Open Access
ARTICLE
Management of Peak Loads in an Emerging Electricity Market
1 Laboratoire de Génie Electrique, Mécatronique et Traitement du Signal, ENSPY, Université de Yaoundé I, Yaounde, Cameroun
2 Department of Electrical and Electronic Engineering, National Higher Polytechnic Institute (NAHPI), University of Bamenda, Bamenda, Cameroon
3 Department of Electrical and Power Engineering, Higher Technical Teacher Training College (HTTTC), University of Bamenda, Bamenda, Cameroon
4 Mekin Hydroelectric Development Corporation, Meyomessala, Cameroon
5 ENEO Cameroun S.A., Douala, Cameroon
* Corresponding Author: Edwin N. Mbinkar. Email:
Energy Engineering 2022, 119(6), 2637-2654. https://doi.org/10.32604/ee.2022.023419
Received 25 April 2022; Accepted 18 July 2022; Issue published 14 September 2022
Abstract
An electricity market is a trading platform provided by the actors in the electricity sector to sell and buy electricity while maintaining the stability of the transmission network and minimizing energy losses. The management of electrical energy for rational use consists of all the operations that the consumers can carry out in order to minimize their electricity bill, while the producers optimize their benefits and the transmission infrastructure. The reduction of active and reactive power consumption and the smoothing of daily and yearly load profiles are the main objectives in this work. Many developed countries already have properly functioning electricity markets, but developing countries are still in their infancy of deregulated electricity markets. The major tools used in smoothing the load profiles include decentralized generation, energy storage and demand response. A load power smoothing control strategy is proposed to smooth the load power fluctuations of the distribution network. The required power change is determined by evaluating the power fluctuation rate of the load, and then the required power change is allocated to some generators or to some stored reserves. Otherwise, the consumers are made to curtail their power consumption. The ideas proposed in this work provide important opportunities for energy policy makers and regulators. These ideas would only be feasible if there exists real-time communication among the actors in the electricity market. The results indicate that as much as 1100 Megawatt-hours of energy can be stored for smoothing the load profile, when applied to the Southern Interconnected Grid of the Cameroon power system; and that Time of Use (TOU) pricing could be used instead of rotating blackouts in case of energy shortage.Graphic Abstract
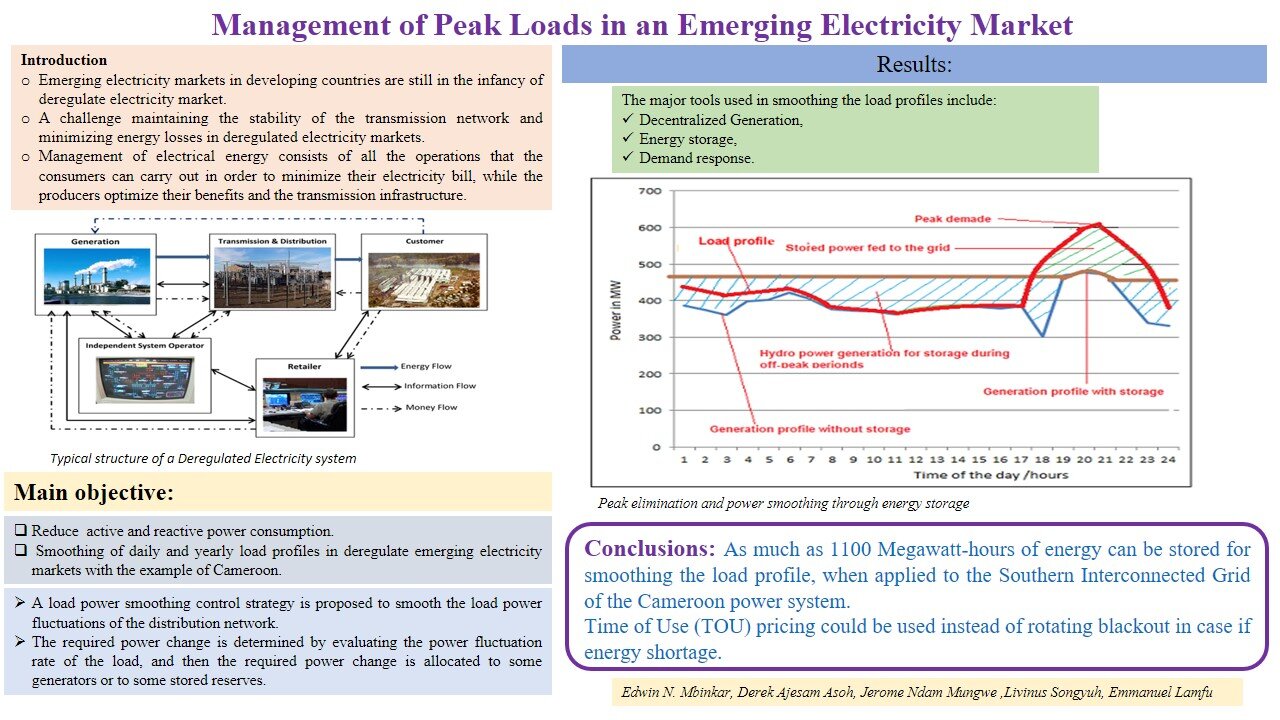
Keywords
Nomenclature
DG | Distributed Generation |
DR | Demand Response |
ISO | Independent System Operator |
PV | Photovoltaic System |
PSH | Pumped Storage Hydro |
SIG | Southern Interconnected Grid of the Cameroon Power System |
SONATREL | National Electricity Transmission Company |
TSO | Transmission System Operator |
This paper looks at the strategies for saving electrical energy for use during peak periods. When the peak demand for electrical energy exceeds the total installed capacities of all generators connected to the grid, in order to maintain grid stability, the system operator must proceed to load shedding. Peak load management strategies range from low cost operation through the use of energy efficient equipment to the installation of capital intensive projects like battery storage and new generation units.
The use of renewable energy to supply electricity power grids requires that the electricity market must undergo some changes in order to cope with the intermittent nature of these resources. The major control problem in electricity systems, is to balance demand and supply at all times. Due to the need for the increasing grid connection of renewable energy resources, it is incumbent to compensate for fluctuations in both demand and supply. For this balance to be maintained, electricity market operators frequently rely on decentralized generation, energy storage, and demand response.
Decentralized Generation (DG) is opposed to conventional generation, by large power generation plants that are connected to the High Voltage (HV) grid, whose location and power are centrally controlled to participate in the control of frequency and voltage, and to ensure reliable and economical operation of the entire operation of the whole network. DG generation is developing in most developing countries, based on renewable energy or traditional production units, installed by independent producers [1]. Technologies using renewable energies are numerous and well-known, which makes them to be of great economic and environmental benefit. The main technologies are photovoltaic, small thermal plants, mini hydroelectricity plants, wind turbines and biomass [2].
The increasing dependence on DG for supply of electrical energy to the electricity grid has caused the consumers to become producers of electricity themselves. The current digitization in the electricity sector such as the installation of smart meters means that customers can reduce their overall monthly bill by choosing to consume electricity during low-tariff periods rather than during high-tariff periods, even if the total amount of energy used is the same [3]. Most of the generators at customer sites are usually fossil fuel generators that are not meant to run for days. These can be used as peak-load plants if they are connected to the grid. Peak-load power plants are designed to operate only when the demand is very high. However, they are generally smaller and less efficient than base load plants, and they run on more expensive fuels such as natural gas or diesel.
Due to difficulties in storing electrical energy unlike gas or water, there is a unique problem faced by electric utilities all over the world. There is need to generate, transmit, and distribute electrical energy as demanded. Electric utilities face high demand peaks in the evenings as people leave their workplaces and go back home, tuning on the air conditioners, televisions, lights, and other electrical appliances. At night, when most people are sleeping, there is of course a significant reduction in the demand for electricity. Typically, such times of the day and the year are called “on-peak” and “off-peak,” with times in between referred to as “partial peak” or “medium peak” [4].
Demand Response (DR) technologies provide another tool to manage grid stability when DG is grid-connected. Traditionally, grid management has always been focused on supply management. However, there are new technologies, such as smart grids and smart metering that allow for real-time monitoring and communication between producers and consumers of electricity to optimize grid usage. With DG, many consumers of electricity can at times be producers of electricity, selling their surplus generation capacity to the network operator.
Implementing real-time control systems in electricity networks can greatly facilitate grid management and the building of a truly decentralized energy system [5]. Real time control systems make the operation of the network more robust and automatically responding to changing demand by adjusting the outputs from different generating units appropriately, and ensuring the availability of the transmission infrastructure.
The integration of these decentralized sources of electricity production, whose position is not only random but also seldom predictable, has impacts on the network. The presence of DGs in the distribution system usually changes the voltage profile and it is necessary to check to ensure that the voltage remains within its contractual range at all DG connection points. This can also cause local active and reactive powers to vary from those coming from the upstream network, which can cause the voltage to rise, especially on the branch where it is connected. This insertion may cause overvoltage if the power produced by the DG is very high compared to that in the upstream network due to short-circuit impedance [5].
The key constraints facing the electricity supply companies in some developing countries like Cameroon relate to the little generation capacity, the geography of the country and relative obsolescence of the transmission and distribution equipment. Consequently, there is usually a significant unmet solvent demand. This situation is exacerbated by the fact that the country’s three main transmission grids are completely isolated from one another and no exchange of available surpluses can be made between the grids. Some of such countries run several thermal power plants, which are significantly more costly to operate than hydro plants because they are peak load plants. It would make economic sense for the utility to avoid using the more expensive power plants, if there is no penalty for this, especially as the regulated selling price is always below the operating cost of these units.
2 Decentralized Electricity Generation
In many developing countries, the shortfall in electricity generation capacity is being aggravated by load growth, transmission line constrains, limitations in the ability to construct large scale generation plants due to environmental policy restrictions, and lack of appropriate technology to meet new requirements. The number of Independent Power Producers (IPPs) connected to the distribution network is on the increase in many countries. Due to reliability concerns, there is renewed interest in the development of energy sources that have remained unexploited previously such as biomass, micro-hydro systems, wind turbines and solar energy. The capacity of most of these sources seldom exceed a few hundreds of kW. The electrical power generation infrastructures in most developing countries are relatively old and outdated, lack proper maintenance, improper relationship between the various stakeholders in the energy sector, and so contribute to the shortfall in electricity supply [6].
The advantages of DG are very important for both suppliers and consumers. For the former, it turns out that this means of production can be installed close to the consumer, leading to a decrease in transportation and distribution costs, and sometimes less electrical losses. The geographic locations for small generators are easier to find, and the installation times are also considerably short. However, the massive installation of DG will also greatly modify energy flows in the networks. There will be a possibility of power flow reversal on the distribution network, which brings into question its architecture, which was initially designed exclusively for a tree-structured distribution of energy from the transmission or distribution networks [7]. Consequently, given the advances in DG and related controls, the idea of building huge power stations and then investing in hundreds of miles of transmission and distribution lines to deliver the power to the markets is a proposition that many developing countries are beginning to find difficult to justify economically.
Wind energy is converted into electrical energy by means of wind turbines. One interesting characteristic of wind power is the fact that power produced is proportional to the cube of the wind speed [8]. That is, if the wind speed is doubled, the power generated will increase by a factor of eight. This makes it easy to understand why wind turbines should be placed in windy locations. Clearly, wind energy potential depends strongly on the wind speed, which is a necessary condition, not an option.
The available power also depends directly on the area through which the wind passes. The power generated is generally considered to be proportional to the area swept by the rotor of a wind turbine, which also depends on the radius of its rotor. Wind power is considered as free energy with no fuel costs. Wind power is also considered renewable energy, since wind actually never goes away. The available wind power is calculated as shown in Eq. (1).
This power increases with the cube of the wind speed (V), the area of the rotor being swept by the wind (A) and the air density (ρ). For a rated power of 7.0 MW corresponding to an annual wind speed of 11 m/s, the rotor diameter is about 164 m with a sweep area of 21,124 m2 [9]. Wind generators are designed as induction electrical machines because of their variable speeds of rotation and the necessity for them to have light weight since they are always mounted on poles.
Several strategies can be used to control the performance of wind turbines depending on the design. There are two control objectives; speed control and power limiting, which need to be addressed. In order to address these objectives, it is necessary that the controller relieves the stress throughout the wind turbine, filters the generated power and maximizes energy capture. In a typical wind generator, there is a drive train that has a gearbox, and the controller clearly avoids any resonant frequencies [10]. Most wind turbines are used to produce electricity which is consumed locally especially in isolated sites, or injected into the electrical grid. The electricity generated is fed directly into the electrical grid or to wind power storage batteries.
Photovoltaic (PV) modules convert the sun’s energy into electricity using semiconductor technology. The intensity of the incoming solar radiation at any point on the earth’s surface is determined by a multitude of factors. These include the position of the sun in the sky, which is determined by the time of day, the latitude, season, atmospheric pollution, and the height above sea level at which the measurement is taken. For PV modules to work in a photovoltaic system connected to the grid, several modules are needed, connected to each other. There are two ways to connect them: in series or in parallel. These two options are quite different, and are used one or the other depending on the needs. When PV modules are connected in series, the voltages are added together, while maintaining the same amperage. This is normally done between modules of the same amperage. If not, the array is aligned with the lowest amperage [11]. When modules are connected in parallel, the currents are added together, while the voltage remains the same. This is normally done between modules of the same voltage, in order to avoid any risk of overvoltage or short-circuit [12]. The currents can be different, since they add up.
PV systems more often than not, use energy storage devices to store the energy generated. However, grid-connected PV installations in an interconnected grid can be done without any particular need for storage. The grid ensures the supply to the user in case of a shortfall in PV production. The surplus can be supplied to the grid and bought back by the utility company. This integration can be done at the level of the distribution lines or the low voltage network. Therefore, the electric utilities will from time to time modify some of their transmission infrastructure like wire sizes and voltage control settings in order to cope with the new power flow patterns [12].
When there is excess PV production, it is then stored in batteries. In this case, the power inverter is remotely controlled by a controller that regulates the production according to the capacity of the distribution lines. The storage used in most cases consists of lead-acid batteries because they are very suited for low capacity storage and daily use. For the daily variations between day and night and seasonal variations, meteorology has a strong impact on productivity, considering the influence of cloud cover or temperature variations. From the consumer's point of view, in order to make up for this intermittency, a means for reversible storage must be added to these sources. Thus, the surplus of energy during the day can be stored to be used when there is a lack [13].
Small hydroelectricity systems have proven their worth in isolated grids or as stand-alone systems. A small hydroelectricity system is defined as an energy production facility with a capacity of less than 10,000 kW that converts hydraulic energy from a river into electrical energy. They are classified according to the installed capacity such as; small hydro system for a power between 2,000 and 10,000 kW; mini hydro system for a power between 500 and 2,000 kW; micro hydro system for a capacity between 20 and 500 kW; and a pico hydro system for a capacity of less than 20 kW [14]. The two most important factors in the energy generation available are the potential head and the water flow rate, which depend on the site and must be studied beforehand.
To boost the production and consumption of renewable energies and reinforce electricity networks, electricity storage has become an essential tool. Electricity per se cannot be stored, at least not with current technologies. In reality, electricity storage consists of converting an electric current into another form of storable energy. This can then be mobilized on demand, a few minutes or hours or even days later, to produce electricity again. Therefore, electricity storage poses a unique problem for electric utilities the world over as there is the need to generate, transmit, and distribute the energy as it is demanded in real-time.
Electricity supply companies usually face significant demand peaks at certain times of the day or year. During the peak periods, the utility companies generate electricity from expensive sources such as fuel and gas fired plants to meet up with the demand. Other renewable sources like photovoltaic and wind turbines can also be used. But, unfortunately, these sources are complicated by the fact that they have low power densities and are subject to both systematic and random variations in their availabilities.
As a result of very high costs of electricity during the on-peak periods, utility companies are developing new technologies to attack these high-priced peaks; including demand response, hybrid generation, and energy storage for peak shifting. Various types of energy storage systems now exist to shift power to peak times and also offer ancillary services, even though they are faced with the major weaknesses of renewables, namely intermittency. For instance, wind blows primarily at night in most tropical countries and is also variable in speed from minute to minute, so coupling storage to wind generators can redress a lot of these issues. In the case of photovoltaic generation, the sun shines only during the day, so to consume this form of electricity generation in the night, it must be coupled with a storage system.
The main objective envisaged in electrical energy storage is to enable renewable energies to deploy their full efficiency potential to meet the electricity and energy needs of the electricity consumers. From this point of view, the aim is to avoid losing the electricity produced when production exceeds demand. Conversely, it is a question of ensuring the continuity of supply when some of the generation equipment are not producing power [15]. Therefore, the objectives of storage are to rationalize the use of intermittent generation; to ensure the demand/supply balance and contribute to the stability of the electricity network; to ensure high reliability for renewable resources operating off-grid; and to give renewable energies a more important place in the electricity mix.
The energy storage capacity can be estimation using numerical integration techniques such as the trapezium rule. The trapezium rule is a way of estimating the area under a curve. If we want to find the area under a curve between the points x0 and xn, we divide this interval up into smaller intervals, each of which has length h as shown in Fig. 1. The trapezium rule works by splitting the area under a curve into a number of trapeziums of known area,
Figure 1: Illustration of trapezium rule
Then we find that:
where
There are several battery technologies that have been developed for storing electrical energy, but only a few of them have been used in the field. The batteries are electrochemical systems, which store energy in chemical form and release it in electrical form. Technologies that have been proven to be cost effective in the field include lead-acid, nickel/cadmium, sodium/sulfur, and vanadium-redox flow batteries [16]. Other battery technologies, such as the many lithium-ion batteries, are less matured and not yet well-developed for these applications [17]. Batteries are used for massive energy storage, but can deliver power only for a few hours or days. They can withstand a number of charge/discharge cycles.
Grid connected electricity storage systems are increasingly attracting the attention of utility companies and decision makers [18]. The main reasons that have led to this great interest include the growing proportion of intermittent renewable energy sources in electricity generation, the high costs of the infrastructure needed for improvement in the reliability of power systems, as well as the significant costs associated with managing peak electricity demand. The perceived benefits of electricity storage systems have led to the development of new storage technologies, demonstration projects, and research projects that quantify the benefits of storage systems.
When a lead-acid battery is in use, it is subject to discharge. The discharge reaction can be summarized by the chemical processes depicted by Eq. (3).
This means that both the lead dioxide of the positive plate and the sponge lead of the negative plate react with the sulphuric acid to form lead sulphate and water. This reaction is electronically 100% efficient [18]. The recharge process which is the reverse is depicted in Eq. (4).
The efficiency may be 100% depending on the charge of the battery. When the efficiency of the recharge reaction is less than 100%, then gassing or electrolysis of water takes place [12]. In the context of DG, electricity storage has a key role to play. In addition to its usefulness in compensating for variations in electricity generation, it makes it possible to adapt to demand by injecting previously stored energy into the network at any time.
3.2 Pumped Storage Hydro (PSH)
In pumped storage plants, water is pumped from a lower reservoir or river to an upper reservoir during off-peak hours, using the unsupplied electricity generated, and leading to the leveling out of the daily generated power. PSH is intended for operation at the level of the grid or intermittent production systems such as wind and PV energy. The water between the two reservoirs at different heights (difference in height h) is either pumped or drives turbines (Fig. 2). The stored energy W (in J) is calculated by Eq. (5).
Figure 2: Hydroelectric dam cross-section with PSH [21]
where m is the mass of water (in kg) and g = 9.81 m/s2 is the acceleration due to gravity.
PSH is the most widespread of the large-scale storage facilities with 90 GW produced in the world annually, i.e., 3% of the global production capacity [19]. For example, the Grand-Maison dam in France has a 935 m drop, a 170,000,000 m3 water reservoir and can produce a power output of about 1800 MW.
The operation of large pumped storage plants offers a unique opportunity for massive energy storage [20]. Its advantages include the energy performance (high overall conversion efficiency) as well as the investment cost (long life and mature technology) and operating cost (operating and maintenance costs, and reduced failure rates). PSH thus offers the possibility to store surplus electrical energy and to respond reactively and with large capacities to supply and demand variability. Massive storage technologies are able to inflect the fatal and intermittent nature of renewable energy resources over significant periods of time, with a strong capacity to adapt to market needs by decoupling energy production and consumption. Fig. 2 illustrates the cross-section of a hydroelectric dam with PSH, which has been adapted from [21].
It is difficult to evaluate the storage needs of PSH because these needs depend on several factors such the proliferation of intermittent sources in different geographical locations which are therefore subject to seasonal variations; and the evolution of consumption patterns which depend on the capacity of consumers to adapt to generation capacities and other factors influencing the electricity market.
When DG is grid-connected, Demand Response (DR) can be a useful tool for managing grid stability. The strategies used to implement DR can be classified as either active or passive, or as direct and indirect. Active strategies require the consumers of electrical energy to play a significant role in the determination of the programs in which they can participate and the level of their participation. Examples of such direct strategies may include time-of-use rate plans and programmed load shedding. For passive demand response programs, the electricity consumers have very little or no control over it. The power supply company applies their intended solution without informing the customer, and the consumers have no choice than to cope with the program being implemented. Some examples of passive demand response techniques include rolling blackouts and voltage reduction.
DR techniques can also be direct or indirect. Direct approaches to DR result in quantifiable outcomes that directly affect the demand for electricity [22]. It is possible to relate the action to the reaction. Indirect DR involves influencing the consumers to behave differently, such as indicating the amount of energy currently being consumed and providing an incentive to reduce consumption and showing how such a change could positively affect the electricity bill at the end of the month. In this case, it is difficult to directly relate the action to the reaction. Short DR programs encourage end users to reduce their electricity consumption when prices are high or when the grid is approaching overload or instability. There are different business models that can be used to attain DR. Typically, the capacity factor of DR solutions is quite low as consumers are only willing to curtail their demand for a limited period of time, in the face of extreme prices [23].
Electricity peaks must be controlled to avoid stressing the electrical network. The peak demand or peak electricity consumption corresponds to the periods during which the demand for electricity is the highest. Peak loads vary by season and time of day (as well as by region of the world, but attention is focused on emerging electricity markets such as in some Sub Saharan African countries). We can therefore distinguish between daily peaks and seasonal peaks.
The daily peak is the time of the day when electricity demand (and therefore consumption) is highest. It is generally more pronounced on weekdays. This can be explained by behavior and lifestyles, which influence the use of electrical appliances and installations. In Cameroon, for example, this daily peak occurs usually from about 6 pm to 10 pm. In the southern interconnected grid of the Cameroon power system (SIG), these daily peaks have been steadily increasing for several years. This is explained by an overall increase in electricity consumption due to increase in demography and industrial development [4].
The seasonal peak is the time of year when electricity consumption is the highest. In Cameroon, there is actually no identifiable seasonal peak. However, due to the high dependence of the Cameroon power system on renewable energy resources such as hydroelectricity, there are periods of the year when electricity generation is very low; and the total available generation capacity cannot meet the demand from consumers, even during the daily off-peak periods. These periods of low electricity production correspond to the dry season when there is very little or no rainfall.
Operations and maintenance costs vary widely between different forms of power generation but form an important part of any power plant's business. The peak load plants are very expensive to run, and so can be run only for few hours in a day. For a given power plant with a certain installed capacity in MW, the cost is divided into fixed cost and variable cost. The cost of land and the needed machinery for example do not necessarily depend on the output of the plant. However, the cost of fuel is variable depending on the output of the plant. Therefore, the cost function of a generating plant is a function of the output of the plant in MW.
The average cost of operating the plant is given by the cost per MW of the plant [24]. Thus the average operating cost of the plant can be determined as in Eq. (7). CP, CF and CV are respectively the cost function of the plant, the fixed cost and the variable cost.
The operating cost required to produce one MWh of electrical energy is called the marginal cost [25]. Peak load plants are generally fossil-fired power plants in which fuel costs dominate, and they can be started very fast from the cold. For renewable energy power plants, fuel is generally free and base load plants are generally of this type, in many developing countries. Table 1 shows typical ranges for capital (fixed) and operating (variable) costs for power plants.
5.1 Techniques for Managing the Peaks
The management of electric energy during the peaks is usually to ensure the efficient use of energy by the consumers in order to reduce or minimize their electricity bills. The techniques used involve the reduction in the consumption of active power and reactive power. The utility company encourages the optimal use of generation and transmission infrastructure. Since investment in new infrastructure is difficult to come by, proper management of the peaks can delay the need for the construction of some peak load plants. DR is a novel technique for managing the peaks. There are two main strategies to do this, that are applicable to emerging electricity markets; the first involves giving incentives such as payments or reduced prices to customers who eliminate or minimize their consumption when requested by the Transmission System Operator (TSO). The second strategy is based on the reaction of customers to changes in prices of electricity over time. However, the two techniques are so interwoven in such a way that most DR techniques make use of elements of both.
Consumers are made to understand that the curtailment of their maximum load, will result in a direct reduction of the power costs. This can be done by optimization of the consumption patterns and smoothing of the load curve over time; or by shifting some of the load from its own peaks to the troughs. Depending on the sector, significant savings of power costs can be achieved by avoiding consumption during peak hours. Time of Use (TOU) pricing is a means of making electricity market pricing and hence making demand and supply more rational and efficient [24]. TOU rate structures encourage energy consumers to shift energy use to less costly partial-peak and off-peak hours. The load information that is needed mostly by the utility company is how the consumers use electric energy at different hours of the day, different days of the week and seasons of the year and the utility's total load. The determination of customer’s hourly load from statistics of load measurements is very important in fixing the TOU rates and determining the amount of energy that is needed to satisfy the peak demand.
Energy storage techniques like PSH can be used to cope with peak loads in the electricity system. Fig. 3 shows the electricity generation within the SIG for a Monday in April (towards the end of the dry season), which is a working day. The peak period is from 8 pm to 9 pm when the total power generated is about 600 MW, with 470 and 130 MW coming from the hydro plants and thermal plants respectively. This corresponds to the typical power generation for a working day at the peak of the dry season when thermal plants are operated almost at their limits. Fig. 4 shows the typical generation for a Saturday in April. Although the peak power is almost the same as that of a working day, the contribution from the thermal plants at the peak is less. In the rainy season, hydro production increases remarkably as shown in Figs. 5 and 6 corresponding to a Wednesday and a Sunday, respectively. On Wednesday, the peak power is about 670 MW and the contribution of the thermal plants is just about 70 MW at the peak period. On Sunday, the maximum production falls below 600 MW and thermal plants are almost absent; because the system can supply all the customers using only the hydro plants.
Figure 3: Typical SIG power production on Monday in April
Figure 4: Typical SIG power production in MW for a Saturday in April
Figure 5: Typical SIG power production in MW for a Wednesday in July
Figure 6: Typical SIG power production in MW for a Sunday in July
The data used in drawing the graphs is obtained from AES SONEL achieved data in a previous work [5]. The data for each day is averaged for four consecutive weeks of the same month. The generation is from two hydroelectric plants in Edea and Songloulou (264 and 384 MW installed capacities respectively) and two thermal peak-load plants in Limbe (85 MW) and Dibamba (88 MW). Fig. 7 illustrates how power smoothing is achieved. It makes use of data obtained from the SIG, corresponding to Fig. 3 for illustration. This technique can eliminate the need for backup thermal power plants to operate during peak periods, resulting in better utilization of base load power plants and a dramatic reduction in greenhouse gas (GHG) emissions [26]. Applying the trapezium rule to Fig. 7 to estimate the area enclosed between the hydro-generation profile and the smoothened load profile, it is seen that approximately 1100 MWh of equivalent energy can be stored in the case of PSH.
Figure 7: Peak elimination and power smoothing through energy storage
The combination of DG, energy storage and DR leads to the optimization of operations in deregulated electricity market systems, both from a technical and economic point of view. These solutions, even if they are very complex compared to other solutions such as installation of peak load thermal plants, are clearly of great interest because of their incomparable flexibility, their operating flexibility and their cost price.
5.2 Structure of Emerging Electricity Markets
Today, it is possible to vertically separate the activities of electricity companies into separate units, namely generation, transmission and distribution. Theoretically, there can be competition within each of these activities. However, analysts believe that, at present, competition can realistically be said to exist only in the production segment [27]. Currently, transactions in the electricity generation market take two forms: the bilateral contracts (market) between suppliers and consumers and the auction or spot market in which generators submit bids to a centralized agent which determines the winning bids and the winning price [27].
In order for there to be fair competition in the generation sector, and for trading to take place, potential generators must be able to deliver electricity to consumers. This requires non-discriminatory access to transmission networks, which are generally monopolies. The determination of the modalities of access to these networks (prices and technological aspects) is the responsibility of the regulator, while the management of access to the networks is the responsibility of a new entity called the independent system operator or ISO, which must be independent of the owners of the generation equipment, in order not to be in a conflict of interest situation, which would be detrimental to competition.
From the economic standpoint, electricity must be produced when demand arises on the grid, which must therefore have access at all times to sufficient power to meet the demand. A power system must therefore operate and be managed as an integrated system. To ensure the physical integrity of the system, the balance between supply and demand, or between generation and distribution, must be maintained at all times [27]. There is no link between the energy supplied by a given generator and the energy consumed by a given appliance. The grid acts as a kind of an aggregation tool for electricity supplied (supply) and distributes the electricity to the load centers (demand). If issues of reliability arise in the transmission system, leading to congestion in the transmission lines, congestion management is required [28].
A number of market structures may emerge around the world, but one fact that seems always to be true is that transmission and generation services have to be separated from one another [29]. The market for generation is more competitive, with many market participants that are able to sell their energy. Meanwhile, the operation of the transmission system is usually a regulated monopoly whose function is to allow open, non-discriminatory and comparable access to all suppliers and consumers of electrical energy. This function is usually implemented by the ISO [30] as shown in Fig. 8.
Figure 8: The typical structure of a deregulated electricity system [5]
In Fig. 8, the typical structure of a deregulated electricity system with links of information and money flow between various actors is presented. Deregulation or restructuring of the electricity market is the removal of control over the prices with introduction of market actors or players in the sector. Deregulation of the power industry has also let to changes in power generation technology, with most power plants built nearer to the load centers. The configuration of the electricity market shown in Fig. 8 may not be universal, as each country may have its own peculiarities [31]. The different power producers deliver their product to their customers over a common set of transmission and distribution facilities. These operations are supervised by the ISO. The generators, transmission and distribution companies with the ISO communicate among themselves and money changes hands. Consumers usually communicate with retailers with the aim of knowing the energy price [32].
The management of peak load represents a major challenge in grid management and control of flow of electrical energy from generators to the consumers. Demand response, decentralized generation through the use of renewable energy and energy storage are the best fit candidates for maintaining grid stability and customer satisfaction during periods of peak electricity demand. Looking from a utility’s standpoint, changes in demand are the best way to manage system stability issues necessitating a balance between the power generated and the power consumed by the loads. However, in the case of industrial or commercial consumers, they represent a major complication in market operations and environmental concerns.
For the case of Cameroon, the transmission network operator SONATREL publishes a weekly program of blackouts, throughout the areas supplied by the SIG, in an attempt to curtail demand from both industry and households, as a means of managing the peaks in demand. However, the imposition of this blanket method of load shedding has often lead to many legal issues with industrial consumers and some households. In the face of these challenges, it would be preferable to apply TOU pricing for electrical energy as a means of managing the peaks in electricity demand. In the past, the main generation company had often blamed these frequent blackouts on the level of water in the reservoir dams (because they would not generate energy from costly thermal plants and supply to consumers at the regulated prices). This is when PSH deserves some consideration in the SIG.
A good number of developing countries such as Cameroon are showing interest in the development of wholesale electricity markets. Monopoly has in the past characterized the electricity supply companies in most of these countries. Consequently, the governments, or their regulators, have to set rules of behavior (pricing, service quality, etc.) to protect consumers and promote industrial and social objectives. To achieve these objectives, the regulatory agency can intervene at two levels: regulation of the utility's internal activities and regulation of market activities. The development and expansion of emerging electricity markets will also require certain paradigm shifts. Some of these might include the following:
- Ensure the economic viability of the public utility companies. This is consistent with the government's responsibilities, because of the importance of electricity in the social life of a country and the consequences of the bankruptcy of an electricity company. The generation companies must be able to increase their installed generation capacities as demand for electricity increases, so that blackouts should seize to be used as a tool for managing the peaks.
- Exclude households and small & medium size enterprises from TOU pricing of electrical energy and from the necessity to change their metering equipment when there is a change of supplier. It may be preferable to place low income earners on prepaid subscription. The goal of this is to make market systems more inclusive and more efficient for the poor.
- Prohibit companies from carrying out transmission and distribution of electrical energy concurrently or multiple activities that are subject to competition. From the economist’s standpoint, a perfectly competitive market has many attributes, the most important being that a single player is not able to change the market price.
- Distribution companies must provide quality services to consumers at reasonable prices. The regulator must impose on the supply companies service quality requirements (reliability, safety, etc.) that must be respected, otherwise the consumers must be compensated, for poor quality or unsupplied energy.
- There should be an independent body responsible for the provision of information to the participants in the electricity market, all companies involved must have equal access to information.
- The tax laws should be modified to ensure that all companies carrying out activities exposed to competition are treated equitably.
Given the additional flexibilities that can result from the liberalization of electricity markets, the risks of imbalance and load shedding will be greatly minimized. The techniques proposed in this paper are based on the new possibilities offered by digital technologies: active energy management in the electricity system, incentives to install controllable equipment or equipment that replaces old equipment that has become obsolete (heat pumps, heating systems, etc.); and the use of new technologies to reduce energy consumption. Incentives to implement smart or real-time technologies in the operation of emerging electricity markets have to be put in place.
With a good peak load management system, additional income can be made by the actors in the electricity market. It is therefore necessary to optimize the profitability of these actors by each of them joining the energy control market in order to enhance the efficiency of the market, regardless of the type of production, consumption or storage systems used.
Acknowledgement: The authors thank the authorities of the University of Bamenda and ENSPY-University of Yaoundé I, Cameroon for their institutional support.
Funding Statement: The authors received no specific funding for this study.
Conflicts of Interest: The authors declare that they have no conflicts of interest to report regarding the present study.
References
1. Mehigan, L., Deane, J. P., Gallachóir, B. O., Bertsch, V. (2018). A review of the role of distributed generation (DG) in future electricity systems. Energy, 163(4), 822–836. DOI 10.1016/j.energy.2018.08.022. [Google Scholar] [CrossRef]
2. Momoh, J. A., Meliopoulos, S., Saint, R. (2012). Centralized and distributed generated power systems–A comparison approach. In: Prepared for the project the future grid to enable sustainable energy systems. Washington DC: Funded by the U.S. Department of Energy, PSERC Publication. [Google Scholar]
3. Diesendorf, M. (2007). The base-load fallacy. ANZSEE Solar 2007 Conference, Sydney: Alice Springs, UNSW Press. [Google Scholar]
4. Ding, Y., Li, Y., Liu, C., Sun, Z. (2015). Solar electrical energy storage. Solar energy storage. In: Sørensen, B. (Ed.Solar energy storage, pp. 7–25. London: Academic Press. [Google Scholar]
5. Mbinkar, E. N. (2017). Real-time control of a geographically distributed system–The case of the southern network of the cameroon power system (Ph.D. thesis). ENSPY, University of Yaoundé I, Cameroon. [Google Scholar]
6. Ibrahim, I. D., Hamam, Y., Alayli, Y., Jamiru, T., Sadiku, E. R. et al. (2021). A review on Africa energy supply through renewable energy production: Nigeria, Cameroon, Ghana and South Africa as a case study. Energy Strategy Reviews, 38, 100740. DOI 10.1016/j.esr.2021.100740. [Google Scholar] [CrossRef]
7. Caire, R. (2004). Gestion de la production décentralisée dans les réseaux de distribution d’énergie électrique (Thesis). Institut National Polytechnique de Grenoble, France. [Google Scholar]
8. Blume, S. W. (2016). Electric power system basics for the nonelectrical professional. 2nd edition. Wiley-IEEE Press. [Google Scholar]
9. Shun, S., Ahmed, N. A. (2012). Wind turbine performance improvements using active flow control techniques. Procedia Engineering, 49, 83–91. DOI 10.1016/j.proeng.2012.10.115. [Google Scholar] [CrossRef]
10. Leithead, W. E., Rogers, M. C. M. (2001). A comparison of the performance of constant speed HAWT’s. Proceeding of the IEE Conference on Renewable Energy-Clean Power 2001, vol. 1993, pp. 165–170. London, UK. [Google Scholar]
11. Schwartz, R. J. (1993). Photovoltaic power generation. Proceedings of the IEEE Special Issue on Advanced Generation Technologies, vol. 81, no. 3, pp. 355–364. DOI 10.1109/5.241492. [Google Scholar] [CrossRef]
12. Newmiller, J., Chuck, W., Ropp, M., Norris, B. (2008). Renewable systems interconnection study: Distributed photovoltaic systems design and technology requirements. US Department of Energy (2007). [Google Scholar]
13. Mbinkar, E. N., Asoh, D. A., Tchuidjan, R., Baldeh, A. (2021). Design of a photovoltaic mini-grid system for rural electrification in Sub-Saharan Africa. Energy and Power Engineering, 13(3), 91–110. DOI 10.4236/epe.2021.133007. [Google Scholar] [CrossRef]
14. Klunne, W. J. (2007). Small hydropower development in Africa. https://www.esi-africa.com/top-stories/small-hydropower-development-in-africa-8038/. [Google Scholar]
15. Brown, P. D., Lopes, J. P., Matos, M. A. (2008). Optimization of pumped storage capacity in an isolated power system with large renewable penetration. IEEE Transactions on Power Systems, 23(2), 523–531. DOI 10.1109/TPWRS.2008.919419. [Google Scholar] [CrossRef]
16. Eyer, J. M., Iannucci, J. J., Corey, G. P. (2004). Energy storage benefits and market analysis handbook. In: A study for the DOE energy storage systems program. New Mexico, California, USA: Sandia National Laboratories. [Google Scholar]
17. McDowell, J. (2008). Lithium-ion technologies–Gaining ground in electricity storage. Energy Storage Association Annual Meeting, Orange, CA. [Google Scholar]
18. Daniel, H. D., Butler, P. C., Akhil, A. A., Clark, N. H., Boyes, D. J. (2010). Batteries for large-scale stationary electrical energy storage. The Electrochemical Society Interface, 19(3), 49–53. [Google Scholar]
19. Oak Ridge National Laboratory (2010). Pumped storage hydropower. https://www.hydro.org/wp-content/uploads/2017/08/PSHSummit.pdf. [Google Scholar]
20. Józsa, L., Šljivac, D., Topić, D. (2010). Stochastic modeling of pump-storage hydroelectric power plants: Part I. Technical Gazette, 17, 153–162. Osijek, Croatai. [Google Scholar]
21. Shafiqur, R., Luai, H., Alam, M. (2015). Pumped hydro energy storage system: A technological review. Renewable and Sustainable Energy Reviews, 44(9), 586–598. DOI 10.1016/j.rser.2014.12.040. [Google Scholar] [CrossRef]
22. Kluza, J., Grose, J., Eckstain, J. (2010). Who wins the peak? The battle of solar, storage and smart grid to fill peak demand. Lux Research–LRSI-R-10-02. https://www.yumpu.com/en/document/view/36598237/full-document-eos-energy-storage/19. [Google Scholar]
23. Hussin, N. S., Abdullah, M. P., Ali, A. I. M., Hassan, M. Y., Hussin, F. (2014). Residential electricity time of use (ToU) pricing for Malaysia. IEEE Conference on Energy Conversion (CENCON), pp. 429–433. Johor Bahru, Malaysia. DOI 10.1109/CENCON.2014.6967542. [Google Scholar] [CrossRef]
24. Necoechea-Porras, P. D., López, A., Salazar-Elena, J. C. (2021). Deregulation in the energy sector and Its economic effects on the power sector: A literature review. Sustainability, 13(6), 3429. DOI 10.3390/su13063429. [Google Scholar] [CrossRef]
25. Ibrahim, H., Ilinca, A., Ghandour, M., Dimitrova, M., Boulay et al. (2010). Investigation des diverses options de stockage d’énergie face À L’intégration des parcs Éoliens dans les Réseaux. 1ère Conference Franco-Syrienne Sur Les énergies Renouvelables, pp. 24–28. Damascus, Syria. [Google Scholar]
26. Jon and Willie Leone Family (2020). Basic economics of power generation, transmission and distribution, Published on EME 801. Energy Markets, Policy, and Regulation. https://www.e-education.psu.edu/eme801. [Google Scholar]
27. Bhattacharya, K., Bollen, M. H. J., Daalder, J. E. (2001). Operation of restructured power systems. New York: Springer. [Google Scholar]
28. Nguinbe, B., Tchuidjan, R., Mbinkar, E., Onanena, R., Motto, F. B. (2021). A new congestion cost allocation method in a deregulated power system using weighting of contractual preferences and geographical location of users. Electric Power Systems Research, 196(3), 107271. DOI 10.1016/j.epsr.2021.107271. [Google Scholar] [CrossRef]
29. Meyer, R. (2012). Vertical economies and the costs of separating electricity supply—A review of theoretical and empirical literature. The Energy Journal, 33(4), 161–185. [Google Scholar]
30. Harris, P. G. (2000). Impacts of deregulation on the electric power industry. IEEE Power Engineering Review, 20(10), 4–6. DOI 10.1109/39.876876. [Google Scholar] [CrossRef]
31. Taylor, B., Taylor, C. (2015). Demand response: Managing electric power peak load shortages with market mechanisms–A review of international experience and suggestions for China. Regulatory Assistance Project. https://www.raponline.org/wp-content/uploads/2016/05/rap-china-demandresponsemanagingpeakloadshortages-2015-mar.pdf. [Google Scholar]
32. Högselius, P., Kaijser, A. (2010). The politics of electricity deregulation in Sweden: The art of acting on multiple arenas. Energy Policy, 38(5), 2245–2254. DOI 10.1016/j.enpol.2009.12.012. [Google Scholar] [CrossRef]
Cite This Article
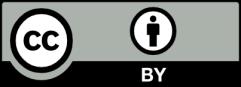
This work is licensed under a Creative Commons Attribution 4.0 International License , which permits unrestricted use, distribution, and reproduction in any medium, provided the original work is properly cited.