Open Access
REVIEW
Involvement of ZBP1 in Cancer and Its Potential Therapeutic Target Effects
1 School of Environmental and Biological Engineering, Nanjing University of Science and Technology, Nanjing, 210000, China
2 School of Basic Medical Sciences, Fudan University, Shanghai, 200032, China
* Corresponding Authors: Dan Weng. Email: ; Yan Pan. Email:
(This article belongs to the Special Issue: Cell Death and Inflammation in Signaling and Diseases)
BIOCELL 2025, 49(3), 381-398. https://doi.org/10.32604/biocell.2025.059432
Received 08 October 2024; Accepted 25 December 2024; Issue published 31 March 2025
Abstract
Z-DNA binding protein 1 (ZBP1) has emerged as a critical player in cancer biology, functioning as a cytosolic nucleic acid sensor that triggers PANoptosis, a form of programmed cell death that integrates pyroptosis, apoptosis, and necroptosis. Although ZBP1 was initially recognized for its role in antiviral defense, recent research has highlighted its importance in the tumor microenvironment (TME), where it is essential for suppressing tumor growth and proliferation. This review explores the multifaceted role of ZBP1 in various cancer types, emphasizing its ability to detect Z-nucleic acids and double-stranded RNAs, leading to the initiation of PANoptosis through receptor-interacting protein homotypic interaction motif (RHIM)-dependent interactions. However, the antitumor potential of ZBP1 involves adenosine deaminase RNA specific 1 (ADAR1), particularly its ADAR1-P150 isoform (150 kDa), which inhibits ZBP1-mediated cell death, thereby promoting tumor progression. This has spurred interest in the development of ADAR1 inhibitors and combination therapies with US Food and Drug Administration (FDA)-approved agents such as interferon-α (IFN-α) to increase ZBP1 activity. Promising studies have demonstrated that ZBP1 regulation can significantly impact tumor suppression, particularly in necrotic tumors, where its expression is correlated with reduced tumor growth. Furthermore, oligomerization of telomeric repeat-containing RNA (TERRA)-bound ZBP1 on the mitochondrial membrane has been linked to mitochondrial antiviral signaling (MAVS)-induced interferon responses, adding another layer to the tumor-suppressive functions of ZBP1. Clinical investigations into nuclear export inhibitors (NEIs) such as KPT-330 and KPT-8602, in combination with IFN therapy, have shown potential in harnessing ZBP1 to induce PANoptosis and suppress tumor growth. Additionally, the small molecule curaxin CBL0137 has been identified as a promising agent for reversing immune checkpoint blockade (ICB) resistance by inducing Z-DNA (Z form DNA) formation and ZBP1-mediated necroptosis in tumor fibroblasts. This review consolidates recent advancements in ZBP1 research, highlighting its therapeutic potential as a target for cancer treatment and its promising role in overcoming resistance to existing therapies.Keywords
Z-nucleic acid-binding protein 1 (ZBP1, also known as DAI or DLM-1) is a cytosolic sensor of DNA and RNA that plays a crucial role in the innate immune response by inducing ZBP1-dependent cell death [1]. This activation typically occurs through the recognition of double-stranded Z-form nucleic acids (Z-NAs) or Z-RNA/DNA structures [2]. ZBP1 is not only an interferon (IFN)—inducible protein but also a receptor-interacting protein homotypic interaction motif (RHIM) domain-containing protein [3]. Key molecules such as receptor-interacting kinase protein 1/3 (RIPK1/3) are integral to the regulation of apoptosis and necroptosis via caspase-8 (CASP8) [4] and mixed lineage kinase domain-like (MLKL) protein-mediated cell death [1]. The two functional domains at the N-terminus of ZBP1, zα1, and zα2 [5], recognize and bind to Z-Nas [6], triggering a cascade of immune responses. When double-stranded RNAs (dsRNAs) accumulate in the cytoplasm, ZBP1 is upregulated, inducing cell death through the binding of zα1/2 with these endogenous ligands (Z-form dsRNAs) [7]. Once binding occurs the interaction between the RHIM-domain of ZBP1 and the RHIM domain of RIPK3 starts the signaling cascade of CASP8-apoptosis, pyroptosis through gasdermin D (GSDMD) cleavage, and RIPK3-MLKL induced necroptosis [8,9]. The C-terminal domain of ZBP1 is involved in signal transduction, interacting with factors such as interferon regulatory factor 3 (IRF3) and TANK-binding kinase 1 (TBK1) to induce immune responses, particularly type I IFN responses mediated by the cyclic guanosine monophosphate/adenosine monophosphate synthase (cGAS) pathway [10,11].
Initially named DLM-1 due to its upregulation of IFN-γ-inducible genes within activated macrophages and the tumor stroma, the protein was later renamed ZBP1 after its Zα domain was found to be conserved across humans and other vertebrates, and its ability to bind Z-NAs was confirmed [2,5,8,12]. Endogenous nucleic acids can activate ZBP1, as evidenced by necroptosis through RIPK3-MLKL observed in RIPK1-deficient cells following IFN treatment and ZBP1 upregulation [3]. Jiao et al. reported that RHIM-mutated mice (Ripk1mR/mR), along with epithelial-specific Fas-associated death domain (FADD)-deficient (FADDIEC-KO) and epidermis-specific RIPK1-deficient (RIPK1E-KO) mice, experienced ZBP1-induced perinatal lethality due to Zα domain sensing of endogenous ligands [13]. Furthermore, the researchers have reported that dsRNAs derived from endogenous retroelements (EREs) could also prompt ZBP1 activation; hence, these ERE-derived dsRNAs may be treated as zα ligands. Interestingly, the RHIM of RIPK1 is deemed vital during development since it restricts the RHIM of ZBP1 from activating RIPK3-MLKL-dependent necroptosis [14]. However, the absence of RIPK1 may lead to RIPK3 activation via ZBP1, which is also critical for the survival of intercrossed RIPK1, CASP8, ZBP1, and Toll/interleukin 1 (IL-1) receptor (TIR) domain-containing adapter-inducing IFN-β (TRIF)-deficient mice [14].
The role of ZBP1 in various diseases has become more apparent since its discovery. In pulmonary infections, particularly with influenza A virus (IAV), ZBP1 has been identified as an innate sensor of Z-form dsRNAs. Studies have shown that ZBP1 can sense IAV RNA and initiate cell death, altering its characterization as a “cytosolic DNA sensor” and suggesting its broader role in sensing endogenous nucleic acids [15]. This led to a reevaluation of the role of ZBP1 in promoting cell death via RIPK1-RIPK3-MLKL necroptosis, nucleotide-binding oligomerization domain (NOD)-, leucine rich repeat (LRR)- and pyrin domain-containing protein 3 (NLRP3) inflammasome regulation, caspase cascade, and pyroptosis. These mentioned cell death signaling pathways are known to be instigated by ZBP1 in response to IAV infection [16]. Furthermore, Zα2 is crucial for the activation of programmed cell death, as the Z-RNA of IAV is sensed by this domain and colocalized in the nucleus [17]. Similarly, severe acute respiratory syndrome coronavirus 2 (SARS-CoV-2), the cause of coronavirus disease 2019 (COVID-19), induces increased Z-RNA production and necroptosis through the ZBP1-RIPK3 pathway. Studies in ZBP1-/- and RIPK3-/- mice infected with SARS-CoV-2 revealed reduced levels of proinflammatory cytokines and chemokines and immune cell infiltration, leading to decreased lung damage [18]. While necessary for viral clearance and attenuation, uncontrolled activation of these pathways can lead to excessive inflammation and cell damage.
Although ZBP1 contains a role in tumor growth inhibition, it can intriguingly serve as the backbone to the IRF3-TBK1 interaction and phosphorylation, which can influence the cell cycle and promote proliferation, as observed in multiple myeloma [19]. However, the ability of ZBP1 to induce programmed cell death through pyroptosis, necroptosis, and apoptosis has attracted attention in cancer research. TERRA transcripts have been shown to activate both ZBP1 and the cGAS-stimulator of interferon genes (cGAS-STING) pathways [20] in a cascade to eliminate tumor progression, which occurs when telomeres in crisis amplify the two pathways, initiating IFN responses [21]. This makes ZBP1 a promising therapeutic target in cancer, as modulating its activity could improve patient outcomes. The immune response suppressor adenosine deaminase RNA specific 1 (ADAR1), specifically ADAR1-p150 binds to Z-NAs since it is the only known isoform that contains zα domain competing with other zα domain-containing proteins, such as ZBP1 [22,23]. Several studies have shown that nuclear export inhibitors (NEIs), including KPT-330, KPT-8602, and leptomycin B, prevent ADAR1-p150 from translocating to the cytosol [24], along with CBL0137, which induces Z-NA formation hence ZBP1-intigated programmed cell death leading to tumor regression, and ultimately enhancing the immune checkpoint blockade (ICB) therapy and ameliorate antitumor immunity [25]. Furthermore, in endoplasmic reticulum (ER) stress or reticulophagy, ZBP1 was correlated with melanoma prognosis and tumor advancement along with several ER stress biomarkers associated with survival and progression which give importance to organelle-specific autophagy therapeutic approach [26]. Given the growing body of research on the involvement of ZBP1 in cancer, this review explores recent findings and the implications of potential ZBP1-targeting therapies.
2.1 The Instigator of Cell Death
ZBP1 induces multiple forms of programmed cell death, including pyroptosis through activation of the NLRP3 inflammasome; apoptosis through caspase cleavage; and necroptosis through the phosphorylation of mixed lineage kinase domain-like protein (p-MLKL) [27]. The functional domains of ZBP1, particularly its Zα1 and Zα2 domains, are essential for recognizing RNAs/DNAs in a Z-conformation. Additionally, the RHIM domain of ZBP1 enables interactions with other RHIM-containing proteins, such as receptor-interacting protein kinase 3 (RIPK3), further facilitating these cell death pathways.
The Zα1 and Zα2 domains, located at the N-terminus of ZBP1, are crucial for binding Z-DNA/RNA as seen in Fig. 1. Upon recognition of Z-RNA/DNA, typically in response to viral infection, cellular stress, or IFN stimulation the RHIM domain of ZBP1 can broadly instigate the formation of the ZBP1-RIPK3-RIPK1-CASP8-FADD complex triggering either necroptosis, pyroptosis, or apoptosis and commonly occur in immune cells (such as macrophage and T cells), fibroblasts and lung epithelial cells [34,35]. TRIF is another RHIM domain-containing toll-like receptor (TLR) adapter protein that acts as a docking station, much like ZBP1, for RIPK3-induced MLKL phosphorylation and oligomerization [36]. Surprisingly ZBP1 supports nucleation of TRIF-RIPK1 and TLR downstream cell death suggesting the interplay between both inflammatory responses [37]. Although RIPK1’s RHIM counteracts ZBP1-mediated cell death according to genetically engineered mouse studies, its kinase activity is needed in RIPK3 activation mediated by ZBP1 and TRIF [38,39]. Typically, the absence of both CASP8 and FADD leads to lethality caused by ZBP1- and TRIF-mediated necroptosis [40]. CASP8 plays a pivotal role in both pyroptosis and apoptosis, as it is involved in the cleavage of GSDM family proteins, particularly GSDMC, and acts as a caspase initiator in extrinsic apoptosis [29,31]. Additionally, CASP8 binds to NLRP3, forming the NLRP3-ASC-CASP1 complex [30]. This signaling scaffold enhances GSDM cleavage and activates proinflammatory cytokines, leading to pyroptosis.
Figure 1: Signaling pathway of ZBP1-dependent cell death. Z-NAs typically from viral infections are recognized by the Zα domains of ZBP1, which has a specific binding affinity for Zα2. Upon binding, the RHIM domain of ZBP1 interacts with other RHIM-containing kinases, such as RIPK3, leading to the formation of the ZBP1-RIPK3-RIPK1-CASP8-FADD complex [28]. If CASP8 is inhibited, this complex initiates necroptosis through the activation of RIPK3 and p-MLKL [29]. Conversely, if CASP8 is active, it drives apoptosis via the cleavage of CASP3/7 [30,31]. Additionally, the formation of this complex leads to the activation of the NLRP3 inflammasome, which contains CASP1. CASP1 cleaves GSDMD into its N-terminal (N-GSDMD) and C-terminal (C-GSDMD) domains, where N-GSDMD forms pores in the cell membrane, triggering pyroptosis [32]. CASP1 also processes proinflammatory cytokines, such as interleukins (IL-1β, IL-6, and IL-18), into their active forms. Furthermore, CASP8 from the apoptotic pathway can directly cleave GSDMD, illustrating the interconnected nature of ZBP1-mediated cell death pathways [33]. Abbreviations: RHIM, receptor-interacting protein (RIP) homotypic interaction motif; CAS8, caspase 8; CAS3/7, caspase 3/7; CAS1, caspase 1; GSDMD, gasdermin D; IL-1β/6/18, interleukin 1β/6/18; MLKL/pMLKL, (phosphorylated) mixed lineage kinase domain-like protein; FADD, Fas-associated death domain; NLRP3, NOD-, LRR- and pyrin domain-containing protein 3 inflammasome; ASC, apoptosis-associated speck-like protein containing a caspase recruitment domain (CARD). Illustration: Adobe Photoshop v.24.0.0
The release of inflammatory cytokines represented as orange and yellow dots in Fig. 1, such as interleukin 1β (IL-1β), interleukin 6 (IL-6), interleukin 18 (IL-18), tumor necrosis factor-α (TNF-α), and IFNs, can exacerbate cell and tissue damage, particularly during a cytokine storm caused by excessive cell death and inflammation [41]. TNF-α can bind to TNF receptor 1 (TNFR1) which initiates the non-cannon RIPK1-dependent necroptosis, RIPK1 kinase can directly phosphorylate RIPK3, which then activates p-MLKL and oligomerisation, alternatively, RIPK3 can directly phosphorylate MLKL [42]. Although these pathways are crucial for the host’s defense against infections, their dysregulation can become detrimental. However, ZBP1-dependent pyroptosis, apoptosis, and necroptosis (PANoptosis) have been shown to limit tumorigenesis in certain cancer types. A recent study by Wang and research partners reported a long non-coding RNA (lncRNA)-microRNA (miRNA)-mRNA regulatory axis related to necroptosis (RP11-138A9.1/hsa-miR-98-5p/ZBP1 axis) was linked to the immunogenic process in periodontitis where ZBP1 mechanistically modulate the necroptotic cell death in which could play a role in tumor repression [43]. ZBP1 is often highly expressed in advanced necrotic tumor cells, yet similar zα domain-containing proteins, such as ADAR1, rival ZBP1 in inhibiting tumor growth and targeting these proteins can enhance this efficiency, implicating ZBP1 as a therapeutic target for antitumor treatments and ICB therapy responsiveness [44]. Nonetheless, the rising concerns regarding ZBP1’s mechanism in the tumor environment exist.
Three identified mammalian ADAR enzymes contain a deaminase domain that catalyzes the conversion of adenosine to inosine (A-to-I), a common post-transcriptional RNA modification [45]. These ADAR family members carry dsRNA binding domains albeit ADAR2 and ADAR3 contain two, ADAR1 and its isoforms, ADAR1-p150 and p110, hold three [46]. Both isoforms contain nuclear localization signal and zβ yet only ADAR1-p150 has the Z-NA-binding zα domain promoting A-to-I editing of Alu elements, and nuclear export signal aiding in translocation between the nucleus and cytoplasm, p110, however, stays cytoplasmic [47]. ADAR1-p150 can use its zα to interact and bind to ZBP1’s zα domain thus suppressing ZBP1-RIPK3 interactions [44]. Apart from ZBP1, ADAR1 competes with other zα domain-containing proteins such as E3L and PKZ of Z-NA binding, all of which have significance in pathogen interaction and localization in stress granules [48]. ADAR1 knockout studies, particularly ADAR1-p150, caused embryonic death and lethality, increased the melanoma differentiation-associated protein 5- (MDA5) and dsRNA-dependent protein kinase- (PKR) type I IFN response, and unprompted ZBP1 activation, revealing its importance in innate immunity [49,50]. However, overexpression and activity of ADAR1 have been implicated in numerous cancer types, breast cancer, lung adenocarcinoma, and hepatocellular carcinoma to name a few, acting as a negative regulator of IFN responses [51]. Researchers have stated that ADAR1 suppresses the accumulation of Z-RNA, which is needed in ZBP1-dependent death and type I IFN response of tumor cells [23], consequently leading to ICB resistance, nevertheless, the use of curaxins and exportins proved efficient in overcoming this blockade [52]. In a study by Kulkarni and colleagues, AVA-ADR-001, a potent ADAR1-p150 inhibitor through binding with the zα domain, combined with an anti-PD1 antibody caused a tumor growth inhibition of 56% compared to the 33% of the anti-PD1 control group along with an increase in MDA5-induced IFN response in b16f10 melanoma mouse model, additionally a tumor weight reduction of 70% was noted [53]. Earlier this year a Proteolysis-Targeting Chimera (PROTAC) [54] AVA-ADR-703 small molecule inhibitor has been discovered as another ADAR1-p150 degrader with efficient selectivity [55]. With the discovery of these molecules that indirectly assist ZBP1-driven cell death and IFN responses through inhibition of p150, ADAR1 can pose a therapeutic target for ICB challenges.
3 Role of ZBP1 in Different Types of Cancer
The tumor microenvironment (TME) often experiences conditions such as glucose deprivation and mitochondrial DNA (mtDNA) released into the cytoplasm where it interacts with ZBP1 and activates a downstream signaling pathway that promotes programmed cell death and enhances inflammatory responses.
Consequently, ZBP1 comes with downsides (Fig. 2) as it can promote tumor progression, and metastasis due to tumor necroptosis, and worsen IFN-based treatments during lung or bacterial infection in the lungs. These advantages and disadvantages will be further elaborated according to the cancer types.
Figure 2: Advantages (left panel) and disadvantages (right panel) of increased ZBP1 activation and expression in certain cancer types. Abbreviations: T47DHBCC, T47D human breast cancer cell; TNBC, triple-negative breast cancer. Illustration: Adobe Photoshop v.24.0.0
Pyroptosis plays a crucial role in the development, progression, and prognosis of breast cancer. Research has shown that ZBP1 is not expressed in normal cells but is upregulated in later stages of breast cancer and that glucose deprivation leads to mtDNA damage which binds to ZBP1 triggering MLKL necroptosis [56]. These findings suggest that increasing ZBP1 levels can promote pyroptosis, thereby inhibiting the proliferation of cancer cells. ZBP1 activation also regulates the localization of β-actin and E-cadherin mRNAs at cell-cell contacts in T47DHBCC, which inhibits the chemotaxis and metastasis of these cells (Fig. 2, left panel) [57]. Huang et al. reported that high ZBP1 expression was associated with a better prognosis in TNBC patients with brain metastasis (Fig. 2, left panel) [58]. While RIPK1 was initially considered a key player in tumor necroptosis, recent studies have shown that ZBP1 and RIPK3 levels are increased during necrotic tumorigenesis. In recurrent breast cancer, high RIPK3 expression makes cells vulnerable to death through cysteine deprivation, despite RIPK3 promoter methylation, which silences its expression in primary cancer cells [59] while inhibition of G9a using BIX-01294 promotes necroptotic genes such as TNF and ZBP1 [60]. Deletion of ZBP1 however inhibits lung metastasis of the MVT-1 (derived from MMTV-c-Myc/Vegf tumors) breast cancer model, blocks tumor necrosis during development, and increases cleaved-CASP3 (apoptosis) [61]. It should be pointed out that MVT-1 breast cancer inherently induces lung metastasis [62] and Liu et al. researchers found that MLKL deletion also reduced this metastasis concluding that tumor necrosis can consequently induce cancer migration (Fig. 2, right panel) [63]. Since ZBP1 can instigate necroptosis as shown in Fig. 1 and based on the studies mentioned, this Z-NA sensor can be marked as a “double-edged sword” making limitations and further study of ZBP1 in cancer treatment necessary.
Pyroptosis is also linked to ovarian cancer. The GSDM family, which is associated with pyroptosis, is significantly different in ovarian cancer cells compared with normal cells. For example, GSDM-B is upregulated in ovarian cancer but is unchanged in advanced stages; GSDM-C and GSDM-D are expressed, while GSDM-E negatively correlates with GSDMA-D, which is regulated by the caspase family [64]. Wu et al. reported that pyroptosis-related lncRNAs, including GSDM-C, NLRP9, GSDM-A, absent in melanoma 2 (AIM2), and IL-1β, were upregulated in ovarian cancer patients [65]. Additionally, the lncRNA GAS5 (growth arrest-specific 5) induces pyroptosis in ovarian cancer [66], whereas HOXA transcript at the distal tip (HOTTIP) upregulation and GAS5 inhibition increases NLRP1 inflammasome formation [67,68]. Fisetin-induced necroptosis in ovarian cancer is mediated by ZBP1 and involves the RIP3/MLKL pathway [69], whereas Zhang and colleagues reported that high ZBP1 expression increases survival rates in ovarian cancer patients and is associated with several antitumor pathways, including the TLR, NOD-like receptor (NLR), TNF, and programmed cell death protein-1 (PD-1) checkpoint pathways [70].
Unlike in breast cancer, ZBP1 is expressed in both myeloma and normal plasma cells (PCs), as well as in late germinal center B cells. ZBP1 interacts with IRF3 and TBK1, which bind and activate cell cycle genes, promoting myeloma cell proliferation [8,19] In early-stage myeloma, an active type I IFN response restrains PC proliferation but sustains ZBP1 expression, which has a limited pro-proliferative function. In advanced multiple myeloma, ZBP1 activation plays a major role in cell proliferation. Ponnusamy and colleagues reported that isoform 1 (ZBP1 containing both Zα1 and Zα2 domains) and isoform 2 (lacking the Zα1 domain) are toxic to certain multiple myeloma cell lines, suggesting that isoform 1 is vital for myeloma cell survival [19]. Paradoxically ZBP1 acts as an “anchor” to both IRF3 and TBK1, leading to TBK1-dependent IRF3 phosphorylation, which regulates the myeloma cell cycle by activating genes such as early 2 factor 1 (E2F1), early 2 factor 2 (E2F2), Aurora B kinase (AURKB), cyclin E1 (CCNE1), and proliferation marker Ki-67 (MKI67), the authors then stated that cell cycle arrest of myeloma cells occurred when TBK1 depleted and that loss of ZBP1 reduced the IRF3-TBK1 phosphorylation. Therefore, limiting the ZBP1-IRF3 interaction could feasibly provide a therapeutic approach in multiple myeloma.
In the TME, where tumor invasion and proliferation are promoted, lung carcinoma metastasis progresses through several mechanisms. The release of elevated inflammatory effectors, including IL-6 and IL-22, and a decrease in E-cadherin lead to metastatic lesion development and tumor proliferation. IL-6 activates signal transducer and activator of transcription 1 (STAT1)/activator of transcription 1-zinc finger E-box-binding homeobox 2 antisense RNA1 (ZEB2-AS1), inducing cluster of differentiation 133+ (CD133+) cell self-renewal, whereas IL-22 enhances the phosphoinositide 3-kinase/protein kinase B/mammalian target of rapamycin (PI3K-PKB-mTOR) signaling pathway, promoting cell cycle progression [71]. Interestingly ADAR1 expression was highly seen in later stages of lung adenocarcinoma patients and proneness to lymph node metastasis, moreover ADAR1 had higher expression in CD8+ T cells, CD4+ memory T cells, M0, and M1 macrophages in tumor tissues marking its relation in TME [72]. ZBP1 also enhances M1 macrophage polarization in lung adenocarcinoma, preventing tumor growth. M1 macrophages aid in tumor suppression during early stages, but in later stages, the TME and cg09897064 methylation (ZBP1 inhibition) can increase M2 macrophage levels [73]. Alluding to ADAR1’s capability in attenuating ZBP1-induced type I IFN response [74], IFN therapies could aggravate SARS-CoV-2 infection [75]. During lung injury caused by viral infection, ZBP1 can exacerbate lung inflammation and the COVID-19 pandemic revealed that SARS-CoV-2 generates large amounts of Z-RNAs and activation of type I and III IFN responses promoting ZBP1-mediated cell death [76]. To add further, free fatty acid uptake by CD36 leads to increased ZBP1-mediated necroptosis potentiating lung epithelial injury in chronic obstructive pulmonary disease (COPD) [34]. These studies underscore the importance of maintaining ZBP1 expression throughout lung cancer progression and to impede IFN-based treatment during infectious diseases.
3.6 ZBP1 in Other Types of Cancer
In colorectal cancer, ZBP1 restricts tumorigenesis through Z-NA-dependent cell death, similar to its role in melanoma [77]. While ZBP1-driven cell death signaling is necessary to suppress tumor development, it can paradoxically trigger inflammation and release growth factors such as epidermal growth factor (EGF), TGF-β, and fibroblast growth factor (FGF), potentially promoting tumor invasion and metastasis [78,79]. Recent studies reported that CBL0137 directly induces Z-DNA formation, promoting ZBP1 activity in mouse melanoma models, whereas ADAR1 is needed to prevent severe ZBP1-driven cell death. Zhang et al. reported that ADAR1 depletion induces significant ZBP1-dependent cell death in mouse embryonic fibroblasts, suggesting that ADAR1 and its isoform, ADAR p150, are crucial for controlling tumor progression [52]. In cancer-associated fibroblasts (CAFs), CBL0137 potentially induces ZBP1-initiated necroptosis through Z-DNA formation, which can aid in antitumor responses and potentially reverse resistance to ICB therapy [52]. Patients with head and neck squamous cell carcinoma (HNSCC) displayed high expression of ZBP1 in tumor tissues compared with normal tissues alongside increased immune cell infiltration (having a high relation with T cell activation), immune checkpoints, and better survival probability [80]. In esophageal cancer (ESCA) however, in vitro experiments showed that ZBP1 was highly correlated with ESCA proliferation and migration, additionally, a high expression of ZBP1 also revealed ESCA invasion in KYSE-150 and KYSE-30 cells (human ESCA cell lines) [81]. TERRA, an evolutionarily conserved group of sequences expressed at various human chromosomal ends, was found to activate cGAS-STING-induced ZBP1 isoforms [21]. TERRA accumulation during the G1/S cell cycle transition can lead to telomere instability and DNA damage [82,83]. These maladjusted telomeres synthesize TERRA transcripts, which are taken up by human spliced ZBP1-S (lacking Zα1), initiating MAVS-induced type I IFN responses [84,85]. Moreover the immunosuppressive TME can inhibit T cell activation and function, IFN response potentiated by herpes simplex virus-based oncolytic viruses (oHSVs) in combination with Fusobacterium nucleatum outer membrane vesicle can instigate the ZBP1-mediated PANoptosis induction in tumor cells and increased the expression of PD-L1 and cytotoxic T-lymphocyte-associated protein 4 (CTLA-4) as well as CD8+ T cell infiltration further solidifying ZBP1’s role in immune checkpoint inhibitor immunotherapy [86].
4 Pharmaceutical Development in Aiding ZBP1 as a Potential Treatment for Cancer
Curaxin CBL0137 [1,1′-(9-(2-(isopropylamino)ethyl)-9H-carbazole-3,6-diyl) bis(ethan-1-one)] is a compound that intercalates with DNA, thereby inhibiting the binding of histones and DNA. CBL0137 transforms B-form DNA to Z-form DNA (Fig. 3) that can be recognized by ZBP1 to induce necroptosis which inhibits tumor growth and metastasis as seen in dying tumor stromal fibroblasts with anti-PD1 treatment [87]. This leads to the activation of p53, an IFN response, and the regulation of the nuclear factor kappa-light-chain enhancer of activated B cells (NF-κB) pathway by targeting the facilitates chromatin transcription (FACT) complex. Additionally, CBL0137 can inhibit cancer stem cells by targeting the Notch receptor 1 (NOTCH1) signaling pathway, resulting in antitumor activity in various cancers, such as glioblastoma, small-cell lung cancer, and melanoma [88,89]. CBL0137 has emerged as a promising drug for treating multiple cancer types and is currently under phase I/II clinical trials.
Figure 3: The small molecule CBL0137 promotes B-to-Z DNA inside the nucleus [87]. The Z-DNA is then recognized by ZBP1’s zα domain initiates ZBP1-RIPK3 interaction, RIPK3 then phosphorylates MLKL (indicated as a red circle with P) which translocates to the nuclear envelope ultimately leading to nuclear necroptosis, envelope disruption, and plasma membrane necroptosis [25]. Illustration: Adobe Photoshop v.24.0.0
In 2020, Sarantopoulos and fellow researchers reported the phase I trial of CBL0137’s efficacy in advanced solid tumor patients in which tumor regression for different types of cancer ranges from 10% to 22% [90]. A preclinical mouse model of medulloblastoma revealed that CBL0137’s combination with cisplatin, a chemotherapy drug, has greatly reduced tumor growth and volume and that medulloblastoma cells treated with CBL0137 alone inhibited the FACT complex and sensitized to radiation and chemotherapy [91]. On the other hand, CBL0137 showed signs of cytotoxicity in human AML and multiple myeloma cell lines and activated p53 reducing the expression of epidermal growth factor receptor (EGFR) [92]. Li and colleagues demonstrated that CBL0137 can induce necroptosis when apoptosis is inhibited, which is important for overcoming drug resistance in cancers where apoptosis is impaired. CBL0137 has been shown to induce Z-DNA formation and upregulate ZBP1 and ADAR1 expression in HepG2 cells while also promoting the expression of the RIPK1-RIPK3-MLKL necroptotic cell death markers [25]. Furthermore, a combination of CBL0137 and an anti-PD-1 antibody enhanced the recruitment of CD3+CD8+ T cells via ZBP1-dependent necroptosis, but this effect was not detected in Zbp1−/− mice and that injection of CBL0137 alone in B16-F10 melanoma instigated the rampant formation of Z-DNA at the site of injection [52]. In cervical cancer cells (CaSki), CBL0137 inhibits DNA methylation, one of the main factors for cancerous tumors caused by epigenetic changes, by suppressing the activity of the methylation-related enzymes DNA methyltransferase 1 (DNMT1) and DNA methyltransferase 3A (DNMT3A), while inhibiting the Bromodomain and Extra-Terminal Domain (BET) protein family [93]. Additionally, CBL0137 has been shown to block FACT in ovarian cancer cells, leading to the inhibition of nuclear factor erythroid 2-related factor 2 (NRF2)-mediated transcription of antioxidant genes. This inhibition causes the release of reactive oxygen species and triggers pyroptosis [94]. However, recent studies have highlighted the potential adverse effects of CBL0137, including systemic inflammatory responses, liver damage, and renal dysfunction in mice, although dose-dependent manner and patient supervision might counter these adverse effects [25,90].
4.2 Combination of Vinca Alkaloids and IFN Therapy
Vinca alkaloids are widely used chemotherapy drugs that inhibit cell mitosis and cause cell cycle arrest [95,96]. These compounds, including vinblastine (VBL), vinorelbine (VRL), and vincristine (VCR), disrupt microtubule function by binding to the β-subunit of tubulin dimers, thereby preventing the assembly of microtubules [97].
In addition to their cytotoxic effects, Vinca alkaloids have been studied in combination with IFN therapy. IFN has been shown to upregulate ZBP1 levels, inducing apoptosis and necroptosis. Frank et al. reported that combining Vinca alkaloids with IFN enhances cell death, with VRL and IFN-β being the most effective combination observed in mouse embryonic fibroblasts (MEFs) and HT29 cells (human colorectal adenocarcinoma cell line) [98]. Additionally, VRL and IFN α2c have shown promising results in increasing survival rates in patients with metastatic renal cell carcinoma, which is consistent with the results of VRL and IFN α treatment [99].
ADAR1-p150, a subtype of ADAR1, binds to ZBP1 to prevent its interaction with RIPK3 and inhibits PANoptosis, making it a key target for therapeutic intervention. NEIs have been identified as potential rivals to ADAR1-p150, preventing its export from the nucleus and thus inhibiting its interaction with ZBP1 [24,100]. ADAR1-p150 is exported to the cytosol by binding with the nuclear export protein exportin 1 (XPO1). Once in the cytosol, it binds with several zα-containing domains such as ZBP1 thus limiting ZBP1-induced cell death. NEIs, by competing with ADAR1-p150 for XPO1 binding, keep ADAR1-p150 within the nucleus preventing its zα domain from binding with Z-NAs [24]. As a selective inhibitor of nuclear export (SINE), KPT-330 explicitly targets XPO1 through irreversible binding to its nuclear export groove blocking their cargo export, including ADAR-p150, from transporting from the nucleus to the cytoplasm as seen in Fig. 4 [101–103]. This binding and export blockage can retain p53 and p21 tumor suppressor proteins within the nucleus as seen in cutaneous T-cell lymphoma [103]. Recent studies have successfully combined the NEI KPT-330 (Selinexor) with IFN in melanoma models to reduce tumor volume. Both IFN-β and IFN-γ potentiate the cleavage of several cell death factors (CASP8 & RIPK3) induced by KPT-330, initiating PANoptosis in melanoma and colorectal cancer [24].
Figure 4: ADAR1-p150 translocates to the cytoplasm as an XPO1 cargo using the nuclear pores, both ADAR1-p150’s and ZBP1’s zα then interact limiting the ZBP1-RIPK3 interaction and preventing cell death [44]. NEIs such as KPT-330 and KPT-8602 bind to XPO1 and reduce ADAR1-p150-XPO1 interaction thus inhibiting the release of ADAR1-p150 in the cytoplasm [24]. Illustration: Adobe Photoshop v.24.0.0
However, KPT-330 may have adverse effects at higher doses such as gastrointestinal toxicities and varying side effects including nausea, vomiting, diarrhea, and fatigue in metastatic colorectal cancer patients hence the maximum tolerated dose was established at 30 mg/m² in the clinical phase II study of KPT-330 in castration-resistant prostate cancer patients [104]. This led to the development of KPT-8602 (Eltanexor), a modified version that cannot penetrate the blood-brain barrier, thus allowing for higher, safer dosages [24,44,105]. KPT-8602 has been shown to bind directly to the cysteine 528 residue of XPO1, inhibiting its export function. Wang and colleagues reported that the combination of KPT-8602 and sorafenib (a tyrosine kinase inhibitor) inhibited tumor growth, particularly in cases where sorafenib alone could not overcome hepatocellular carcinoma resistance [106]. This combination therapy presents a promising strategy for suppressing the antagonistic role of ADAR1-p150 in ZBP1-induced cell death.
5 Conclusion and Promising Remarks
ZBP1, a cytosolic nucleic acid sensor that induces cell death, plays a crucial role as an initiator of PANoptosis, which is essential for controlling and combating certain NA viruses. Although ZBP1 was initially considered an IFN-inducible protein, recent studies have highlighted the significant role of ZBP1 in TME and its necessity in eliminating tumor cells. The recognition of Z-NAs through its zα domain drives ZBP1 to trigger PANoptosis by binding its RHIM domain with other RHIM-containing molecules, thereby inhibiting tumor growth and proliferation [9]. However, another zα-ligand-containing protein, ADAR1—particularly its ADAR1-p150 subtype—interferes with this binding, obstructing ZBP1-dependent cell death activation and contributing to tumor progression [7].
This challenge has spurred researchers to explore potential ADAR1 inhibitors or combination therapies with other targets, such as the FDA-approved IFN-α. This review discusses recent advances and novel therapeutic strategies involving ZBP1 and its role in various cancer types. A promising study by Nassour and colleagues proposed that the oligomerization of TERRA-bound ZBP1 on the mitochondrial membrane could initiate MAVS-induced IFN responses, reinforcing the role of ZBP1 in tumor suppression [21]. Similarly, Karki and Kanneganti suggested a strategy in which ADAR1, particularly the ADAR1-P150 subtype, is targeted by inhibiting its cytosolic activity [100]. While this approach could be a valuable checkpoint in cancer treatment, excessive ZBP1 activation could be detrimental in inflammatory diseases. Clinical studies of NEIs, such as KPT-330 and KPT-8602, are ongoing, with KPT-8602 showing greater promise [24,44]. The combination of these NEIs with IFN therapy has demonstrated potential in suppressing tumor growth and mechanistically supporting ZBP1-initiated PANoptosis [44]. Additionally, the role of ADAR1 in ICB treatment resistance has been investigated by Zhang and colleagues. They reported that CBL0137, a small curaxin molecule, could induce Z-DNA formation and reverse ICB resistance through ZBP1-mediated necroptosis in tumor fibroblasts [52]. These promising results highlight the potential therapeutic targets of ZBP1 regulation in future studies and cancer therapies.
Although these promising approaches aid ZBP1 in reducing tumor growth, knockout models of ZBP1 in myeloma cells linked its role in cell cycle gene promotion and proliferation, acting as a hub for IRF3 and TBK1 activation [19]. Therefore, studies regarding IRF3 and TBK1 might be useful in enhancing ZBP1’s anti-tumor capability, including the inhibitor of DNA binding protein 2 (ID2) that interferes with IRF3 activation [107], TBK1 inhibitor BX-795 with antiviral effects [108], and the novel LIB3S0280 that reduced cell growth and proliferation in pancreatic ductal adenocarcinoma [109]. Tumor necrosis was considerably decreased in ZBP1 knockout tumors and increased apoptosis in breast cancer lines. This led to the conclusion that ZBP1, and its zα domain, mediates necroptosis in the TME instead of RIPK1. On top of that, this cell death presumably enhances metastasis, and TME adaptation, and promotes neoplasm dispersion [61,110]. The investigation by Liao’s team found that necroptosis mediated by MLKL stimulates the CD47 signal (prevents phagocytosis and is highly expressed in the TME) and attracts tumor-associated macrophages that secrete macrophage extracellular traps (MET), forming CXCL8 and contributing to pro-metastatic extracellular matrix (ECM) degradation in pancreatic ductal adenocarcinoma with liver metastasis [111]. Furthermore, the authors highlighted that GW806742X (MLKL inhibitor) and the anti-CD47 therapeutic combination could prove useful in cancer treatment [111,112]. The mentioned inhibitors might curb the challenges about ZBP1’s tumor-promoting tendencies thus further studies are necessary. The heterogeneity of these occurrences in different tumor types raised concerns about the reliability of ZBP1 as a universal biomarker for treatment efficacy across diverse cancer populations. Moreover, the complexity of ZBP1’s interactions with other signaling pathways poses another constraint. The presence of immune checkpoint inhibitors and the TME can both alter its involvement in modulating immunological responses. These interactions may lead to unpredictable outcomes when targeting ZBP1 in combination with other therapies. Therefore, while targeting ZBP1 holds the potential to improve anti-tumor immunity and promote cancer cell death, addressing these limitations is crucial for developing safe and effective cancer therapeutics.
Acknowledgement: None.
Funding Statement: This study was supported by the National Natural Science Foundation of China under Grant 22376100. This study was also supported by the Open Project Program of the Key Laboratory of Metabolic Engineering and Biosynthesis Technology, Ministry of Industry and Information Technology, Nanjing University of Science and Technology.
Author Contributions: The authors confirm contribution to the paper as follows: study conception and design: Emmanuel Mago, Dan Weng, Jiayi Xu; literature acquisition: Emmanuel Mago, Jiayi Xu; figures: Emmanuel Mago; draft manuscript preparation: Emmanuel Mago, Jiayi Xu; manuscript revision: Emmanuel Mago, Jiayi Xu, Dan Weng, Yan Pan. All authors reviewed the results and approved the final version of the manuscript.
Availability of Data and Materials: Not applicable.
Ethics Approval: Not applicable.
Conflicts of Interest: The authors declare no conflicts of interest to report regarding the present study.
References
1. Koerner L, Wachsmuth L, Kumari S, Schwarzer R, Wagner T, Jiao H, et al. ZBP1 causes inflammation by inducing RIPK3-mediated necroptosis and RIPK1 kinase activity-independent apoptosis. Cell Death Differ. 2024;31(7):938–53. doi:10.1038/s41418-024-01321-6. [Google Scholar] [PubMed] [CrossRef]
2. Schwartz T, Behlke J, Lowenhaupt K, Heinemann U, Rich A. Structure of the DLM-1-Z-DNA complex reveals a conserved family of Z-DNA-binding proteins. Nat Struct Biol. 2001;8(9):761–5. doi:10.1038/nsb0901-761. [Google Scholar] [PubMed] [CrossRef]
3. Kesavardhana S, Kanneganti T-D. ZBP1: a STARGᐰTE to decode the biology of Z-nucleic acids in disease. J Exp Med. 2020;217(7):e20200885. doi:10.1084/jem.20200885. [Google Scholar] [PubMed] [CrossRef]
4. Song Q, Fan Y, Zhang H, Wang N. Z-DNA binding protein 1 orchestrates innate immunity and inflammatory cell death. Cytokine Growth Factor Rev. 2024;77:15–29. doi:10.1016/j.it.2017.11.002. [Google Scholar] [PubMed] [CrossRef]
5. Thapa RJ, Ingram JP, Ragan KB, Nogusa S, Boyd DF, Benitez AA, et al. DAI senses Influenza A virus genomic RNA and activates RIPK3-dependent cell death. Cell Host Microbe. 2016;20(5):674–81. doi:10.1016/j.chom.2016.09.014. [Google Scholar] [PubMed] [CrossRef]
6. Zhang T, Yin C, Boyd DF, Quarato G, Ingram JP, Shubina M, et al. Influenza virus Z-RNAs induce ZBP1-mediated necroptosis. Cell. 2020;180(6):1115–29.e13. doi:10.1016/j.cell.2020.02.050. [Google Scholar] [PubMed] [CrossRef]
7. Chen X-Y, Dai Y-H, Wan X-X, Hu X-M, Zhao W-J, Ban X-X, et al. ZBP1-mediated necroptosis: mechanisms and therapeutic implications. Molecules. 2022;28(1):52. doi:10.3390/molecules28010052. [Google Scholar] [PubMed] [CrossRef]
8. Kuriakose T, Kanneganti T-D. ZBP1: innate sensor regulating cell death and inflammation. Trends Immunol. 2018;39(2):123–34. doi:10.1016/j.it.2017.11.002. [Google Scholar] [PubMed] [CrossRef]
9. Wang R, Li H, Wu J, Cai Z-Y, Li B, Ni H, et al. Gut stem cell necroptosis by genome instability triggers bowel inflammation. Nature. 2020;580(7803):386–90. doi:10.1038/s41586-020-2127-x. [Google Scholar] [PubMed] [CrossRef]
10. Takaoka A, Wang Z, Choi MK, Yanai H, Negishi H, Ban T, et al. DAI (DLM-1/ZBP1) is a cytosolic DNA sensor and an activator of the innate immune response. Nature. 2007;448(7152):501–5. doi:10.1038/nature06013. [Google Scholar] [PubMed] [CrossRef]
11. Ablasser A, Chen ZJ. cGAS in action: expanding roles in immunity and inflammation. Science. 2019;363(6431):eaat8657. doi:10.1126/science.aat8657. [Google Scholar] [PubMed] [CrossRef]
12. Ha SC, Kim D, Hwang H-Y, Rich A, Kim Y-G, Kim KK. The crystal structure of the second Z-DNA binding domain of human DAI (ZBP1) in complex with Z-DNA reveals an unusual binding mode to Z-DNA. Proc Natl Acad Sci U S A. 2008;105(52):20671–6. doi:10.1073/pnas.0810463106. [Google Scholar] [PubMed] [CrossRef]
13. Jiao H, Wachsmuth L, Kumari S, Schwarzer R, Lin J, Eren RO, et al. Z-nucleic-acid sensing triggers ZBP1-dependent necroptosis and inflammation. Nature. 2020;580(7803):391–5. doi:10.1038/s41586-020-2129-8. [Google Scholar] [PubMed] [CrossRef]
14. Newton K, Wickliffe KE, Maltzman A, Dugger DL, Strasser A, Pham VC, et al. RIPK1 inhibits ZBP1-driven necroptosis during development. Nature. 2016;540(7631):129–33. doi:10.1038/nature20559. [Google Scholar] [PubMed] [CrossRef]
15. Thomas PG, Shubina M, Balachandran S. ZBP1/DAI-dependent cell death pathways in Influenza A virus immunity and pathogenesis. Curr Top Microbiol Immunol. 2023;442:41–63. doi:10.1007/82_2019_190. [Google Scholar] [PubMed] [CrossRef]
16. Kuriakose T, Man SM, Malireddi RKS, Karki R, Kesavardhana S, Place DE, et al. ZBP1/DAI is an innate sensor of influenza virus triggering the NLRP3 inflammasome and programmed cell death pathways. Sci Immunol. 2016;1(2):aag2045. doi:10.1126/sciimmunol.aag2045. [Google Scholar] [PubMed] [CrossRef]
17. Basavaraju S, Mishra S, Jindal R, Kesavardhana S. Emerging role of ZBP1 in Z-RNA sensing, Influenza virus-induced cell death, and pulmonary inflammation. mBio. 2022;13(3):e0040122. doi:10.1128/mbio.00401-22. [Google Scholar] [PubMed] [CrossRef]
18. Li S, Zhang Y, Guan Z, Ye M, Li H, You M, et al. SARS-CoV-2 Z-RNA activates the ZBP1-RIPK3 pathway to promote virus-induced inflammatory responses. Cell Res. 2023;33(3):201–14. doi:10.1038/s41422-022-00775-y. [Google Scholar] [PubMed] [CrossRef]
19. Ponnusamy K, Tzioni MM, Begum M, Robinson ME, Caputo VS, Katsarou A, et al. The innate sensor ZBP1-IRF3 axis regulates cell proliferation in multiple myeloma. Haematologica. 2022;107(3):721–32. doi:10.3324/haematol.2020.274480. [Google Scholar] [PubMed] [CrossRef]
20. Decout A, Katz JD, Venkatraman S, Ablasser A. The cGAS-STING pathway as a therapeutic target in inflammatory diseases. Nat Rev Immunol. 2021;21(9):548–69. doi:10.1038/s41577-021-00524-z. [Google Scholar] [PubMed] [CrossRef]
21. Nassour J, Aguiar LG, Correia A, Schmidt TT, Mainz L, Przetocka S, et al. Telomere-to-mitochondria signalling by ZBP1 mediates replicative crisis. Nature. 2023;614(7949):767–73. doi:10.1038/s41586-023-05710-8. [Google Scholar] [PubMed] [CrossRef]
22. Minton K. ADAR1 inhibits ZBP1 activation by endogenous Z-RNA. Nat Rev Genet. 2022;23(10):581. doi:10.1038/s41576-022-00525-1. [Google Scholar] [PubMed] [CrossRef]
23. De Reuver R, Verdonck S, Dierick E, Nemegeer J, Hessmann E, Ahmad S, et al. ADAR1 prevents autoinflammation by suppressing spontaneous ZBP1 activation. Nature. 2022;607(7920):784–9. doi:10.1038/s41586-022-04974-w. [Google Scholar] [PubMed] [CrossRef]
24. Camilli S, Lockey R, Kolliputi N. Nuclear export inhibitors Selinexor (KPT-330) and Eltanexor (KPT-8602) provide a novel therapy to reduce tumor growth by induction of PANoptosis. Cell Biochem Biophys. 2023;81(3):421–6. doi:10.1007/s12013-023-01135-2. [Google Scholar] [PubMed] [CrossRef]
25. Li J, Tang M, Ke R-X, Li P-L, Sheng Z-G, Zhu B-Z. The anti-cancer drug candidate CBL0137 induced necroptosis via forming left-handed Z-DNA and its binding protein ZBP1 in liver cells. Toxicol Appl Pharmacol. 2024;482:116765. doi:10.1016/j.taap.2023.116765. [Google Scholar] [PubMed] [CrossRef]
26. Qi J, Zhang Q, Wang L, Wen X, He G, Jiang X. Recent advances in organelle-specific autophagy in melanoma. Oncologie. 2024;26(6):871–83. doi:10.1515/oncologie-2024-0228. [Google Scholar] [CrossRef]
27. Zheng M, Kanneganti T-D. The regulation of the ZBP1-NLRP3 inflammasome and its implications in pyroptosis, apoptosis, and necroptosis (PANoptosis). Immunol Rev. 2020;297(1):26–38. doi:10.1111/imr.12909. [Google Scholar] [PubMed] [CrossRef]
28. Xie F, Wu D, Huang J, Liu X, Shen Y, Huang J, et al. ZBP1 condensate formation synergizes Z-NAs recognition and signal transduction. Cell Death Dis. 2024;15(7):487. doi:10.1038/s41419-024-06889-y. [Google Scholar] [PubMed] [CrossRef]
29. Fritsch M, Günther SD, Schwarzer R, Albert M-C, Schorn F, Werthenbach JP, et al. Caspase-8 is the molecular switch for apoptosis, necroptosis and pyroptosis. Nature. 2019;575(7784):683–7. doi:10.1038/s41586-019-1770-6. [Google Scholar] [PubMed] [CrossRef]
30. Feng Y, Li M, Yangzhong X, Zhang X, Zu A, Hou Y, et al. Pyroptosis in inflammation-related respiratory disease. J Physiol Biochem. 2022;78(4):721–37. doi:10.1007/s13105-022-00909-1. [Google Scholar] [PubMed] [CrossRef]
31. Malireddi RKS, Sharma BR, Bynigeri RR, Wang Y, Lu J, Kanneganti T-D. ZBP1 drives IAV-induced NLRP3 inflammasome activation and lytic cell death, PANoptosis, independent of the necroptosis executioner MLKL. Viruses. 2023;15(11):2141. doi:10.3390/v15112141. [Google Scholar] [PubMed] [CrossRef]
32. Shao R-G, Xie Q-W, Pan L-H, Lin F, Qin K, Ming S-P, et al. Necrostatin-1 attenuates Caspase-1-dependent pyroptosis induced by the RIPK1/ZBP1 pathway in ventilator-induced lung injury. Cytokine. 2022;157:155950. doi:10.1016/j.cyto.2022.155950. [Google Scholar] [PubMed] [CrossRef]
33. Demarco B, Grayczyk JP, Bjanes E, Le Roy D, Tonnus W, Assenmacher C-A, et al. Caspase-8-dependent gasdermin D cleavage promotes antimicrobial defense but confers susceptibility to TNF-induced lethality. Sci Adv. 2020;6(47):eabc3465. doi:10.1126/sciadv.abc3465. [Google Scholar] [PubMed] [CrossRef]
34. Wang J, Wang R, Li Y, Huang J, Liu Y, Wang J, et al. Lipolysis engages CD36 to promote ZBP1-mediated necroptosis-impairing lung regeneration in COPD. Cell Rep Med. 2024;5(9):101732. doi:10.1016/j.xcrm.2024.101732. [Google Scholar] [PubMed] [CrossRef]
35. Muendlein HI, Connolly WM, Magri Z, Smirnova I, Ilyukha V, Gautam A, et al. ZBP1 promotes LPS-induced cell death and IL-1β release via RHIM-mediated interactions with RIPK1. Nat Commun. 2021;12(1):86. doi:10.1038/s41467-020-20357-z. [Google Scholar] [PubMed] [CrossRef]
36. Riebeling T, Kunzendorf U, Krautwald S. The role of RHIM in necroptosis. Biochem Soc Trans. 2022;50(4):1197–205. doi:10.1042/BST20220535. [Google Scholar] [PubMed] [CrossRef]
37. Muendlein HI, Connolly WM, Magri Z, Jetton D, Smirnova I, Degterev A, et al. ZBP1 promotes inflammatory responses downstream of TLR3/TLR4 via timely delivery of RIPK1 to TRIF. Proc Natl Acad Sci U S A. 2022;119(24):e2113872119. doi:10.1073/pnas.2113872119. [Google Scholar] [PubMed] [CrossRef]
38. Imai T, Lin J, Kaya GG, Ju E, Kondylis V, Kelepouras K, et al. The RIPK1 death domain restrains ZBP1- and TRIF-mediated cell death and inflammation. Immunity. 2024;57(7):1497–513.e6. doi:10.1016/j.immuni.2024.04.016. [Google Scholar] [PubMed] [CrossRef]
39. Nagata M, Carvalho Schäfer Y, Wachsmuth L, Pasparakis M. A shorter splicing isoform antagonizes ZBP1 to modulate cell death and inflammatory responses. EMBO J. 2024;43(21):5037–56. doi:10.1038/s44318-024-00238-7. [Google Scholar] [PubMed] [CrossRef]
40. Solon M, Ge N, Hambro S, Haller S, Jiang J, Baca M, et al. ZBP1 and TRIF trigger lethal necroptosis in mice lacking caspase-8 and TNFR1. Cell Death Differ. 2024;31(5):672–82. doi:10.1038/s41418-024-01286-6. [Google Scholar] [PubMed] [CrossRef]
41. Yang L, Xie X, Tu Z, Fu J, Xu D, Zhou Y. The signal pathways and treatment of cytokine storm in COVID-19. Signal Transduct Target Ther. 2021;6(1):255. doi:10.1038/s41392-021-00679-0. [Google Scholar] [PubMed] [CrossRef]
42. Li D, Chen J, Guo J, Li L, Cai G, Chen S, et al. A phosphorylation of RIPK3 kinase initiates an intracellular apoptotic pathway that promotes corpus luteum regression. eLife. 2021;10:e67409. doi: 10.1101/2021.02.14.431152. [Google Scholar] [CrossRef]
43. Wang Z, Chen H, Peng L, He Y, Zhang X. Revealing a potential necroptosis-related axis (RP11-138A9.1/hsa-miR-98-5p/ZBP1) in periodontitis by construction of the ceRNA network. J Periodontal Res. 2023;58(5):968–85. doi:10.1111/jre.13157. [Google Scholar] [PubMed] [CrossRef]
44. Karki R, Sundaram B, Sharma BR, Lee S, Malireddi RKS, Nguyen LN, et al. ADAR1 restricts ZBP1-mediated immune response and PANoptosis to promote tumorigenesis. Cell Rep. 2021;37(3):109858. doi:10.1016/j.celrep.2021.109858. [Google Scholar] [PubMed] [CrossRef]
45. Jiao Y, Xu Y, Liu C, Miao R, Liu C, Wang Y, et al. The role of ADAR1 through and beyond its editing activity in cancer. Cell Commun Signal CCS. 2024;22(1):42. doi:10.1186/s12964-023-01465-x. [Google Scholar] [PubMed] [CrossRef]
46. Rehwinkel J, Mehdipour P. ADAR1: from basic mechanisms to inhibitors. Trends Cell Biol. 2024;550:249. doi:10.1016/j.tcb.2024.06.006. [Google Scholar] [PubMed] [CrossRef]
47. Zhang Y, Zhang J, Xue Y. ADAR1: a mast regulator of aging and immunity. Signal Transduct Target Ther. 2023;8(1):7. doi:10.1038/s41392-022-01276-5. [Google Scholar] [PubMed] [CrossRef]
48. Chiang DC, Li Y, Ng SK. The role of the Z-DNA binding domain in innate immunity and stress granules. Front Immunol. 2020;11:625504. doi:10.3389/fimmu.2020.625504. [Google Scholar] [PubMed] [CrossRef]
49. Hu S-B, Heraud-Farlow J, Sun T, Liang Z, Goradia A, Taylor S, et al. ADAR1p150 prevents MDA5 and PKR activation via distinct mechanisms to avert fatal autoinflammation. Mol Cell. 2023;83(21):3869–84.e7. doi:10.1016/j.molcel.2023.09.018. [Google Scholar] [PubMed] [CrossRef]
50. Nakahama T, Kawahara Y. The RNA-editing enzyme ADAR1: a regulatory hub that tunes multiple dsRNA-sensing pathways. Int Immunol. 2023;35(3):123–33. doi:10.1093/intimm/dxac056. [Google Scholar] [PubMed] [CrossRef]
51. Baker AR, Slack FJ. ADAR1 and its implications in cancer development and treatment. Trends Genet TIG. 2022;38(8):821–30. doi:10.1016/j.tig.2022.03.013. [Google Scholar] [PubMed] [CrossRef]
52. Zhang T, Yin C, Fedorov A, Qiao L, Bao H, Beknazarov N, et al. ADAR1 masks the cancer immunotherapeutic promise of ZBP1-driven necroptosis. Nature. 2022;606(7914):594–602. [Google Scholar] [PubMed]
53. Kulkarni A, Goswami A, Deb B, Mohanty A, Goyal S, Singh K, et al. AVA-ADR-001 suppresses tumor growth and induces anti-tumor immunity by selectively inhibiting ADAR1 p150. Research Square. 2023. doi: 10.21203/rs.3.rs-2676355/v1. [Google Scholar] [CrossRef]
54. Li R, Liu M, Yang Z, Li J, Gao Y, Tan R. Proteolysis-targeting chimeras (PROTACs) in cancer therapy: present and future. Molecules. 2022;27(24):8828. doi:10.3390/molecules27248828. [Google Scholar] [PubMed] [CrossRef]
55. Goswami A, Goyal S, Singh K, Khurana P, Deb B, Kulkarni A. Abstract 4484: discovery of a potential first-in-class Za targeted small molecule inhibitor of ADAR1 p150 which demonstrates strong anti-tumor efficacy. Cancer Res. 2024;84:4484–4. doi:10.1158/1538-7445.AM2024-4484. [Google Scholar] [CrossRef]
56. Baik JY, Choksi S. ZBP1 is a crucial regulator for tumor necroptosis during tumor development. J Immunol. 2022;208(1_Supplement):177–16. doi:10.4049/jimmunol.208.Supp.177.16. [Google Scholar] [CrossRef]
57. Gu W, Katz Z, Wu B, Park HY, Li D, Lin S, et al. Regulation of local expression of cell adhesion and motility-related mRNAs in breast cancer cells by IMP1/ZBP1. J Cell Sci. 2012;125:81–91. doi:10.1242/jcs.086132. [Google Scholar] [PubMed] [CrossRef]
58. Huang QF, Fang DL, Nong BB, Zeng J. Focal pyroptosis-related genes AIM2 and ZBP1 are prognostic markers for triple-negative breast cancer with brain metastases. Transl Cancer Res. 2021;10(11):4845–58. doi:10.21037/tcr-21-2182. [Google Scholar] [PubMed] [CrossRef]
59. Lin C-C, Mabe NW, Lin Y-T, Yang W-H, Tang X, Hong L, et al. RIPK3 upregulation confers robust proliferation and collateral cystine-dependence on breast cancer recurrence. Cell Death Differ. 2020;27(7):2234–47. doi:10.1038/s41418-020-0499-y. [Google Scholar] [PubMed] [CrossRef]
60. Mabe NW, Garcia NMG, Wolery SE, Newcomb R, Meingasner RC, Vilona BA, et al. G9a promotes breast cancer recurrence through repression of a pro-inflammatory program. Cell Rep. 2020;33(5):108341. doi:10.1016/j.celrep.2020.108341. [Google Scholar] [PubMed] [CrossRef]
61. Baik JY, Liu Z, Jiao D, Kwon H-J, Yan J, Kadigamuwa C, et al. ZBP1 not RIPK1 mediates tumor necroptosis in breast cancer. Nat Commun. 2021;12(1):2666. doi:10.1038/s41467-021-23004-3. [Google Scholar] [PubMed] [CrossRef]
62. Xiao Y, Cong M, Li J, He D, Wu Q, Tian P, et al. Cathepsin C promotes breast cancer lung metastasis by modulating neutrophil infiltration and neutrophil extracellular trap formation. Cancer Cell. 2021;39(3):423–37. doi:10.1016/j.ccell.2020.12.012. [Google Scholar] [PubMed] [CrossRef]
63. Liu Z, Choksi S, Kwon H-J, Jiao D, Liu C, Liu Z-G. Tumor necroptosis-mediated shedding of cell surface proteins promotes metastasis of breast cancer by suppressing anti-tumor immunity. Breast Cancer Res. 2023;25(1):10. doi:10.1186/s13058-023-01604-9. [Google Scholar] [PubMed] [CrossRef]
64. Berkel C, Cacan E. Differential expression and copy number variation of gasdermin (GSDM) family members, pore-forming proteins in pyroptosis, in normal and malignant serous ovarian tissue. Inflammation. 2021;44(6):2203–16. doi:10.1007/s10753-021-01493-0. [Google Scholar] [PubMed] [CrossRef]
65. Wu Y, Liang L, Li Q, Shu L, Wang P, Huang S. The role of pyroptosis-related lncRNA risk signature in ovarian cancer prognosis and immune system. Discov Oncol. 2023;14(1):149. doi:10.1007/s12672-023-00767-3. [Google Scholar] [PubMed] [CrossRef]
66. Fang Y, Tian S, Pan Y, Li W, Wang Q, Tang Y, et al. Pyroptosis: a new frontier in cancer. Biomed Pharmacother. 2020;121:109595. doi:10.1016/j.biopha.2019.109595. [Google Scholar] [PubMed] [CrossRef]
67. Lu L, Zhang Y, Tan X, Merkher Y, Leonov S, Zhu L, et al. Emerging mechanisms of pyroptosis and its therapeutic strategy in cancer. Cell Death Discov. 2022;8(1):338. doi:10.1038/s41420-022-01101-6. [Google Scholar] [PubMed] [CrossRef]
68. Zhang M, Dang P, Liu Y, Qiao B, Sun Z. Noncoding RNAs in pyroptosis and cancer progression: effect, mechanism, and clinical application. Front Immunol. 2022;13:982040. doi:10.3389/fimmu.2022.982040. [Google Scholar] [PubMed] [CrossRef]
69. Liu Y, Cao H, Zhao Y, Shan L, Lan S. Fisetin-induced cell death in human ovarian cancer cell lines via zbp1-mediated necroptosis. J Ovarian Res. 2022;15(1):57. doi:10.1186/s13048-022-00984-4. [Google Scholar] [PubMed] [CrossRef]
70. Zhang B, Li Z, Wang K, Duan M, Yin Y, Zhan Q, et al. Exploration of pyroptosis-associated prognostic gene signature and lncRNA regulatory network in ovarian cancer. Comput Biol Med. 2023;164:107343. doi:10.1016/j.compbiomed.2023.107343. [Google Scholar] [PubMed] [CrossRef]
71. Xie S, Wu Z, Qi Y, Wu B, Zhu X. The metastasizing mechanisms of lung cancer: recent advances and therapeutic challenges. Biomed Pharmacother. 2021;138:111450. doi:10.1016/j.biopha.2021.111450. [Google Scholar] [PubMed] [CrossRef]
72. Yang W, Chen K, Yu Q, Liao R, He H, Peng Y, et al. ADAR1 is a prognostic biomarker and is correlated with immune infiltration in lung adenocarcinoma. Cancer Med. 2023;12(13):14820–32. doi:10.1002/cam4.6044. [Google Scholar] [PubMed] [CrossRef]
73. Wang A, Zheng W-S, Luo Z, Bai L, Zhang S. The innovative checkpoint inhibitors of lung adenocarcinoma, cg09897064 methylation and ZBP1 expression reduction, have implications for macrophage polarization and tumor growth in lung cancer. J Transl Med. 2024;22(1):173. doi:10.1186/s12967-024-04995-1. [Google Scholar] [PubMed] [CrossRef]
74. Jiao H, Wachsmuth L, Wolf S, Lohmann J, Nagata M, Kaya GG, et al. ADAR1 averts fatal type I interferon induction by ZBP1. Nature. 2022;607(7920):776–83. doi:10.1038/s41586-022-04878-9. [Google Scholar] [PubMed] [CrossRef]
75. Karki R, Lee S, Mall R, Pandian N, Wang Y, Sharma BR, et al. ZBP1-dependent inflammatory cell death, PANoptosis, and cytokine storm disrupt IFN therapeutic efficacy during coronavirus infection. Sci Immunol. 2022;7(74):eabo6294. doi:10.1126/sciimmunol.abo6294. [Google Scholar] [PubMed] [CrossRef]
76. Yin C, Balachandran S. ZBP1 inflames the SARS-CoV-2-infected lung. Cell Res. 2023;33(5):333–4. doi:10.1038/s41422-023-00784-5. [Google Scholar] [PubMed] [CrossRef]
77. Wang F, Li K, Wang W, Hui J, He J, Cai J, et al. Sensing of endogenous retroviruses-derived RNA by ZBP1 triggers PANoptosis in DNA damage and contributes to toxic side effects of chemotherapy. Cell Death Dis. 2024;15(10):779. doi:10.1038/s41419-024-07175-7. [Google Scholar] [PubMed] [CrossRef]
78. Zhang Q, Wang Y, Liu F. Cancer-associated fibroblasts: versatile mediators in remodeling the tumor microenvironment. Cell Signal. 2023;103:110567. doi:10.1016/j.cellsig.2022.110567. [Google Scholar] [PubMed] [CrossRef]
79. Ruan R, Li L, Li X, Huang C, Zhang Z, Zhong H, et al. Unleashing the potential of combining FGFR inhibitor and immune checkpoint blockade for FGF/FGFR signaling in tumor microenvironment. Mol Cancer. 2023;22(1):60. doi:10.1186/s12943-023-01761-7. [Google Scholar] [PubMed] [CrossRef]
80. Li Y, Wang N, Yang G. Multi-omic analysis and validation reveal ZBP1 as a potential prognostic and immunotherapy-related biomarker in head and neck squamous cell carcinoma. J Stomatol Oral Maxillofac Surg. 2024;125(4S):101901. doi:10.1016/j.jormas.2024.101901. [Google Scholar] [PubMed] [CrossRef]
81. Wusiman S, Liu Y, Li H, Deng Y, Qu X, Tuerxun H, et al. Highly expressed Z-DNA binding protein 1 in esophageal cancer promotes tumor growth. Dig Dis Sci. 2024;69(5):1674–90. doi:10.1007/s10620-024-08375-z. [Google Scholar] [PubMed] [CrossRef]
82. Chebly A, Ropio J, Baldasseroni L, Prochazkova-Carlotti M, Idrissi Y, Ferrer J, et al. Telomeric repeat-containing RNA (TERRAa review of the literature and first assessment in cutaneous T-cell lymphomas. Genes. 2022;13(3):539. doi:10.3390/genes13030539. [Google Scholar] [PubMed] [CrossRef]
83. Rivosecchi J, Jurikova K, Cusanelli E. Telomere-specific regulation of TERRA and its impact on telomere stability. Semin Cell Dev Biol. 2024;157:3–23. doi:10.1016/j.semcdb.2023.11.001. [Google Scholar] [PubMed] [CrossRef]
84. Nassour J, Schmidt TT, Karlseder J. Telomeres and cancer: resolving the Paradox. Annu Rev Cancer Biol. 2021;5(1):59–77. doi:10.1146/annurev-cancerbio-050420-023410. [Google Scholar] [PubMed] [CrossRef]
85. Maelfait J, Rehwinkel J. The Z-nucleic acid sensor ZBP1 in health and disease. J Exp Med. 2023;220(8):e20221156. doi:10.1084/jem.20221156. [Google Scholar] [PubMed] [CrossRef]
86. Wang S, Song A, Xie J, Wang Y-Y, Wang W-D, Zhang M-J, et al. Fn-OMV potentiates ZBP1-mediated PANoptosis triggered by oncolytic HSV-1 to fuel antitumor immunity. Nat Commun. 2024;15(1):3669. doi:10.1084/jem.20221156. [Google Scholar] [PubMed] [CrossRef]
87. Jiao H, Pasparakis M. Inducing Z-DNA overcomes immune checkpoint blockade resistance. Cell Res. 2022;32(10):871–2. doi:10.1038/s41422-022-00705-y. [Google Scholar] [PubMed] [CrossRef]
88. Lv Y, Du Y, Li K, Ma X, Wang J, Du T, et al. The FACT-targeted drug CBL0137 enhances the effects of rituximab to inhibit B-cell non-Hodgkin’s lymphoma tumor growth by promoting apoptosis and autophagy. Cell Commun Signal CCS. 2023;21(1):16. doi:10.1186/s12964-022-01031-x. [Google Scholar] [PubMed] [CrossRef]
89. Jin M-Z, Xia B-R, Xu Y, Jin W-L. Curaxin CBL0137 exerts anticancer activity via diverse mechanisms. Front Oncol. 2018;8:598. doi:10.3389/fonc.2018.00598. [Google Scholar] [PubMed] [CrossRef]
90. Sarantopoulos J, Mahalingam D, Sharma N, Iyer RV, Ma WW, Ahluwalia MS, et al. Results of a completed phase I trial of CBL0137 administered intravenously (IV) to patients (Pts) with advanced solid tumors. J Clin Oncol. 2020;38(15_Supplement):3583–3. [Google Scholar]
91. Song H, Xi S, Chen Y, Pramanik S, Zeng J, Roychoudhury S, et al. Histone chaperone FACT complex inhibitor CBL0137 interferes with DNA damage repair and enhances sensitivity of medulloblastoma to chemotherapy and radiation. Cancer Lett. 2021;520:201–12. [Google Scholar] [PubMed]
92. Fetisov TI, Borunova AA, Antipova AS, Antoshina EE, Trukhanova LS, Gorkova TG, et al. Targeting features of Curaxin CBL0137 on hematological malignancies in vitro and in vivo. Biomedicines. 2023;11(1):230. [Google Scholar] [PubMed]
93. Maksimova V, Popova V, Prus A, Lylova E, Usalka O, Sagitova G, et al. Insights into the mechanism of Curaxin CBL0137 epigenetic activity: the induction of DNA demethylation and the suppression of BET family proteins. Int J Mol Sci. 2023;24(16):12874. [Google Scholar] [PubMed]
94. Yang C, Wang Z-Q, Zhang Z-C, Lou G, Jin W-L. CBL0137 activates ROS/BAX signaling to promote caspase-3/GSDME-dependent pyroptosis in ovarian cancer cells. Biomed Pharmacother. 2023;161:114529. [Google Scholar] [PubMed]
95. Dhyani P, Quispe C, Sharma E, Bahukhandi A, Sati P, Attri DC, et al. Anticancer potential of alkaloids: a key emphasis to colchicine, vinblastine, vincristine, vindesine, vinorelbine and vincamine. Cancer Cell Int. 2022;22(1):206. [Google Scholar] [PubMed]
96. Moudi M, Go R, Yien CYS, Nazre M. Vinca alkaloids. Int J Prev Med. 2013;4(11):1231–5. [Google Scholar] [PubMed]
97. Škubník J, Pavlíčková VS, Ruml T, Rimpelová S. Vincristine in combination therapy of cancer: emerging trends in clinics. Biology. 2021;10(9):849. [Google Scholar] [PubMed]
98. Frank T, Tuppi M, Hugle M, Dötsch V, Van Wijk SJL, Fulda S. Cell cycle arrest in mitosis promotes interferon-induced necroptosis. Cell Death Differ. 2019;26(10):2046–60. [Google Scholar] [PubMed]
99. Schmidinger M, Steger GG, Budinsky AC, Wenzel C, Brodowicz T, Locker GJ, et al. Vinorelbine and interferon-alpha2c as second-line therapy in metastatic renal cell carcinoma. Anticancer Drugs. 2000;11(3):175–9. doi:10.1097/00001813-200003000-00005. [Google Scholar] [PubMed] [CrossRef]
100. Karki R, Kanneganti T-D. ADAR1 and ZBP1 in innate immunity, cell death, and disease. Trends Immunol. 2023;44(3):201–16. doi:10.1016/j.it.2023.01.001. [Google Scholar] [PubMed] [CrossRef]
101. Liu S, Wang S, Gu R, Che N, Wang J, Cheng J, et al. The XPO1 inhibitor KPT-8602 ameliorates Parkinson’s disease by inhibiting the NF-κB/NLRP3 pathway. Front Pharmacol. 2022;13:847605. doi:10.3389/fphar.2022.847605. [Google Scholar] [PubMed] [CrossRef]
102. Landes JR, Moore SA, Bartley BR, Doan HQ, Rady PL, Tyring SK. The efficacy of selinexor (KPT-330an XPO1 inhibitor, on non-hematologic cancers: a comprehensive review. J Cancer Res Clin Oncol. 2023;149(5):2139–55. doi:10.1007/s00432-022-04247-z. [Google Scholar] [PubMed] [CrossRef]
103. Chakravarti N, Boles A, Burzinski R, Sindaco P, Isabelle C, McConnell K, et al. XPO1 blockade with KPT-330 promotes apoptosis in cutaneous T-cell lymphoma by activating the p53-p21 and p27 pathways. Sci Rep. 2024;14(1):9305. doi:10.1038/s41598-024-59994-5. [Google Scholar] [PubMed] [CrossRef]
104. Nilsson S, Stein A, Rolfo C, Kranich AL, Mann J, Papadimitriou K, et al. Selinexor (KPT-330an oral Selective Inhibitor of Nuclear Export (SINE) compound, in combination with FOLFOX in patients with Metastatic Colorectal Cancer (mCRC)—Final results of the phase I Trial SENTINEL. Curr Cancer Drug Targets. 2020;20(10):811–7. [Google Scholar] [PubMed]
105. Vercruysse T, De Bie J, Neggers JE, Jacquemyn M, Vanstreels E, Schmid-Burgk JL, et al. The second-generation exportin-1 inhibitor KPT-8602 demonstrates potent activity against acute lymphoblastic leukemia. Clin Cancer Res. 2017;23(10):2528–41. doi:10.1158/1078-0432.CCR-16-1580. [Google Scholar] [PubMed] [CrossRef]
106. Wang Z, Pan B, Yao Y, Qiu J, Zhang X, Wu X, et al. XPO1 intensifies sorafenib resistance by stabilizing acetylation of NPM1 and enhancing epithelial-mesenchymal transition in hepatocellular carcinoma. Biomed Pharmacother. 2023;160:114402. doi:10.1016/j.biopha.2023.114402. [Google Scholar] [PubMed] [CrossRef]
107. Yu C, Wang B, Zhu Y, Zhang C, Ren L, Lei X, et al. ID2 inhibits innate antiviral immunity by blocking TBK1- and IKKε-induced activation of IRF3. Sci Signal. 2022;15(715):eabh0068. doi:10.1126/scisignal.abh0068. [Google Scholar] [PubMed] [CrossRef]
108. Iqbal A, Suryawanshi R, Yadavalli T, Volety I, Shukla D. BX795 demonstrates potent antiviral benefits against herpes simplex Virus-1 infection of human cell lines. Antiviral Res. 2020;180:104814. doi:10.1016/j.antiviral.2020.104814. [Google Scholar] [PubMed] [CrossRef]
109. Shih W-H, Huang H-L, HuangFu W-C, Lin TE, Sung T-Y, Li M-C, et al. Discovery of novel TANK-Binding Kinase 1 (TBK1) inhibitor against pancreatic ductal adenocarcinoma. Int J Biol Macromol. 2024;283:137296. doi:10.1016/j.ijbiomac.2024.137296. [Google Scholar] [PubMed] [CrossRef]
110. Krishnan RP, Pandiar D, Ramani P, Jayaraman S. Necroptosis in human cancers with special emphasis on oral squamous cell carcinoma. J Stomatol Oral Maxillofac Surg. 2023;124(6S):101565. doi:10.1016/j.jormas.2023.101565. [Google Scholar] [PubMed] [CrossRef]
111. Liao C-Y, Li G, Kang F-P, Lin C-F, Xie C-K, Wu Y-D, et al. Necroptosis enhances ‘don’t eat me’ signal and induces macrophage extracellular traps to promote pancreatic cancer liver metastasis. Nat Commun. 2024;15(1):6043. doi:10.1038/s41467-024-50450-6. [Google Scholar] [PubMed] [CrossRef]
112. Liu Y, Weng L, Wang Y, Zhang J, Wu Q, Zhao P, et al. Deciphering the role of CD47 in cancer immunotherapy. J Adv Res. 2024;63:129–58. doi:10.1016/j.jare.2023.10.009. [Google Scholar] [PubMed] [CrossRef]
Cite This Article
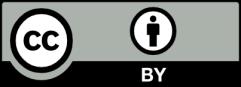
This work is licensed under a Creative Commons Attribution 4.0 International License , which permits unrestricted use, distribution, and reproduction in any medium, provided the original work is properly cited.