameworks [22].
It is clear that the practical implementation of the SD-approach requires the creation of a virtually organized service center for control, coordination and balancing of the activities of energy communities in the above-mentioned markets on a mutually beneficial basis [24]. Herewith, the center should be able to compare heterogeneous and multi-scale projects of system participants, coordinating their local efficiency and comparing the effects received by energy communities from attracting investments within individual projects with system-wide efficiency. At the same time, the center should organize the process of selecting the best investment proposals without prejudice to the interests of any of the participants, since the deterioration in the efficiency of each of them directly affects the performance of all other participants in the system [25].
One of the most effective and widespread forms of such activities is energy service, which, as a rule, performs most of the functions necessary for a SD business (technical, economic, financial and legal) [20]. Among them are design, engineering, installation, commissioning, monitoring and verification of energy saving measures (projects), including those based on EE and RES, which are carried out by energy service companies (ESCOs) [26–28]. It is important that ESCOs provide energy services to their clients by assuming a certain degree of financial risk and receiving payment (in whole or in part) for the services rendered based on achieving results in improving energy, economic and environmental efficiency.
However, with the traditionally established relations between legally independent providers of energy products and services, in which their own interests in relation to making a profit do not coincide, synergy of interaction between participants is not fully realized outside the SD-approach.
To do this, it is proposed to modify the known mechanism of energy performance contracting (EPC) [20], based on the following principles that should be binding on every provider of energy products and services within the framework of the common EPC, as well as their users:
• To guarantee the quality of products and services provided to clients (subcontractors) within the terms stipulated by each particular EPC, which will improve the economy and/or energy and the environment of the clients;
• To invest own funds (in whole or in part) in the implementation of energy service projects within the EPC on a mutually beneficial basis;
• To carry out a competent restructuring of own business, aimed at improving energy efficiency, competitiveness and the financial stability of all EPC providers, as well as their users.
The development of business relations between providers of products and services within the SD-approach framework is implemented through a systemically coordinated integration of their production and communication processes based on closed performance management cycles. The cycles organized in this way can be thought of as Deming’s continuous improvement process that combines the steps of planning (establish objectives and plans), doing (implement plans), checking (measure and monitor results against planned) and acting (correct and improve plans) into one ongoing performance management process.
In our case, the generalized model that reflects this process consists of at least two system-coordinated closed cycles managed by the service center (Fig. 1). With an internal closed cycle, the agreed harmonization of services provided by business entities in the SD-approach framework is carried out throughout the entire life cycle of the product. These are: primary resource services; product-related services; UES-related services; waste recovery services; and consumer-related services. All these services sequentially go through each step of the external cycle in accordance with the Deming process.
Figure 1: The cycles of the organizational mechanism to support the implementation of the SD-approach
It is clear that the process of performing such complex procedures requires, on the one hand, the involvement of a professional organization, and, on the other hand, methods and models suitable for engineering design. The role of such organizations (let us call them coordination-balancing service centers) is usually performed by ESCOs, while the numerical methods and models necessary for this are acutely scarce, especially for HES.
The last task considered within the framework of the SD-approach is not easy, even from a mathematical point of view, since it is necessary to connect each stage of each service with each other in a systemically balanced and consistent way, while optimizing the resulting process according to a set of technical, economic and environmental criteria.
3 Mathematical Model and Engineering Calculations
3.1 Principles of Building a Generalized Model
Turning structured model of SD-approach, presented in Fig. 1, into a formal mathematical one is an essential for its implementation in practice. To achieve this goal, we used the synergistic combination of energy systems requirements analysis and set theory, reflecting business activity of participants (energy communities) considered above to create a system of adequate equations. First of all, it should be noted that from the point of view of Dubin’s method of structural-component analysis the model shown in Fig. 1 is a three-level vertically organized system of performance management, where coordination-balancing service centers are located at the upper (first) level of this system. The next (second) level of the system is occupied by business entities providing different types of services. The third level is the level considering the stages of the performance management cycle reflected by the external cycle.
In accordance with this, the structured three-level model shown in Fig. 1, can be formalized based on symbolic mappings of set theory and is represented by three mutually consistent systems of equations (the main and two auxiliary ones) [29,30]. The main system of equations in our case will reflect the relationship of input variables into sets of output variables for each level (subsystem) of the model under consideration, and the auxiliary ones will reflect the energy, economic and environmental efficiency of interaction between levels (subsystems) due to direct and feedback links:
where: X—set of input, and Y—set of output variables of the system of equations; U is the set of control variables (direct links), and Z is the set of feedback variables of one subsystem with another (others);
As a result of applying Eq. (1) to formalize the three-level model shown in Fig. 1, we have received a structured procedure for searching and accounting for all possible interactions in the system. This is not an easy task for a three-level model. For example, if you need to take into account n parameters in each of the variables X, U and Z, then this will be a task for n3 different types of their interaction. For further formalization of Eq. (1), we propose to display them in the form of mutually consistent subsystems of balance equations (energy, economic, environmental), linking input and output variables (parameters) for each business entity and the energy system as a whole. This makes it possible to use the logical operations of set-theoretic union or in a linear form—the summation functions.
The linear form of balance equations is most suitable for finding engineering solutions in the HES sphere, since the energy, economic and environmental performance indicators here, as a rule, are subject to the superposition principle. However, the search of the optimal solution for multilevel system based on the totality of energy, economic and environmental objective functions is a vector (multi-criteria) optimization problem, which generally does not have a single solution.
To overcome this limitation, we propose to apply the following 2-step algorithm: firstly, to solve three separate one-criteria optimization problems, according to energy, economic and environmental criteria and then, using the consensus method, to find the quasi-optimal solution at the system level.
The single-objective functions used are as follows:
where:
Note that the solution of optimization problem (1) and (2) is guaranteed, since the objective functions (2) will be deterministic, continuous, differentiable, unimodal and convex if the numerical values of the parameters of these functions you will measure or in monetary units, for example, in thousands of dollars, or in energy units, for example, in kg of oil equivalent (kgoe), kWh, etc.
3.2 Engineering Model and Calculations
The practical use of the above aspects of building a generalized model of the HES functioning will be further considered using one of the examples of microgrid systems, which are actively developing today and are beginning to occupy one of the central places in the structure of the modern electricity generation.
In accordance with the approach of balance equations, the engineering model should allow for a comprehensive analysis of the economic and technical aspects of the microgrid systems functioning, aimed at minimizing costs and losses in the production, transmission and distribution of electricity, as well as optimizing the choice of type and balancing the power of generators, taking into account the influence of transient (dynamic) processes when changing consumer loads. The cost of energy produced by local microgrid systems is in most cases calculated on the basis of economically justified costs, where investment (capital), operating and fuel costs are distinguished for energy technologies [31–33].
3.2.1 Generation and Supply Costs
Consider a microgrid system consisting of the following energy subsystems (modules): wind turbines modules, solar photovoltaic modules, storage battery modules, and uninterruptible power supply modules, the total annual cost C of generating electricity of which consists of the total fixed costs
where: h is the number of energy modules in the system.
The total fixed costs of the microgrid system consist of
where:
Let us represent the costs for each energy subsystem in the following detailed form:
where:
When several modules of different types of subsystems operate in parallel or with their own dependences of costs for power, the total costs are determined as the sum of the generation costs of each:
where: n is the number of wind modules, m is the number of solar modules, v is the number of uninterruptible modules.
Variable system costs are usually associated with the fuel consumption of uninterruptible modules, energy losses for charging and discharging batteries, losses at insufficiently low loads of wind and solar modules, and are mainly due to atypical climatic conditions.
Various methods and models are used to justify the design decisions of HESs, primarily linear and nonlinear programming, genetic, fuzzy logic, neural networks, etc. [34–37]. For example, according to the linear programming method, a savings of about 19% were achieved while minimizing operating costs [33].
3.2.2 Optimization According to Technical and Economic Indicators
To optimize according to technical and economic indicators in the concept of transactive energy, an algorithm improved on the basis of the nonlinear programming method was used. Based on the calculations performed using expressions (3)–(6), the dependences of variables operating costs for power are established, which make it possible to optimize the microgrid system as a whole according to the criterion of minimum costs:
To solve the optimization problem (7), a step by step algorithm for distributing power between modules has been developed, which optimizes the operation of the microgrid system both from the technical and economic side. To do this, at each time interval, taking into account the power generated by the modules in the previous interval, to ensure its current value, the required power take-off from each module is calculated to ensure minimal system costs. Along with this, at each time interval, the system has to be in energy balance, and its total generated power P must be equal to the total power required by consumers and required to charge storage batteries:
where:
Let us specify the possibilities of applying the approach indicated by expressions (1)–(8) using the example of a microgrid system with one wind, one solar and one uninterruptible modules. The operation of the step-by-step algorithm for distributing power between modules in this case consists in solving the following tasks:
1. Setting the initial conditions for each of three generating modules: curves C1(P), C2(P), C3(P) in the form of continuous functions and initial power values P10, P20, P30;
2. Setting the current value of the consumer power Pli and the maximum power taken from the solar and wind modules;
3. Calculation of the required range of the total change in the power of the modules
4. Finding the minimum of the objective function
subject to the following constraints:
where:
5. Calculation of powers required to achieve minimum costs:
The obtained power values are the initial conditions for the next calculation step;
6. The calculation is repeated according to item 2.
Unlike the algorithm built on the basis of linear programming, where each module is optimized in turn [33], the developed algorithm allows simultaneous distribution of power over each module.
Features of renewable energy sources, this algorithm takes into account as follows. As for real-time wind parameters, which are stochastic in nature due to changes in wind speed and direction the power of the wind turbine at any time of the year should be calculated based on local weather conditions [31]:
where:
The instantaneous power of the wind turbine with a horizontal axis of rotation (using the example of an ideal wind wheel) can be determined by the formula
Here: η is the energy efficiency ratio (coefficient of performance) of the turbine; K is the wind energy utilization coefficient; ρ is the air density, kg/m3; D is the wind wheel diameter, m.
The power of the solar panel (module) is calculated by the expression:
Here:
According to the Eqs. (3)–(15) of the algorithm considered above, daily graphs of the dependence on the power of the solar and wind modules (Fig. 2) were built with an interval of T = 10 min.
Figure 2: Time dependence on the power of wind (1) and solar (2) modules
Unlike renewable sources, whose energy generation opportunities are sometimes limited by the climatic conditions instability, the uninterruptible power supply modules are usually used in microgrid systems to compensate for such types of energy shortages among consumers, as well as in other cases of damage and losses in the energy system [38].
3.2.3 Study of Steady-State Operating Modes
When solving the problem of optimizing the operating modes of microgrid systems, one of the determining factors is the amount of energy consumed. The use of actual measured hourly or every 15–30 min power consumption data provides sufficient information on the rate of change of load, including peak loads, necessary for the engineering analysis of such systems. As an example, Fig. 3 shows in the form of a step function the total daily consumer schedule (load curve), divided into T = 144 intervals of 10 min each.
Figure 3: Daily load curve in steady state operating mode
Taking into account the weather conditions on that day, optimization calculations were carried out under the criterion of minimum costs, according to the results of which the powers were distributed between the system modules at each time interval. The results of these calculations are shown in Fig. 4.
Figure 4: Power distribution among the modules in steady-state operating mode. I—solar module, II—uninterruptible module, III—wind module
The time distribution of the corresponding total costs, calculated in relative units, is shown in Fig. 5.
Figure 5: Optimal system costs in steady state operating mode
Analysis of the obtained results shows that at intervals 44–56 and 127–132 there is a sharp increase in total costs, since the maximum wind energy is taken to cover the load. In this case, there is a minimum consumption of solar energy, the inverter of the solar generator is underloaded and its efficiency is low. The maximum load power take-off is ensured with the maximum use of solar energy. The average total costs per day is
Note that the presented above algorithm, operating within the framework of the service-dominant logic, makes it possible to optimize the composition of the microgrid system and the rated powers of its modules to reduce costs without involving consumers to change their load schedules. For comparison, the costs were analyzed for a uniform distribution of power over three modules. In this case, the power of the solar module was equal to
3.2.4 Study of Transient Operating Modes
Transient processes in hybrid microgrid systems that occur with rapid changes in environmental conditions, as well as loads, as a rule, lead to corresponding structural and/or regime changes that reduce the stability limits of these systems. In these cases, a hybrid system can be considered sustainable if it is able to function normally under disturbance conditions, satisfying consumer demand in terms of the volume and quality of generated energy.
An excess level of such impacts can lead to the transition of hybrid microgrid systems to a state close to emergencies, when they operate at the limit of their capabilities. There are various scenarios for the development of events: an emergency shutdown of the load, which they are unable to provide, or the connection of an additional generator, in particular, an uninterruptible one. Herewith, the microgrid system can be characterized as stable if it is able to return from an emergency or extreme state to a normal one within a certain period of time limited by the norms.
The results of the simulation of transient processes in a hybrid microgrid system are shown in Figs. 6–8, where: Fig. 6 shows a load curve with four (1–4) different sections (types) of transients; the optimal distribution of power between modules is shown in Fig. 7 and the time distribution of the corresponding costs is shown in Fig. 8.
Figure 6: Load curve in transient operating mode
Figure 7: Power distribution among the modules in transient operating mode (I—uninterruptible module, II—wind module, III—solar module)
Figure 8: Optimal system costs in transient operating mode
As can be seen from Fig. 6, load change in time interval I causes a transient overshoot by 58%, which leads to a change in the operating mode of only the uninterruptible module, while solar and wind generation remains unchanged (Fig. 7). Transients in time intervals II and III caused minor fluctuations in the power of all modules, and the oscillatory transient process in the interval IV leads to an extreme power distribution over all modules, so the microgrid system is at the limit of stability here. Note, that the microgrid system costs during transients increase in each of the intervals I–IV (see Fig. 8). So, with a sharp change in load, it is calculated that costs increase of up to 2%.
Hybrid energy systems (HESs) and microgrids as their brightest representative are already today a decisive factor in the eco-transformation of the unified energy system (UES). It is also not difficult to predict the emergence of ‘nanogrid’ systems in the near future when each workplace will be equipped with a smart power system. At the same time, a number of problems facing the modern UES, primarily due to the rapid spread of RESs and the stochastic nature of the energy they generate, have already required significant structural and technological changes in the direction of its decentralization and greening based on specialized energy efficiency methods, energy management systems and information smart technologies.
Modern HESs generally cope with the tasks of providing end consumers with electrical and thermal energy, both in terms of volume and quality. Nevertheless, in the list of unresolved problems that these systems still need to untie, among others, we note the following, which are of the greatest importance in our study:
• From the organizational side—creation of management systems (service center) for coordination and balancing the energy needs of each specific energy community at the level of a household, an energy cooperative, a city, a country, a region, etc., in conditions of limited self-regenerating capabilities of their environment;
• From the methodological side—development of theory, software, tools and means that implement a cycle of continuous improvement of energy production and consumption processes, which include technological renewal, recycling and disposal of waste equipment and materials involved in this cycle.
To solve these problems we conducted a structural-component analysis and developed a three-level vertically organized model of performance management, where:
• Coordination-balancing service centers are located at the upper (first) level;
• Business entities providing different types of services occupy the next (second) level;
• Stages of the performance management are explored at the third level of the model.
To formalize this three-level model, symbolic mappings of set theory, represented by three mutually consistent systems of equations, were used at the first stage, which were then displayed in the form of balance equations (energy, economic, environmental) at the second stage and, finally, in the form of summation functions for solving optimization problems at the third stage of formalization. Of course, the considered method, model and procedures for their implementation are not the only ones of their kind, and the identified problems cannot be considered unambiguously resolved.
In general, the considered logic of the service-dominant (SD) implementation of HESs helps to open up new possibilities for solving optimization problems due to:
• Increase in the share of services aimed at providing end-users of energy with not so much quantitative as quality services in lighting, ventilation, heating and air conditioning, etc.;
• Development and implementation of virtually organized energy services management systems (centers) for decentralized and spatially distributed generation, as well as for its integration with centralized unified system;
• Active attraction of specialized energy services companies (ESCOs).
The presented results of engineering calculations of HES operation in steady and transient modes confirm that the developed optimization algorithm allows minimizing total costs, optimizing power take-off from HES modules, as well as transient parameters when switching consumer loads. So, in the calculation example, the average daily costs are reduced by 14.7% compared to the costs with a uniform distribution of power between the modules, although the costs due to transients can reduce this achievement by 2%.
In general, the proposed structural-and-functional framework for the transformation of modern energy systems, based on SD logic, allows researchers and engineers to more deeply and clearly identify problems and make a strategic decision to improve the results of the widespread introduction of renewable energy and energy efficiency measures in energy systems.
Further research in this direction will be aimed at expanding the scope of the discussed model, which makes it possible to carry out simulation calculations of HES operating modes, taking into account the possibilities of recycling and reuse of spent equipment and materials.
Funding Statement: The authors received no specific funding for this study.
Conflicts of Interest: The authors declare that they have no conflicts of interest to report regarding the present study.
References
1. Walker, C., Devine-Wright, P., Rohse, M., Gooding, L., Devine-Wright, H. et al. (2021). What is ‘local’ about smart local energy systems? Emerging stakeholder geographies of decentralized energy in the United Kingdom. Energy Research & Social Science, 80, 1–13. https://doi.org/10.1016/j.erss.2021.102182 [Google Scholar] [CrossRef]
2. Saki, R., Kianmehr, E., Rokrok, E., Doostizadeh, M., Khezri, R. et al. (2022). Interactive multi-level planning for energy management in clustered microgrids considering flexible demands. International Journal of Electrical Power and Energy Systems, 138, 107978. https://doi.org/10.1016/j.ijepes.2022.107978 [Google Scholar] [CrossRef]
3. Ahlqvist, V., Holmberg, P., Tangeras, T. (2022). A survey comparing centralized and decentralized electricity markets. Energy Strategy Reviews, 40, 1–14. https://doi.org/10.1016/j.esr.2022.100812 [Google Scholar] [CrossRef]
4. Dedecca, J. G., Opinska, L. G., Nuffel, L., Altmann, M. (2020). Study on energy costs, taxes and the impact of government interventions on investments in the energy sector. Rotterdam: Trinomics B.V. https://doi.org/10.2833/827631 [Google Scholar] [CrossRef]
5. Berrada, A., Mrabet, R. E. (2020). HES models. Cambridge: Academic Press. https://doi.org/10.1016/C2019-0-03501-7 [Google Scholar] [CrossRef]
6. Mohammed, O. H., Amirat, Y., Benbouzid, M. E. H., Feld, G. (2017). Optimal design and energy management of a hybrid power generation system based on Wind/Tidal/PV sources: Case study for the Ouessant French Island. In: Smart energy grid design for island countries, pp. 381–413. Basel: Springer International Publising. https://doi.org/10.1007/978-3-319-50197-0_12.hal-02969931 [Google Scholar] [CrossRef]
7. Ishaq, S., Khan, I., Rahman, S., Hussain, T., Iqbal, A. et al. (2022). A review on recent developments in control and optimization of micro grids. Energy Reports, 8(4), 4085–4103. https://doi.org/10.1016/j.egyr.2022.01.080 [Google Scholar] [CrossRef]
8. Hirscha, A., Paraga, Y., Guerrerob, J. (2018). Microgrids: A review of technologies, key drivers, and outstanding issues. Renewable and Sustainable Energy Reviews, 90, 402–411. https://doi.org/10.1016/j.rser.2018.03.040 [Google Scholar] [CrossRef]
9. Bower, W. I., Ton, D. T., Guttromson, R., Glover, S. F., Stamp, J. E. et al. (2014). The advanced microgrid. Integration and interoperability. USA: Sandia National Lab. https://doi.org/10.2172/1204100 [Google Scholar] [CrossRef]
10. Zhuikov, V. Y., Boiko, I. Y., Denysiuk, S. P. (2021). Model of dynamic tariffing Microgrid’s electricity consumption in local energy markets. Science and Education a New Dimension, IX(31)(250), 46–49. https://doi.org/10.31174/SEND-NT2021-250IX31-11 [Google Scholar] [CrossRef]
11. Melton, R. B. (2015). GridWise transactive energy framework. USA: Pacific Northwest National Laboratory. https://www.osti.gov/biblio/1123244 [Google Scholar]
12. Huang, Q., Amin, W., Umer, K., Gooi, H. B., Eddy, F. Y. S. et al. (2021). A review of transactive energy systems: Concept and implementation. Energy Reports, 7(3), 7804–7824. https://doi.org/10.1016/j.egyr.2021.05.037 [Google Scholar] [CrossRef]
13. Onumanyi, A. J., Sherrin, J. I., Kruger, C. P., Abu-Mahfouz, A. M. (2021). Transactive energy: State-of-the-art in control strategies, architectures, and simulators. IEEEE Access, 9, 131552–131573. https://doi.org/10.1109/ACCESS.2021.3115154 [Google Scholar] [CrossRef]
14. Khorasany, M., Azuatalam, D., Glasgow, R., Liebman, A., Razzaghi, R. (2020). Transactive energy market for energy management in microgrids: The monash microgrid case study. Energies, 13(8), 1–23. https://doi.org/10.3390/en13082010 [Google Scholar] [CrossRef]
15. Raddats, C., Kowalkowski, C., Benedettini, O., Burton, J., Gebauer, H. (2019). Servitization: A contemporary thematic review of four major research streams. Industrial Marketing Management, 83, 207–223. https://doi.org/10.1016/j.indmarman.2019.03.015 [Google Scholar] [CrossRef]
16. Vargo, S. L., Lusch, R. F. (2015). Institutions and axioms: An extension and update of service dominant logic. Journal of the Academy of Marketing Science, 44(1), 5–23. https://doi.org/10.1007/s11747-015-0456-3 [Google Scholar] [CrossRef]
17. Karpen, I. O., Bove, L. L., Lukas, B. A., Zyphur, M. J. (2015). Service-dominant orientation: Measurement and impact on performance outcomes. Journal of Retailing, 91(1), 89–108. https://doi.org/10.1016/j.jretai.2014.10.002 [Google Scholar] [CrossRef]
18. Lusch, R. F., Nambisan, S. (2015). Service innovation: A service-dominant logic perspective. MIS Quarterly, 39(1), 155–175. https://doi.org/10.25300/MISQ/2015/39.1.07 [Google Scholar] [CrossRef]
19. Tukker, A. (2015). Product services for a resource-efficient and circular economy—A review. Journal of Cleaner Production, 97(3), 76–91. https://doi.org/10.1016/j.jclepro.2013.11.049 [Google Scholar] [CrossRef]
20. Eutukhova, T., Kovalko, O., Novoseltsev, O., Woodroof, E. (2020). Energy services: A proposed framework to improve results. Energy Engineering, 117(3), 99–110. https://doi.org/10.32604/EE.2020.010864 [Google Scholar] [CrossRef]
21. Kovalko, O., Eutukhova, T., Novoseltsev, O. (2022). Energy-related services as a business: Eco-transformation logic to support the low-carbon transition. Energy Engineering, 119(1), 103–121. https://doi.org/10.32604/EE.2022.017709 [Google Scholar] [CrossRef]
22. Martinez, V., Bastl, M., Kingston, J., Evans, S. (2010). Challenges in transforming manufacturing organizations into product-service providers. Journal of Manufacturing Technology Management, 21(4), 449–469. https://doi.org/10.1108/17410381011046571 [Google Scholar] [CrossRef]
23. Chupryna, L., Kovalko, O., Novoseltsev, O., Woodroof, E. (2020). Virtual organization of energy management: Service-oriented framework to improve results. International Journal of Energy Management, 2, 47–63. https://www.researchgate.net/publication/356980815 [Google Scholar]
24. Poslad, S. (2009). Ubiquitous computing smart devices, environments and interactions. England: Wiley. https://www.wiley.com/en-us/Ubiquitous+Computing:+Smart+Devices,+Environments+and+Interactions-p-9780470035603 [Google Scholar]
25. Kovalko, O. M., Kovalko, N. M., Novoseltsev, O. V. (2018). Result-oriented investment management system for targeted energy efficiency programs. Scientific Bulletin of National Mining University, 3(165), 160–166. https://doi.org/10.29202/nvngu/2018-3/20 [Google Scholar] [CrossRef]
26. Hansen, S. J., Bertoldi, P., Langlois, P. (2009). ESCOs around the world: Lessons learned in 49 countries. USA: The Fairmont Press. https://doi.org/10.1201/9781003151494 [Google Scholar] [CrossRef]
27. Stuart, E., Larsen, P. H., Carvallo, J. P., Goldman, C. A., Gilligan, D. (2016). U.S. Energy Service Company (ESCO) industry: Recent market trends. USA: Ernest Orlando Lawrence Berkeley National Laboratory. https://emp.lbl.gov/publications/us-energy-service-company-esco [Google Scholar]
28. Boza-Kiss, B., Bertoldi, P., Economidou, M. (2017). Energy service companies in the EU—Status review and recommendations for further market development with a focus on energy performance contracting. Luxembourg: Publications Office of the European Union. https://doi.org/10.2760/12258 [Google Scholar] [CrossRef]
29. Kovalko, O. M., Yevtukhova, T. O. (2010). Set-theoretic model of the hierarchical multilevel organizing-and-technological energy management system. POWER ENGINEERING: Economics, Technique, Ecology, 42–49. https://doi.org/10.20535/1813-5420.2.2010.178273 [Google Scholar] [CrossRef]
30. Deshko, V. I., Kovalko, O. M., Novoseltsev, O. V., Yevtukhova, M. Y. (2020). Energy services market: Conceptual framework and mechanism of forming. International Journal of Civil, Mechanical and Energy Science, 6(6), 48–55. https://doi.org/10.22161/ijcmes.66.4 [Google Scholar] [CrossRef]
31. Suhane, P., Rangnekar, S., Mittal, A., Khare, A. (2016). Sizing and performance analysis of standalone wind-PV based hybrid energy system using ant colony optimization. IET Renewable Power Generation, 10(7), 964–971. https://doi.org/10.1049/iet-rpg.2015.0394 [Google Scholar] [CrossRef]
32. Chaturvedi, N. (2021). Cost-optimal pinch analysis for sizing of hybrid power systems. Cleaner Engineering and Technology, 3, 100094. https://doi.org/10.1016/j.clet.2021.100094 [Google Scholar] [CrossRef]
33. Shufian, A., Mohammad, N. (2022). Modeling and analysis of cost-effective energy management for integrated microgrids. Cleaner Engineering and Technology, 8, 100508. https://doi.org/10.1016/j.clet.2022.100508 [Google Scholar] [CrossRef]
34. Suresh, V., Muralidhar, M., Kiranmayi, R. (2020). Modeling and optimization of an off-grid hybrid renewable energy system for electrification in a rural areas. Energy Reports, 6, 594–604. https://doi.org/10.1016/j.egyr.2020.01.013 [Google Scholar] [CrossRef]
35. Chen, J., Garcia, H. E. (2016). Economic optimization of operations for HESs under variable markets. Applied Energy, 177(11), 11–24. https://doi.org/10.1016/j.apenergy.2016.05.056 [Google Scholar] [CrossRef]
36. Rehman, S., Natrajan, N., Mohandes, M., Alhems, L. M., Himri, Y. et al. (2020). Feasibility study of hybrid power systems for remote dwellings in Tamil Nadu, India. IEEE Access, 8, 143881–143890. https://doi.org/10.1109/ACCESS.2020.3014164 [Google Scholar] [CrossRef]
37. Arrar, S., Xioaning, L. (2022). Energy management in hybrid microgrid using artificial neural network, PID, and fuzzy logic controllers. European Journal of Electrical Engineering and Computer Science, 6(2), 38–47. https://doi.org/10.24018/ejece.2022.6.2.414 [Google Scholar] [CrossRef]
38. Rehman, S. (2021). Hybrid power systems-sizes, efficiencies, and economics. Energy Exploration & Exploitation, 39(1), 3–43. https://doi.org/10.1177/0144598720965022 [Google Scholar] [CrossRef]
Cite This Article
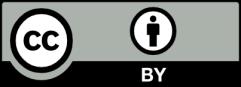
This work is licensed under a Creative Commons Attribution 4.0 International License , which permits unrestricted use, distribution, and reproduction in any medium, provided the original work is properly cited.