Open Access
ARTICLE
Modelling and Performance Analysis of Visible Light Communication System in Industrial Implementations
1 Faculty of Electrical Engineering, Faculty of Engineering, Universiti Teknologi, Malaysia, Skudai, 81310, Malaysia
2 Lightwave Communications Research Group (LCRG), Faculty of Electrical Engineering, Universiti Teknologi, Skudai, Johor, Malaysia
3 Department of Communication Engineering, Faculty of Electrical and Electronic Engineering, Universiti Tun Hussein Onn Malaysia (UTHM), Parit Raja, Batu Pahat, Johor, 86400, Malaysia
4 Faculty of Computing and Informatics, Universiti Malaysia Sabah, Kota Kinabalu, 88400, Malaysia
5 Department of Computer Science, University of São Paulo, São Paulo, Brazil
* Corresponding Author: Muzaffar Hamzah. Email:
Computers, Materials & Continua 2023, 77(2), 2189-2204. https://doi.org/10.32604/cmc.2023.035250
Received 16 August 2022; Accepted 28 January 2023; Issue published 29 November 2023
Abstract
Visible light communication (VLC) has a paramount role in industrial implementations, especially for better energy efficiency, high speed-data rates, and low susceptibility to interference. However, since studies on VLC for industrial implementations are in scarcity, areas concerning illumination optimisation and communication performances demand further investigation. As such, this paper presents a new modelling of light fixture distribution for a warehouse model to provide acceptable illumination and communication performances. The proposed model was evaluated based on various semi-angles at half power (SAAHP) and different height levels for several parameters, including received power, signal to noise ratio (SNR), and bit error rate (BER). The results revealed improvement in terms of received power and SNR with 30 Mbps data rate. Various modulations were studied to improve the link quality, whereby better average BER values of and had been achieved with 4 PAM and 8 PPM, respectively. The simulation outcomes are indeed viable for the practical warehouse model.Keywords
Radio frequency (RF) technology suffers from several restrictions that limit its performance in many applications. These restrictions include capacity crunch, electromagnetic interference, bandwidth bottleneck, high power, and cost [1–3]. Therefore, visible light communication (VLC) utilising light-emitting diodes (LED) seems to be a promising technology that can be employed to overcome all RF restrictions, besides supporting the upcoming fifth generation and beyond (5 GB) [4,5]. A laser diode (LD) based VLC was employed as an alternative technology to RF, mainly because LD is more efficient in operating power and has good reliability, and coherent light with high output power, especially in free space and long-distance communication [6,7]. The VLC occupies a high frequency that varies from 400 to 800 THz [8,9], while simultaneously offering low power consumption, no license fee, high data rate, robustness against electromagnetic interference, and many other advantages over the existing RF technology [10–13]. Additionally, VLC has dual functionality as it can be used in illumination and fast data communication concurrently [14,15], thus having a pivotal role in the fourth industrial revolution (IR 4.0) framework.
The transition of VLC from theory to industrial standardisation is discussed in [16]. Based on a recent market analysis, the universal VLC market has been targeted to hit US$ 51 billion by 2023 with a compound annual growth rate (CAGR) of 70% [16]. The VLC can be used in intelligent manufacturing and smart factory systems [17,18], primarily due to its low power consumption and cost; in comparison to incandescent and fluorescent fixtures [15,19]. The VLC has been applied in conference rooms [20], parking lots [21,22], aviation systems [23,24], mining areas [25], smart homes [26], and hospitals [27]. It has also been implemented in underwater scenarios [28,29] and other essential applications [30–32].
The rest of this paper is organised as follows: Section 2 describes the related works, while Section 3 illustrates the warehouse system model with illumination analyses, the top view of light fixture distribution, equations of channel model, and modulation techniques. Section 4 describes the simulation results and analyses of the proposed model. Finally, this paper ends with a conclusion in Section 5.
VLC is a promising technology for 6G networks and uses LEDs to generate visible light for illumination and communication. It is expected to be used in many new applications in indoor environments. Practically, VLC has been deployed in room and office models, where various light fixtures are distributed on the ceiling to provide an efficient communication system [33–35]. A number of parameters, algorithms, optimisation methods, and modulation techniques have been investigated to improve communication quality [36–38]. However, only a few studies have looked into the industrial environment despite the fact that various companies are developing light fixture products with specific characteristics for industrial environments (i.e., manufacturing, warehouse, transportation, sports facilities, and cold storage) besides mere incandescent and fluorescent fixtures [39,40].
Plenty of problems, potentials, and implementations of VLC in the industrial environment have been discussed in [1,41], where experimental designs were evidenced. The initial actual VLC results in industrial environment were reported by Berenguer et al. in [42], whereby acceptable data rate was achieved. Therefore, the objective of this paper is to investigate the VLC system for a warehouse model and to design a new model that can enhance the quality of the communication system. Different solutions were investigated and proposed to improve the VLC system quality in terms of received power, signal to noise ratio (SNR), and low bit error rate (BER) for industrial environment. One of these conceivable solutions is the distribution of light fixtures on warehouse ceilings [43–45].
Two models of light fixtures are proposed for warehouse implementation. They consist of various high-powered commercial light fixtures made by Philips and OSRAM and designed for industrial regulations with output power and different beam angles [45]. Performance evaluation of the two models was carried out, where acceptable communication quality with a data rate of up to 10 Mbps was obtained. A broadband of
The authors in [47] were analysed the effect of industrial environment, such as dust, artificial light, industrial processes, and other particles, on the VLC system. They prescribed considering these effects when designing VLC systems to avoid attenuation. The distribution of multiple light fixtures for factory automation was elaborated in [48], where advanced modulations and systems were suggested to meet the communication requirement for 5 GB. More wireless network structures for the warehouse model were investigated [49]. In [50], an experimental test using three-dimensional (3D) visible light positioning algorithm was conducted to trace a drone in the industrial setting. The authors executed the experiment by tilting the receiver and the multipath reflections [51].
There is an increasing interest in VLC research work to cater to a wide range of indoor and outdoor implementations. However, few studies have been conducted to evaluate the VLC system in industrial settings, such as warehouses and manufacturing plants [52]. The suitable distribution and the number of light fixtures needed for the room model are discussed extensively to produce an acceptable performance, especially in terms of uniform distribution in the entire room [33–35,53,54]. Notably, the distribution of 13 light fixtures outperformed the others [55]. As a result, this number of light fixtures with high power was used in this study for viable distribution in warehouse applications. The proposed warehouse model’s results were compared to the outcomes reported in [45].
The main contributions of this paper are to propose a distribution system of 13 high bay (HB) light fixtures for the warehouse model and identification of the impact of different semi-angle at half power (SAAHP), as well as various height levels, received power, SNR, and average BER. Additionally, this study evaluated the behaviour of average BER against various SAAHP for the first time in a warehouse model, where several modulation techniques are discussed to determine the most suitable one for a practical VLC system.
The general warehouse model is illustrated in Fig. 1. The dimension of the warehouse model is
Figure 1: The proposed VLC system: (a) warehouse model, (b) top view of light fixtures distribution and coordinates on the ceiling
The LOS link was considered to examine the proposed model. Various modulation techniques were investigated to minimise the BER value. Table 2 lists the main simulation parameters mentioned in [8] and [39].
The light fixtures should fulfil the illumination requirements while providing an acceptable communication performance [1]. Therefore, the illumination intensity level at angle
where I (0) represents the centre luminous intensity from a group of LEDs, while ϕ and m denote the angle of irradiance and the order of Lambertian emission, respectively; where m is related to light fixture semi-angle of half illumination
The illumination of 3D points (x, y, & z) is defined by
where ψ and d refer to the angle of incident and distance between light fixture and receiver, respectively. Light fixtures can be distributed on the ceiling of the warehouse model by using several configuration methods illustrated in [45].
Fig. 2 shows the contour of illumination distribution at a small
Figure 2: Contour of illumination distribution for the proposed warehouse model
3.2 Received Power and SNR Calculations
Upon considering the LOS link, the channel DC gain was computed as follows [45,58]:
where A represents the physical area of the photodiode (PD) detector, ψ is the FOV angle of the receiver,
For the 3D coordinates, as shown in Fig. 1, the light fixtures were installed at the position of (
Moreover, it can be expressed that
The received power (
The SNR can be further computed by:
where R refers to PD responsivity and it can be measured in amperes per watt.
where
Upon further investigation, several modulation techniques were discussed to assess the performances of BER and data rate. The simulation work performed in [45] had applied the on-off keying (OOK) modulation, which gave a data rate of 10 Mbps. Turning to this present study, it looked into the modulation techniques in the warehouse model and the effects of various SAAHP aspects on the average BER performance for the first time. The modulation techniques included binary phase shift keying (BPSK), quadrature phase shift keying (QPSK), pulse position modulation (L-PPM), and pulse amplitude modulation (M-PAM). Eqs. (11) to (15) show the BER calculations for the mentioned modulation techniques, respectively, where L and M refer to the order of modulation [59]. The BER performance was executed in the presence of Gaussian noise.
4 Simulation Results and Discussion
This section demonstrates the simulation results and the analyses of the proposed warehouse model in terms of received power, SNR, and BER, so as to enhance communication performance. Fig. 3 shows the received power and the contour of received power distribution at 5 m height level. The average received power of 0.0753 dBm was obtained at wide SAAHP
Figure 3: (a) Received power and (b) contour of power of the proposed model at 5 m height level
Fig. 4 portrays the SNR distribution and its contour at wide SAAHP
Figure 4: (a) SNR and (b) contour of SNR of the proposed model at 5 m height level
Figs. 5 and 6 demonstrate the behaviour of average received power and SNR performances at different height levels, where various SAAHP values were considered. The received power of 0.0753, −0.6065, and −1.3652 dBm had been recorded at wide SAAHP
Figure 5: Average received power against various SAAHP for different height levels
Figure 6: Average SNR against various SAAHP for different height levels
The results of the proposed model at wide SAAHP and height levels of 7.5 and 10 m were acceptable and better, when compared to previous models [45]. The variation of SAAHP led to a trade-off between received power and SNR with the coverage area in the entire warehouse model. Referring to Figs. 5 and 6, small SAAHP produced the lowest average received power and SNR distribution at 5 m height level. This is ascribed to the LOS link that bounded the light distribution downward from the light fixture, which failed to cover all areas in the warehouse model. However, both received power and SNR distribution were increased by increasing the SAAHP. Although higher SAAHP can degrade both received power and SNR, it can still yield an acceptable level that guaranteed the link quality. This trade-off was diminished with increased height level, mainly because the coverage area increased when the height of light fixture increased both light diffuses and angular range.
Table 4 summarises the received power and SNR results for the past models that used 9 Philips HB and 12 OSRAM HB light fixtures with the proposed model when SAAHP was set at
For further investigation on improving the link quality in terms of BER, different modulation techniques were assessed. Apparently, the lowest BER yielded the best communication quality. A trade-off was noted when various modulation techniques were applied for communication systems, which successfully improved both power and bandwidth efficiencies, apart from minimising BER [60]. Fig. 7 displays the BER performance for OOK-NRZ, BPSK, QPSK, 4 PPM, 8 PPM, and 4 PAM at 5 m height level.
Figure 7: BER performance of the proposed model for 5 m height level: (a) OOK-NRZ, (b) BPSK,(c) QPSK, (d) 4 PPM, (e) 8 PPM, and (f) 4 PAM
Both 8 PPM and 4 PAM produced minimum BER with a data rate of up to 30 Mbps, which met the required BER of
Fig. 8 presents the behaviour of average BER performance against various SAAHP and different height levels. At small SAAHP of
Figure 8: Evaluation of average BER performance against different SAAHP for height levels (a) 5 m, (b) 7.5 m, and (c) 10 m
The proposed model of 13 AGC HB light fixtures for the warehouse displayed improvements in terms of received power, SNR, and data rate. Better BER values were obtained when compared with the results reported by Almadani et al. Referring to the BER performance, higher order of L-PPM and M-PAM generated better average BER than other modulations. As depicted in [60], L-PPM was more efficient in terms of power, while M-PAM had better efficiency in terms of bandwidth. Hence, both modulations are suitable for the design of VLC system in a practical warehouse model.
In this paper, a new modelling of light fixtures is proposed for a warehouse to investigate the performance of communication system in terms of received power, SNR, and BER. The LOS link was considered and different height levels (5, 7.5, & 10 m) were used in the evaluation. The average received power values of 0.0753, −0.6065, and −1.3652 dBm had been obtained at height levels of 5, 7.5, and 10 m, respectively. Next, the average SNR of 69.8135 dB was recorded at 5 m height level, whereas SNR values of 68.45 dB and 66.9328 dB were achieved at 7.5 and 10 m, respectively. The behaviour of average received power and SNR over various SAAHP and height levels had been assessed. The new model showcased better performance than that reported by Almadani et al. Several modulation techniques, including OOK-NRZ, BPSK, QPSK, 4 PPM, 8 PPM, and 4 PAM, were investigated to evaluate the BER performance over various SAAHP and height levels. The average BER values of
Acknowledgement: The authors acknowledge the funding of this project by Research Management Centre (RMC), under the Professional Development Research University Grant (UTM Vot No. 06E59), Universiti Teknologi Malaysia (UTM), Malaysia. This project was also funded by Universiti Malaysia Sabah, Jalan UMS, 88400, Kota 599 Kinabalu, Malaysia, under the name of grant “Smart Vertical Farming Technology for Temperate Vegetable Cultivation in Sabah: Practicing Smart Automation System Using IR and AI Technology in Agriculture 4.0”.
Funding Statement: The manuscript APC is supported by the grant names Smart Vertical farming Technology for Temperate Vegetable Cultivation in Sabah: Practicing Smart Automation System Using IR and AI Technology in Agriculture 4.0”. It was also supported by Professional Development Research University Grant (UTM Vot No. 06E59).
Conflicts of Interest: The authors declare that they have no conflicts of interest to report regarding the present study.
References
1. Y. Almadani, D. Plets, S. Bastiaens, W. Joseph, M. Ijaz et al., “Visible light communications for industrial applications—Challenges and potentials,” Electronics, vol. 9, no. 12, pp. 1–38, 2020. [Google Scholar]
2. S. Wu, H. Wang and C. H. Youn, “Visible light communications for 5G wireless networking systems: From fixed to mobile communications,” IEEE Network, vol. 28, no. 6, pp. 41–45, 2014. [Google Scholar]
3. J. Lian, Z. Vatansever, M. Noshad and M. Brandt-Pearce, “Indoor visible light communications, networking, and applications,” Journal of Physics: Photonics, vol. 1, no. 1, pp. 1–29, 2019. [Google Scholar]
4. A. Al-Saman, M. Mohamed, M. Cheffena and A. Moldsvor, “Wideband channel characterization for 6G networks in industrial environments,” Sensors, vol. 21, no. 6, pp. 1–18, 2021. [Google Scholar]
5. M. Katz and I. Ahmed, “Opportunities and challenges for visible light communications in 6G,” in 2020 2nd 6G Wireless Summit (6G SUMMIT), Levi, Finland, pp. 1–5, 2020. [Google Scholar]
6. T. C. Wu, Y. C. Chi, H. Y. Wang, C. T. Tsai, Y. F. Huang et al., “Tricolor R/G/B laser diode based eye-safe white lighting communication beyond 8 Gbit/s,” Scientific Reports, vol. 7, no. 1, pp. 1–10, 2017. [Google Scholar]
7. Y. C. Chi, D. H. Hsieh, C. Y. Lin, H. Y. Chen, C. Y. Huang et al., “Phosphorous diffuser diverged blue laser diode for indoor lighting and communication,” Scientific Reports, vol. 5, no. 1, pp. 1–9, 2015. [Google Scholar]
8. N. Chi, Y. Zhou, Y. Wei and F. Hu, “Visible light communication in 6G: Advances, challenges, and prospects,” IEEE Vehicular Technology Magazine, vol. 15, no. 4, pp. 93–102, 2020. [Google Scholar]
9. S. Rehman, S. Ullah, P. Chong, S. Yongchareon and D. Komosny, “Visible light communication: A system perspective—Overview and challenges,” Sensors, vol. 19, no. 5, pp. 1–22, 2019. [Google Scholar]
10. A. E. Ibhaze, P. E. Orukpe and F. O. Edeko, “High capacity data rate system: Review of visible light communications technology,” Journal of Electronic Science and Technology, vol. 18, no. 3, pp. 241–265, 2020. [Google Scholar]
11. M. S. M. Gismalla, M. F. L. Abdullah, B. Das, W. A. Mabrouk and N. E. Mahfouz, “Design of an optical attocells configuration for an indoor visible light communications system,” AEU-International Journal of Electronics and Communications, vol. 112, pp. 1–6, 2019. [Google Scholar]
12. M. A. de Oliveira, E. S. Lima, M. S. P. Cunha, M. Abreu and A. C. S., “RGB-based VLC system using 5G NR standard,” Optics Communications, vol. 481, pp. 1–7, 2021. [Google Scholar]
13. S. Arai, M. Kinoshita and T. Yamazato, “Optical wireless communication: A candidate 6G technology?” IEICE Transactions on Fundamentals of Electronics, Communications and Computer Sciences, no. 1, pp. 227–234, 2021. [Google Scholar]
14. M. Z. Chowdhury, M. Shahjalal, M. K. Hasan and Y. M. Jang, “The role of optical wireless communication technologies in 5G/6G and IoT solutions: Prospects, directions, and challenges,” Applied Sciences, vol. 9, no. 20, pp. 1–20, 2019. [Google Scholar]
15. S. Riurean, M. Leba, A. Ionica, O. Stoicuta and C. Buioca, “Visible light wireless data communication in industrial environments,” IOP Conference Series: Materials Science and Engineering, vol. 572, pp. 1–10, 2019. [Google Scholar]
16. M. Uysal, “Visible light communications: From theory to industrial standardization,” in 2019 Optical Fiber Communications Conf. and Exhibition (OFC), San Diego, CA, USA, pp. 1–3, 2019. [Google Scholar]
17. X. Li, D. Li, J. Wan, A. V. Vasilakos, C. F. Lai et al., “A review of industrial wireless networks in the context of industry 4.0,” Wireless Networks, vol. 23, no. 1, pp. 23–41, 2017. [Google Scholar]
18. A. Aijaz, “Private 5G: The future of industrial wireless,” IEEE Industrial Electronics Magazine, vol. 14,no. 4, pp. 136–145, 2020. [Google Scholar]
19. H. Haas, L. Yin, C. Chen, S. Videv, D. Parol et al., “Introduction to indoor networking concepts and challenges in LiFi,” Journal of Optical Communications and Networking, vol. 12, no. 2, pp. A190–A203, 2020. [Google Scholar]
20. S. M. Mana, S. Mohammadi Kouhini, P. Hellwig, J. Hilt, P. W. Berenguer et al., “Distributed MIMO experiments for LiFi in a conference room,” in 2020 12th Int. Symp. on Communication Systems, Networks and Digital Signal Processing (CSNDSP), Porto, Portugal, pp. 1–5, 2020. [Google Scholar]
21. A. Uyrus, B. Turan, E. Basar and S. Coleri, “Visible light and mmWave propagation channel comparison for vehicular communications,” in 2019 IEEE Vehicular Networking Conf. (VNC), Los Angeles, CA, USA, pp. 1–7, 2019. [Google Scholar]
22. Y. Shen, G. Chen, X. Xu, C. Xu, G. Shen et al., “Poster: A VLC solution for smart parking,” in Proc. of the 23rd Annual Int. Conf. on Mobile Computing and Networking, Snowbird Utah, USA, pp. 579–581, 2017. [Google Scholar]
23. A. Singla, D. Sharma and S. Vashisth, “Data connectivity in flights using visible light communication,” in 2017 Int. Conf. on Computing and Communication Technologies for Smart Nation (IC3TSN), Gurgaon, India, pp. 71–74, 2017. [Google Scholar]
24. R. Martinek, L. Danys and R. Jaros, “Visible light communication system based on software defined radio: Performance study of intelligent transportation and indoor applications,” Electronics, vol. 8, no. 4, pp. 1–36, 2019. [Google Scholar]
25. H. Farahneh, F. Hussain and X. Fernando, “A new alarming system for an underground mining environment using visible light communications,” in 2017 IEEE Canada Int. Humanitarian Technology Conf. (IHTC), Toronto, ON, Canada, pp. 213–217, 2017. [Google Scholar]
26. S. V. Tiwari, A. Sewaiwar and Y. H. Chung, “Smart home multi-device bidirectional visible light communication,” Photonic Network Communications, vol. 33, no. 1, pp. 52–59, 2017. [Google Scholar]
27. W. Ding, F. Yang, H. Yang, J. Wang, X. Wang et al., “A hybrid power line and visible light communication system for indoor hospital applications,” Computers in Industry, vol. 68, pp. 170–178, 2015. [Google Scholar]
28. C. Shen, Y. Guo, H. M. Oubei, T. K. Ng, G. Liu et al., “20-meter underwater wireless optical communication link with 15 Gbps data rate,” Optics Express, vol. 24, no. 22, pp. 25502–25509, 2016. [Google Scholar] [PubMed]
29. J. Xu, A. Lin, X. Yu, Y. Song, M. Kong et al., “Underwater laser communication using an OFDM-modulated 520-nm laser diode,” IEEE Photonics Technology Letters, vol. 28, no. 20, pp. 2133–2136, 2016. [Google Scholar]
30. I. Chew, D. Karunatilaka, C. P. Tan and V. Kalavally, “Smart lighting: The way forward? Reviewing the past to shape the future,” Energy and Buildings, vol. 149, pp. 180–191, 2017. [Google Scholar]
31. M. Uysal, F. Miramirkhani, O. Narmanlioglu, T. Baykas and E. Panayirci, “IEEE 802.15.7r1 reference channel models for visible light communications,” IEEE Communications Magazine, vol. 55, no. January, pp. 212–217, 2017. [Google Scholar]
32. Z. N. Chaleshtori, S. Zvanovec, Z. Ghassemlooy, H. B. Eldeeb and M. Uysal, “Coverage of a shopping mall with flexible OLED-based visible light communications,” Optics Express, vol. 28, no. 7, pp. 10015–10026, 2020. [Google Scholar] [PubMed]
33. M. S. M. Gismalla and M. F. L. Abdullah, “Performance evaluation of optical attocells configuration in an indoor visible light communication,” Indonesian Journal of Electrical Engineering and Computer Science, vol. 14, no. 2, pp. 668–676, 2019. [Google Scholar]
34. M. S. M. Gismalla, M. F. L. Abdullah, M. Sami, N. S. M. Shah, B. Das et al., “Effect of optical attocells deployment on the RMSD spread in indoor visible light communication systems,” in 2020 Int. Conf. on Information Science and Communication Technology (ICISCT), Karachi, Pakistan, pp. 1–6, 2020. [Google Scholar]
35. F. Miramirkhani and M. Uysal, “Channel modelling for indoor visible light communications,” Philosophical Transactions of the Royal Society A: Mathematical, Physical and Engineering Sciences, vol. 378, no. 2169, pp. 1–35, 2020. [Google Scholar]
36. M. S. M. Gismalla, M. F. L. Abdullah, M. S. Ahmed, W. A. Mabrouk, N. Al-Fadhali et al., “Design and analysis of different optical attocells deployment models for indoor visible light communication system,” International Journal of Integrated Engineering, vol. 13, no. 6, pp. 253–264, 2021. [Google Scholar]
37. C. Chen, H. Yang, P. Du, W. D. Zhong, A. Alphones et al., “User-centric MIMO techniques for indoor visible light communication systems,” IEEE Systems Journal, vol. 14, no. 3, pp. 3202–3213, 2020. [Google Scholar]
38. M. Z. Afgani, H. Haas, H. Elgala and D. Knipp, “Visible light communication using OFDM,” in 2nd Int. Conf. on Testbeds and Research Infrastructures for the Development of Networks and Communities, 2006: TRIDENTCOM 2006, Barcelona, Spain, pp. 1–6, 2006. [Google Scholar]
39. B. Bechadergue and B. Azoulay, “An industrial view on LiFi challenges and future,” in 2020 12th Int. Symp. on Communication Systems, Networks and Digital Signal Processing (CSNDSP), Porto, Portugal, pp. 1–6, 2020. [Google Scholar]
40. Warehouse lighting, “LED high bay lights,” [Online]. Available: https://www.warehouse-lighting.com/collections/led-high-bay-lights [Google Scholar]
41. V. Jungnickel, P. W. Berenguer, S. M. Mana, M. Hinrichs, S. M. Kouhini et al., “LiFi for industrial wireless applications,” in 2020 Optical Fiber Communications Conf. and Exhibition (OFC), San Diego, CA, USA, pp. 1–4, 2020. [Google Scholar]
42. P. W. Berenguer, P. Hellwig, D. Schulz, J. Hilt, G. Kleinpeter et al., “Real-time optical wireless mobile communication with high physical layer reliability,” Journal of Lightwave Technology, vol. 37, no. 6, pp. 1638–1646, 2019. [Google Scholar]
43. M. Füchtenhans, E. H. Grosse and C. H. Glock, “Smart lighting systems: State-of-the-art and potential applications in warehouse order picking,” International Journal of Production Research, vol. 59, no. 12, pp. 3817–3839, 2021. [Google Scholar]
44. V. Georlette, J. S. Melgarejo, S. Bette, N. Point and V. Moeyaert, “Potential and challenges of visible light communication for industrial assembly lines with mobile workstations,” in 2021 IEEE Int. Conf. on Industry 4.0, Artificial Intelligence, and Communications Technology (IAICT), Bandung, Indonesia, pp. 228–234, 2021. [Google Scholar]
45. Y. Almadani, M. Ijaz, S. Rajbhandari, B. Adebisi and U. Raza, “Application of visible light communication in an industrial environment,” in 2018 11th Int. Symp. on Communication Systems, Networks & Digital Signal Processing (CSNDSP), Budapest, Hungary, pp. 1–6, 2018. [Google Scholar]
46. P. W. Berenguer, D. Schulz, J. Hilt, P. Hellwig, G. Kleinpeter et al., “Optical wireless MIMO experiments in an industrial environment,” IEEE Journal on Selected Areas in Communications, vol. 36, no. 1, pp. 185–193, 2018. [Google Scholar]
47. D. Schneider, A. Shrotri, H. Flatt, O. Stübbe, A. Wolf et al., “Impact of industrial environments on visible light communication,” Optics Express, vol. 29, no. 11, pp. 16087–16104, 2021. [Google Scholar] [PubMed]
48. B. Holfeld, D. Wieruch, T. Wirth, L. Thiele, S. A. Ashraf et al., “Wireless communication for factory automation: An opportunity for LTE and 5G systems,” IEEE Communications Magazine, vol. 54, no. 6, pp. 36–43, 2016. [Google Scholar]
49. A. Karaagac, J. Haxhibeqiri, W. Joseph, I. Moerman and J. Hoebeke, “Wireless industrial communication for connected shuttle systems in warehouses,” in 2017 IEEE 13th Int. Workshop on Factory Communication Systems (WFCS), Trondheim, Norway, pp. 1–4, 2017. [Google Scholar]
50. Y. Almadani, M. Ijaz, W. Joseph, S. Bastiaens, S. Rajbhandari et al., “A novel 3D visible light positioning method using received signal strength for industrial applications,” Electronics, vol. 8, no. 11, pp. 1–20, 2019. [Google Scholar]
51. Y. Almadani, M. Ijaz, B. Adebisi, S. Rajbhandari, S. Bastiaens et al., “An experimental evaluation of a 3D visible light positioning system in an industrial environment with receiver tilt and multipath reflections,” Optics Communications, vol. 483, pp. 1–11, 2021. [Google Scholar]
52. D. Schneider, A. Shrotri, O. Stuebbe, R. Lachmayer and C. A. Bunge, “Optical wireless communication in industrial areas: Potential performance and actual demand,” in Photonic Networks; 22th ITG Symp., pp. 1–8, 2021. [Google Scholar]
53. F. Seguel, A. D. Firoozabadi, P. Adasme, I. Soto, N. Krommenacker et al., “A novel strategy for LED re-utilization for visible light communications,” Optik, vol. 151, pp. 88–97, 2017. [Google Scholar]
54. H. Zheng, J. Chen, C. Yu and M. Gurusamy, “Inverse design of LED arrangement for visible light communication systems,” Optics Communications, vol. 382, pp. 615–623, 2017. [Google Scholar]
55. M. S. M. Gismalla, M. F. L. Abdullah, M. I. Niass, B. Das and W. A. Mabrouk, “Improve uniformity for an indoor visible light communication system,” International Journal of Communication Systems, vol. 33, no. 8, pp. 1–13, 2020. [Google Scholar]
56. AGC Lighting, “120W LED high bay, hot sale high bay light with competitive price-AGC lighting,” [Online]. Available: https://www.agcled.com/120w-led-high-bay.html [Google Scholar]
57. M. Khadr, A. Abd El Aziz, H. Fayed and M. Aly, “Bandwidth and BER improvement employing a pre-equalization circuit with white LED arrays in a MISO VLC system,” Applied Sciences, vol. 9, no. 5, pp. 1–14, 2019. [Google Scholar]
58. Z. Ghassemlooy, W. Popoola and S. Rajbhandari, “Visible light communications,” in Optical Wireless Communications: System and Channel Modelling with Matlab®, 2nd ed., vol. 1. Boca Raton, USA: CRC Press, pp. 443–494, 2019. [Google Scholar]
59. M. S. M. Gismalla, M. F. L. Abdullah, W. A. Mabrouk, B. Das and M. I. Niass, “Data rate and BER analysis for optical attocells configuration model in visible light communication,” in 2019 Int. Conf. on Information Science and Communication Technology (ICISCT), Karachi, Pakistan, pp. 1–6, 2019. [Google Scholar]
60. T. Y. Elganimi, “Studying the BER performance, power- and bandwidth-efficiency for FSO communication systems under various modulation schemes,” in 2013 IEEE Jordan Conf. on Applied Electrical Engineering and Computing Technologies (AEECT), Amman, Jordan, pp. 1–6, 2013. [Google Scholar]
Cite This Article
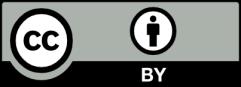
This work is licensed under a Creative Commons Attribution 4.0 International License , which permits unrestricted use, distribution, and reproduction in any medium, provided the original work is properly cited.