Open Access
ARTICLE
Human chemerin induces eryptosis at concentrations exceeding circulating levels
1 Department of Internal Medicine No. 2, Clinical Immunology and Allergology, Kharkiv National Medical University, Kharkiv, 61022, Ukraine
2 Research Institute of Experimental and Clinical Medicine, Kharkiv National Medical University, Kharkiv, 61022, Ukraine
3 Institute of Health, National University of Water and Environmental Engineering, Rivne, 61022, Ukraine
4 Department of Infectious Diseases and Phthisiology, Kharkiv National Medical University, Kharkiv, 61022, Ukraine
5 Biochemistry Department, V.N. Karazin Kharkiv National University Ministry of Education and Science of Ukraine, Kharkiv, 61022, Ukraine
6 Department of Chemistry, Biochemistry, Microbiology and Food Hygiene, State Biotechnological University, Kharkiv, 61022, Ukraine
7 Clinical Research Innovation Lab, Floor 26 Block D Qidi Technology Building 8, Tsinghua Technology Park, Beijing, 100084, China
8 Department of Cryobiochemistry, Institute for Problems of Cryobiology and Cryomedicine of the National Academy of Sciences of Ukraine, Kharkiv, 61015, Ukraine
* Corresponding Authors: YURII KOT. Email: ; ANTON TKACHENKO. Email:
BIOCELL 2024, 48(8), 1197-1208. https://doi.org/10.32604/biocell.2024.050206
Received 30 January 2024; Accepted 15 May 2024; Issue published 02 August 2024
Abstract
Introduction: Human chemerin is an adipokine that regulates chemotaxis, inflammation, and glucose metabolism. In addition, accumulating evidence suggests that chemerin promotes apoptosis, autophagy, and pyroptosis. However, there are no data on its impact on eryptosis. The current study aimed to analyze the effects of human active Glu-Ser chemerin on eryptosis in vitro. Materials and Methods: Human chemerin 0-2-10-50 µg/mL was incubated for 24 h with human erythrocytes (hematocrit 0.4%) obtained from eight healthy individuals. Flow cytometry-based determination of phospholipid scrambling, reactive oxygen species (ROS) production, and intracellular Ca levels was performed. To supplement data on ROS and Ca signaling in chemerin-mediated eryptosis, incubation in the presence or absence of antioxidants vitamin C and N-acetylcysteine and Ca-binding agent EGTA was carried out, respectively. Confocal microscopy-based techniques were used to detect reactive nitrogen species (RNS) generation, involvement of caspase-3 and caspase-8, as well as the state of lipid order in cell membranes of erythrocytes exposed to human Glu-Ser chemerin. Results: Our observations suggest that human Glu-Ser chemerin had no impact on eryptosis parameters at 2 µg/mL. However, chemerin stimulated phosphatidylserine externalization, ROS production, and Ca accumulation at higher concentrations suggesting activation of eryptosis. Ca uptake turned out to be at least partly required for chemerin-mediated eryptosis. Chemerin-mediated erythrotoxicity was additionally mediated by RNS, caspase-3, and caspase-8. Moreover, Glu-Ser chemerin promoted reduction in the liquid-ordered phase of cell membranes in erythrocytes. Conclusions: The present study first discloses that human chemerin can induce eryptosis via Ca-dependent mechanisms at concentrations noticeably exceeding circulating levels. Thus, chemerin-induced eryptosis can hardly contribute to eryptosis-mediated anemia in diseases associated with enhanced levels of chemerin in blood.Keywords
List of Abbreviations
BMI | Body Mass Index |
CMKLR1 | Chemokine-Like Receptor 1 |
GPR1 | G Protein-Coupled Receptor 1 |
CRP | C-Reactive Protein |
DCF | 2′,7′-dichlorofluorescein |
DMSO | Dimethyl sulfoxide |
EGTA | Ethylene glycol-bis(2-aminoethylether)-N, N, N′, N′-tetraacetic acid |
H2DCFDA | 2′,7′-dichlorodihydrofluorescein diacetate |
H2DCF | 2′,7′-dichlorodihydrofluorescein |
MFI | Mean fluorescence intensity |
mitROS | Mitochondrial reactive oxygen species |
NAC | N-acetylcysteine |
NOX | NADPH oxidase |
PBS | Phosphate buffer saline |
PGE2 | Prostaglandin E2 |
RARRES2 | Retinoic acid receptor responder 2 |
RBC | Red blood cell |
RhoA/ROCK | Ras homolog gene family/Rho-associated protein kinase member A |
ROS | Reactive oxygen species |
RNS | Reactive nitrogen species. |
Chemerin is a multifaceted protein discovered in 1997, which acts as a chemoattractant agent, adipokine, and growth factor [1]. Initially, it is secreted by cells as a precursor composed of 163 amino acid residues, which further undergoes proteolytic cleavage by various proteinases being converted into an active form [2]. Chemerin in humans is encoded by the retinoic acid receptor responder 2 (RARRES2) gene discovered in psoriatic patients [3,4]. Chemerin is broadly expressed in human cells, including adipocytes [5], keratinocytes [6], fibroblasts [7], endothelial cells [8], hepatocytes [9], lung cells [10], etc.
Chemerin upon its cleavage to an active form binds to chemokine-like receptor 1 (CMKLR1) or ChemR23 and G protein-coupled receptor 1 (GPR1) receptors, which are renamed as chemerin1 (chemerin receptor 1) and chemerin2 (chemerin receptor 2), respectively [11]. CMKLR1 expression is primarily observed in immune cells, including macrophages, dendritic cells, and NK cells, as well as microglial cells, vascular cells, and adipose tissue cells [12–14].
As an adipokine, it is involved in adipocyte differentiation and regulation of carbohydrate (glucose) metabolism in hepatocytes and skeletal myocytes [15,16]. Notably, recent studies have demonstrated chemerin implication in the regulation of angiogenesis [17,18] and thermogenesis [19]. Some authors indicate that chemerin shows antibacterial effects on the skin [20,21]. Given the multifaceted nature of chemerin, it is obvious that its dysregulation is observed in many human diseases. A growing number of studies demonstrate that chemerin is implicated in the pathogenesis of cardiovascular diseases [4], including metabolic syndrome, hypertension and diabetes mellitus, inflammatory disorders [22,23], and gastrointestinal pathology [24]. The multiple effects of chemerin and its role in disease provide novel horizons for chemerin-modulating therapeutic interventions.
It is important to note that chemerin-associated pathophysiological effects are mediated by the impact on fundamental cellular processes like autophagy and cell death [25]. Chemerin has been reported to induce apoptosis [25], necroptosis [26], and pyroptosis [27]. However, the ability of chemerin to affect eryptosis, a suicidal death of erythrocytes, has not been studied. Given the significantly elevated serum chemerin levels in multiple diseases, chemerin-induced eryptosis may contribute to the pathogenesis of such diseases, in particular, by mediating eryptosis-associated anemia. Eryptosis is a controlled apoptosis-like self-destruction of erythrocytes that occurs in response to unfavorable conditions to ensure clearance of damaged erythrocytes and prevent pro-inflammatory hemolysis [28]. Mounting evidence suggests that accelerated eryptosis is associated with a plethora of diseases and results in excessive clearance of erythrocytes contributing to anemia [29]. Moreover, eryptosis is known to induce platelet activation suggesting that it might contribute to excessive blood clotting [30].
This study aimed to analyze the ability of human chemerin to trigger eryptosis of erythrocytes and the molecular mechanisms behind chemerin-induced eryptosis.
Blood donors and incubation conditions
Blood specimens were provided by 8 healthy volunteers aged from 23 to 32 years (4 males and 4 females). The donors were Caucasians. The mean age was 27 ± 1.2 years of age. The mean body mass index (BMI) was 23.1 ± 1.8. Individuals with hypertension, diabetes mellitus, acute and chronic inflammatory diseases, endocrine pathology, pregnancy, obese individuals, and those receiving any treatment and unwilling to participate in the study were excluded. To confirm the lack of an inflammatory process, the levels of C-reactive protein (CRP) were measured. The Declaration of Helsinki was followed, and the study design was considered and approved by the Ethics Committee of Kharkiv National Medical University (Kharkiv, Ukraine; minutes No. 5 dated September 17, 2019). Informed consent was obligatory for all the volunteers.
Freshly collected blood was used to prepare erythrocyte suspensions. Briefly, blood samples collected in anticoagulant-containing vacutainers were centrifuged (400 g) and washed twice with phosphate buffer saline (PBS, pH7.4, BD, 349524, Franklin Lakes, NJ, USA) to obtain the erythrocyte mass. Erythrocytes (hematocrit 0.4%) were incubated with human chemerin dissolved in PBS at concentrations of 0-2-10-50 µg/mL in Ringer solution (125 mM NaCl, 5 mM KCl, 2 mM CaCl2, 2 mM MgCl2, 32 mM HEPES, and 5 mM glucose) for 24 h. Glu21-Ser157 chemerin was produced by Elabscience (Houston, TX, USA):, purity over 95%, endotoxin level below 1.0 EU per μg of the protein, molecular weight of 16.9 kDa.
Following incubation, the erythrocytes were washed twice in PBS and stained with annexin V-FITC (BD PharmingenTM FITC-Annexin V, BD Biosciences, 560931, San Jose, CA, USA) to figure out the degree of cell membrane scrambling in red blood cells (RBCs), FLUO4 AM (BD Pharmingen™ Fluo-4 AM, Becton Dickinson, 565878, Franklin Lakes, NJ, USA) to detect intracellular calcium ion levels and H2DCFDA (2′,7′-dichlorodihydrofluorescein diacetate (InvitrogenTM, D399, Waltham, MA, USA) to assess the perturbations in redox homeostasis in erythrocytes. These flow cytometric techniques are routinely used to analyze the impact of xenobiotics on eryptosis [31].
Analysis of phosphatidylserine externalization
Cell membrane scrambling, i.e., phosphatidylserine translocation from the inner leaflet of phospholipid bilayer to its outer leaflet, was assessed by measuring the degree of annexin V binding. RBCs (2 µL) incubated with chemerin for 24 h were resuspended in 100 µL Annexin-binding buffer (BD Pharmingen™ Annexin V Binding Buffer, Becton Dickinson, 556454, San Jose, CA, USA). This mixture was stained with 5 µL Annexin V-FITC. The samples were incubated for 15 min in the dark. Directly before flow cytometric measurements, 400 µL annexin-binding buffer was pipetted. Annexin V binding was measured by BD FACS Canto™ II flow cytometer (Becton Dickinson, USA). Hydrogen peroxide (0.1 mM)-exposed samples were used as a positive control [31]. Excitation was performed by a 488 nm laser, while the emission signal was registered at 525 nm.
Measurement of intraerythrocytic ROS levels
To quantify intracellular ROS levels, RBCs (2 µL) incubated with chemerin for 24 h were resuspended in 100 µL PBS and stained with a ROS-sensitive H2DCFDA (5 µM) probe [31]. The probe solution was prepared from its stock solution (5 mM) in dimethyl sulfoxide (DMSO) purchased from Sigma Aldrich (USA) and stored at −20°C. H2DCFDA is non-fluorescent, but in the cells, it is initially deacetylated by esterases to form 2′,7′-dichlorodihydrofluorescein (H2DCF), which is then converted to highly fluorescent 2′,7′-dichlorofluorescein (DCF). DCF fluorescence was determined following incubation for 30 min in the dark by BD FACSCanto™ II flow cytometer. Excitation was carried out by a 488 nm laser, while emission signal was detected at 525 nm.
Detection of cytosolic Ca2+ levels in erythrocytes
To analyze intracellular Ca2+ concentrations in the erythrocytes exposed to chemerin, FLUO4 AM staining was performed. FLUO4 AM enters the cells and gets converted into a calcium ion-sensitive FLUO4 probe. Its fluorescence depends on the cytosolic Ca2+ levels [32]. Erythrocyte suspensions (2 µL) incubated with chemerin for 24 h and 100 µL PBS were stained with FLUO4 AM (2.5 µM). FLUO4 AM solutions were prepared from its stock solution (5 mM) in DMSO. The samples were incubated in the dark for 30 min. The excitation and emission parameters were like those used for the detection of annexin V-FITC and DCF fluorescence. Hydrogen peroxide-treated (0.1 mM) samples of intact erythrocytes were used as positive controls. Mean fluorescence intensity (MFI) values of FLUO4 were analyzed to quantitatively estimate calcium ion levels in erythrocytes.
Mechanisms of chemerin-induced eryptosis
To verify the contribution of ROS and Ca2+ signaling, erythrocytes incubated with 50 µg/mL chemerin were treated or non-treated with vitamin C (0.5 mM), N-acetylcysteine (NAC, 1 mM) or Ca2+-chelating ethylene glycol-bis(2-aminoethylether)-N, N, N′, N′-tetraacetic acid (EGTA) (1 mM) [33]. Following incubation, cell membrane scrambling was assessed by annexin V-FITC staining as described above.
Flow cytometry data processing
The processing of acquired fluorescence data was carried out by FlowJo™ (v10, BD Biosciences, USA).
Detection of reactive nitrogen species (RNS) in erythrocytes
The total content of RNS in RBCs exposed to human chemerin was imaged and quantified directly in live cells using the Cellular ROS/RNS Detection Assay Kit (Abcam, ab139473, Cambridge, UK) according to the manufacturer’s guidelines. The non-fluorescent, cell-permeable NO detection dye reacts with RNS in the presence of O2 with high specificity, sensitivity, and accuracy, yielding a water-insoluble red fluorescent product.
Briefly, the ready-to-use NO Detection Reagent (Red) reagent (Abcam, ab139473, Cambridge, UK) was added to 0.5 mL of pre-warmed (37°C) DPBS buffer (Gibco, 14190144, Waltham, MA, USA) and after vortexing (IKA TTS3 shaker, Staufen, Germany, 4 s) the solution was immediately added to 0.5 mL of cell suspensions to obtain a final probe concentration of 0.01 μM in 1 × 106 cells/mL. Before monitoring, the erythrocyte suspension was incubated with the dye for 30 min at 37°C in the dark using a Galaxy 14S incubator (Eppendorf, Hamburg, Germany). Images of RNS in cells were captured by confocal microscopy (excitation 650 nm/emission 670 nm) as described below.
Lipid order changes in erythrocyte cell membranes
The solvatochromic photostable NR12S probe is a Nile Red dye-based compound that can be applied as a fluorescent membrane probe to measure lipid order changes in cell membranes, primarily reflecting cholesterol content in them. The fluorescence emission spectra of this ratiometric NR12S probe change in response to changes in lipid order, shifting towards shorter wavelengths when the probe is embedded into the liquid-ordered phase compared to the liquid-disordered phase. The NR12S probe changes its emission ratio (633 nm/550 nm) in response to lipid order alterations in cell membranes [34].
To stain erythrocytes exposed to human chemerin with the NR12S probe (Cytoskeleton Inc., MG08, Denver, CO, USA), an appropriate aliquot of its stock solution in DMSO (Invitrogen, D12345, Waltham, MA, USA) was added to 0.5 mL of DPBS buffer (Gibco, 14190144, Waltham, MA, USA) and after vortexing (IKA TTS3 shaker, Staufen, Germany, 4 s) the solution was immediately added to 0.5 mL of cell suspensions to obtain a final probe concentration of 0.01 μM (<0.25% DMSO) in 1 × 106 cells/mL. Before monitoring, the RBC suspension was incubated with the probe for 7 min at room temperature in the dark [35].
Analysis of lipid order changes in erythrocytic cell membranes was performed by calculating the ratiometric index R, which indicates the liquid-disordered phase-specific fluorescence intensity (633 nm, “red” channel)-to-liquid ordered phase-specific fluorescence intensity (550 nm, “green” channel) ratio by confocal microscopy as described below.
Caspase-3 and caspase-8 activity in erythrocytes
The activity of caspase-3 and caspase-8 was assayed directly in live cells by the Caspase Multiplex Activity Assay Fluorometric Kit (Abcam, ab219915, Cambridge, UK) according to the manufacturer’s guidelines. Fluorogenic substrates DEVD-ProRed and IETD-R110 are used in this kit as indicators of the activity of the corresponding caspases. The fluorogenic substrate binds to caspases upon their activation by upstream signaling pathways by limited proteolysis with the formation of highly fluorescent ProRed™ (red fluorescence), and R110 (green fluorescence), which reflect the activity of caspase-3 and caspase-8, respectively.
Briefly, DEVD-ProRed and IETD-R110 reagents were added to 0.5 mL of pre-warmed (37°C) Assay Buffer and after vortexing (IKA TTS3 shaker, Staufen, Germany, 4 sec) the solution was immediately added to 0.5 mL of cell suspensions to obtain a final probe concentration of 0.1 μM in 1 × 106 cells/mL. Before monitoring, the erythrocyte suspension was incubated with the dye for 30 min at 37°C in the dark using a Galaxy 14S incubator (Eppendorf, Hamburg, Germany). The dual imaging of the caspases in cells was carried out by confocal microscopy at excitation 535 nm/emission 620 nm (caspase 3, “red” channel) and excitation 650 nm/emission 670 nm (caspase 8, “green” channel) as described below.
Cell visualization was performed using a laser scanning confocal microscope FV10i-LIV (Olympus, Tokyo, Japan) equipped with a 60/1.2 NA water immersion objective. Cells were visualized in single-well PTFE slides (Thermo Scientific, cat. no. X2XER203B#, 13 µL cell suspension/well, Waltham, MA, USA), covered with coverslips (Ibidi, cat. no. 10812, Gräfelfing, Germany). Confocal images were acquired with a scanning mode format of 1024 × 1024 pixels. The pinhole aperture was 1 Airy unit. Confocal images shown are representative images of six fields of view in different regions of single-well PTFE slides. For triplicate samples, 50 cells per field of view were scored. Post-rendering of the obtained images including cell autofluorescence subtraction, measurements of fluorescence intensities, and ratiometric analysis was performed using Olympus Excellence software (Olympus licensed).
The data were analyzed by Graph Pad Prism 5.0 (Insight Partners, New York City, NY, USA) software. Kruskal-Wallis test followed by the post hoc Dunn’s test was used. Mann-Whitney test was applied to compare two groups of variables. p values below 0.05 indicated the statistical significance.
Human-active Glu21-Ser157 chemerin promotes phospholipid scrambling
Evaluation of annexin V binding, which is used to detect the degree of phospholipid scrambling, demonstrated that lower concentrations of Glu21-Ser157 chemerin (0–10 µg/mL) did not modify the percentage of phosphatidylserine-displaying cells (Figs. 1a, 1d). However, active chemerin enhanced the number of erythrocytes with translocated phosphatidylserine at the concentration of 50 µg/mL (Figs. 1a, 1d).
Figure 1: Human Glu21-Ser157 chemerin induces eryptosis promoting cell membrane scrambling, reactive oxygen species (ROS) production, and Ca2+ accumulation in erythrocytes. Human chemerin (0-2-10-50 µg/mL, 24 h) triggers cell membrane scrambling (annexin V-FITC staining) (a), excessive reactive oxygen species (ROS) production (H2DCFDA staining (b)), and intracellular Ca2+ accumulation (FLUO4-AM staining (c)). Eryptosis parameters in erythrocytes exposed to human chemerin at 0-2-10-50 µg/mL for 24 h: percentage of annexin V-positive cells (d), mean fluorescence intensity (MFI) of dichlorofluorescein (DCF) (e), and MFI of FLUO4 fluorescence (f). Kruskal-Wallis test and post hoc Dunn’s test, n = 8, Median and interquartile range. Statistical significance: ***p < 0.001.
Human Glu21-Ser157 chemerin triggers ROS generation in erythrocytes
ROS generation in RBCs incubated with human chemerin was estimated by H2DCFDA staining. As demonstrated in Figs. 1b, 1e, MFI values of DCF did not differ statistically significantly suggesting that no stimulation of ROS production was observed under the influence of lower concentrations of chemerin (2–10 µg/mL). At the same time, a slight but statistically significant elevation of ROS production in erythrocytes following exposure to chemerin was evident at 50 µg/mL.
Human Glu21-Ser157 chemerin enhances intracellular Ca2+ in erythrocytes
As illustrated in Figs. 1c, 1f, the difference in MFI values of FLUO4 of samples treated with either 2 or 10 µg/mL of human Glu21-Ser157 chemerin was statistically insignificant compared with the control samples with no chemerin added. On the contrary, chemerin at the concentration of 50 µg/mL induced intracellular Ca2+ accumulation, evidenced by statistically significant elevation of FLUO4-related fluorescence compared with the controls.
ROS signaling is not critical for chemerin-induced eryptosis
Given that Glu21-Ser157 chemerin promoted ROS accumulation in erythrocytes, we tested how antioxidants such as NAC and vitamin C affected chemerin-induced eryptosis. Unexpectedly, ascorbic acid and NAC did not abrogate chemerin-induced eryptosis, evidenced by unmodified values of cell membrane scrambling in cells exposed to 50 µg/mL and one of the antioxidants mentioned above compared with the samples incubated only with chemerin (Fig. 2).
Figure 2: Ca2+ and ROS signaling in Glu21-Ser157 chemerin-mediated eryptosis. Incubation of erythrocytes with human chemerin at 50 µg/mL in the presence of either vitamin C or N-acetylcysteine (NAC) did not blunt chemerin-mediated lipid scrambling indicating that ROS signaling was not critical for eryptosis. Incubation with EGTA reduced chemerin-associated phosphatidylserine externalization indicating that Ca2+ uptake at least partly mediated chemerin-induced eryptosis. Mann-Whitney test, n = 8. Median and interquartile range. Statistical significance: **p < 0.01.
Ca2+ influx at least partly mediates Glu21-Ser157 chemerin-induced eryptosis
Incubation of erythrocytes with chemerin in the presence or absence of Ca2+ (EGTA addition) revealed that Ca2+ binding by EGTA reduced phosphatidylserine externalization mediated by chemerin (Fig. 2). Thus, our observations suggest that Ca2+ influx is at least partly required for chemerin-mediated eryptosis.
Human Glu21-Ser157 chemerin promotes RNS generation in erythrocytes
To supplement the data on abnormal redox homeostasis in Glu21-Ser157 chemerin-treated RBCs, we evaluated the ability of this adipokine to induce RNS generation. Since RNS include various NO-derived compounds, their accumulation contributes to the development of oxidative stress common for eryptosis. As shown in Figs. 3 and 4a, the fluorescence that reflects the content of RNS was higher in cells exposed to chemerin indicating accumulation of RNS following treatment of erythrocytes with the adipokine.
Figure 3: The representative images of RNS-specific fluorescence in suspended RBCs exposed to human Glu21-Ser157 chemerin at 0-10-50 µg/mL for 24 h by laser scanning confocal microscopy; Ph–phase contrast mode, Fl–fluorescence mode, merge combines the fluorescence channel with the phase contrast image; scale bar is 10 µm.
Figure 4: Human Glu21-Ser157 chemerin triggers RNS production, changes lipid order in cell membranes, and activates caspase-3 and caspase-8 in erythrocytes. Erythrocytes were exposed to human chemerin at 0-10-50 µg/mL for 24 h: fluorescence values in relative fluorescence units (RFU) per cell, RNS (a), the ratiometric index for the NR12S probe (b), fluorescence values in rfu per cell, caspase-3 (c), and fluorescence values in rfu per cell, caspase-8 (d). Kruskal-Wallis test and post hoc Dunn’s test, n = 8, Median and interquartile range. Statistical significance: ***p < 0.001.
Chemerin-induced erythrotoxicity is associated with changes in the lipid order of cell membranes
As illustrated in Figs. 4b and 5, exposure to high concentrations of human Glu21-Ser157 chemerin increased the ratiometric index of the NR12S probe suggesting the red shift in the emission spectra, which can be explained by diminishment in the liquid-ordered phase of RBC cell membranes.
Figure 5: The representative images of NR12S-specific fluorescence in suspended erythrocytes treated with human Glu21-Ser157 chemerin at 0-10-50 µg/mL for 24 h by laser scanning confocal microscopy; Ph–phase contrast mode, Fl green and F2 red–fluorescence modes of the liquid-ordered and liquid disordered phases, respectively; merge combines the fluorescence channels with the phase contrast image; Ratio–ratiometric images (R = F2 red/Fl green); ROI–regions of interest of the presented magnified images of single cells; scale bar is 10 µm.
Chemerin-triggers eryptosis is mediated by caspase-3 and caspase-8
As shown in Figs. 4c, 4d, and 6, Glu21-Ser157 chemerin-induced eryptosis is accompanied by activation of caspases, including both caspase-8, a regulatory caspase, and a major downstream executioner caspase-3. Activation of caspases is judged by statistically significant elevation of ProRed™-and R110-dependent fluorescence in cells treated with high concentrations of human chemerin.
Figure 6: The representative images of caspase-3-and caspase-8-specific fluorescence in suspended erythrocytes exposed to human Glu21-Ser157 chemerin at 0-10-50 µg/mL for 24 h by laser scanning confocal microscopy; Ph–phase contrast mode, Fl Cas 3 and Fl Cas 8–fluorescence modes reflecting caspase-3 and caspases-8 activity, respectively; merge 1 combines the fluorescence channels; merge 2 combines the fluorescence channels with the phase contrast image; scale bar is 10 µm.
In the present study, the ability of active Glu21-Ser157 chemerin, an adipokine that is implicated in chemotaxis, angiogenesis, adipogenesis, and regulation of carbohydrate metabolism, to induce eryptosis was investigated in vitro. Eryptosis is defined as a stress-induced suicidal cell death of mature organelle-free erythrocytes accompanied by cell shrinkage, cell membrane microvesiculation, and plasma membrane lipid scrambling, i.e., features typical for apoptosis [36,37]. Eryptosis is triggered by intracytosolic elevation of Ca2+ levels, oxidative stress, and accumulation of ceramide, which is a sphingomyelin hydrolysis product [38]. In the current research, major hallmarks of eryptosis, including phosphatidylserine externalization, intracellular calcium ion levels, and ROS production, were evaluated by flow cytometry. Our findings suggest that Glu21-Ser157 chemerin induces oxidative stress and accumulation of Ca2+ inside the cytosol and plasma membrane lipid scrambling, namely phosphatidylserine to the outer leaflet of the plasma membrane phospholipid bilayer. Thus, this study shows for the first time that Glu21-Ser157 chemerin induces eryptosis. Analysis of the mechanisms for chemerin-induced eryptosis reveals the involvement of Ca2+ influx-mediated pathways. Calcium ion entry is known to promote cell membrane scrambling and opening of Ca2+-dependent K+ channels or Gardos channels [37]. ROS accumulation is known to trigger Ca2+ channels [36]. It has been reported that chemerin promotes calcium mobilization in smooth muscle cells of blood vessels stimulating arterial contraction via a CMKLR1-mediated Ras homolog gene family, member A(RhoA)/Rho-associated protein kinase (ROCK) pathway [39]. Thus, our findings are consistent with other observations emphasizing an important role of Ca2+ signaling in chemerin-mediated effects. Additionally, RNS are found to contribute to chemerin-mediated oxidative stress promoting eryptosis. The implication of RNS in cell death of erythrocytes is less studied than ROS. However, RNS are shown to induce cell death of RBCs [40] and eryptosis in particular [41]. Our study supplements other findings on the important role of RNS in the cell death of erythrocytes, which is of particular importance given the participation of erythrocytes in NO metabolism, e.g., NO scavenging [42].
It has been claimed in several papers that chemerin-CMKLR1 interaction promotes ROS overgeneration in cells [43,44]. However, in this study, we believe that the observed effects are not associated with the ligand-receptor interactions, since, to our knowledge, there is no data on the presence of chemerin receptors on the surface of erythrocytes. Moreover, chemerin-induced oxidative stress has been reported to be associated with mitochondrial dysfunction and mitochondrial ROS (mitROS) overproduction [45]. Alternatively, ROS generation in cells in response to chemerin can be catalyzed by NADPH oxidase (NOX) [8,46]. Since erythrocytes lack mitochondria, mitROS cannot contribute to eryptosis. Unlike mitochondria-derived ROS, NOX is expressed in erythrocytes, and NOX-derived ROS are known to trigger eryptosis [41]. Surprisingly, ROS signaling triggered by chemerin in erythrocytes was not crucial for eryptosis, since chemerin-induced eryptosis is not blunted by antioxidants. This suggests the involvement of alternative signaling pathways, which have to be unveiled in further studies.
In contrast to apoptosis, caspase-3 is not inevitably recruited in eryptosis and caspase-3-independent eryptosis is extremely common [28]. In our study, caspase-3 is found to be activated in chemerin-induced eryptosis and might contribute to phosphatidylserine externalization along with ROS, since caspase-3 is reported to promote lipid membrane scrambling through diminishing the activity of flippases [47]. Caspase-8, which is upstream of caspase-3, is also known to contribute to eryptosis and is activated upon oxidative stress [37], which is in line with our observations on chemerin-induced oxidative stress in RBCs.
Eryptotic erythrocytes are known to be cleared from the bloodstream via efferocytosis, a process associated with phagocytosis of eryptotic cells by phagocytes in a phosphatidylserine-mediated fashion [48–50]. Thus, accelerated eryptosis facilitates the clearance of erythrocytes, which may contribute to anemia [29,50,51]. According to different studies, normal mean chemerin serum levels vary significantly reaching up to 227.5 ng/mL [52–54]. Multiple metabolic and inflammatory diseases are associated with elevated circulating levels of chemerin. In this case, serum concentrations of this adipokine may reach up to 1000 ng/mL [55,56]. The concentrations of chemerin that induce eryptosis in this study are orders of magnitude higher than those observed in blood serum both under normal and pathological conditions. Thus, it can be assumed that chemerin does not contribute to erythrocyte loss via eryptosis and anemia development in diseases associated with its excessive circulating levels as it occurs in end-stage renal disease [57]. However, it is important to note that the inactive precursor chem163S is the most common form of chemerin in blood whose activation can occur in response to blood clotting or inflammation locally [2]. Thus, the local concentration of the active Glu21-Ser157 chemerin might reach higher values than in circulation, which explains why the impact of this active form on eryptosis is analyzed in this study. Nevertheless, to our knowledge, there are no data that currently support the hypothesis that even local concentrations of active chemerin in the disease might exceed 1 μg/mL, at which eryptosis is not induced [58]. This emphasizes that chemerin can hardly contribute to eryptosis induction even locally.
Several limitations should be acknowledged in this study, including a small sample size and lack of assessing the contribution of other eryptosis messengers such as ceramide or prostaglandin E2 (PGE2) and multiple eryptosis-regulating kinases, including p38 mitogen-activated protein kinase, casein kinase 1α or protein kinase C. Additionally, it should be acknowledged that the commercially available chemerin used in this study contains traces of protein impurities and endotoxin, which might contribute to erythrotoxicity. The lack of chem1 and chem2 expression in erythrocytes was not confirmed experimentally. This suggests the requirement for further studies on chemerin-induced cell death pathways and elucidation of their molecular mechanisms.
We report that human adipokine Glu21-Ser157 chemerin can induce eryptosis, a suicidal cell death of erythrocytes, at significantly supraphysiological concentrations. Chemerin-induced eryptosis is a Ca2+-dependent process. More studies are required to elucidate the pathophysiological and therapeutic significance of these findings.
Acknowledgement: None.
Funding Statement: This study was performed as a fragment of a research entitled “Ischemic Heart Disease under Comorbidity: Pathogenetic Aspects of the Development, Course, Diagnosis and Treatment Optimization (Kharkiv National Medical University, Kharkiv, Ukraine; State Registration Number 0118U000929).
Author Contributions: All authors have made substantial contributions to this paper. Conceptualization: MT, YuK, AT; Data analysis&Original draft writing: MT, LT, YuK, AT; Experimental data acquisition: MT, VN, VP, DB, AO, KK, LF; Interpretation of data: AT, YuK, MT, KK, DB, AO, VN, VP; Statistical analysis: LT, VN, DB, LF, AO; Funding and resources: YuK, AT. All authors read and approved the final version of the manuscript.
Availability of Data and Materials: Raw data were generated at Kharkiv National Medical University and are available from the corresponding author Anton Tkachenko on request.
Ethics Approval: The study was approved by the Ethics Committee of Kharkiv National Medical University (minutes No. 5 dated September 17, 2019). Informed consent was provided.
Conflicts of Interest: The authors have no conflict of interest to disclose.
References
1. Tan L, Lu X, Danser AHJ, Verdonk K. The role of chemerin in metabolic and cardiovascular disease: a literature review of its physiology and pathology from a nutritional perspective. Nutrients. 2023;15(13):2878. doi:10.3390/nu15132878. [Google Scholar] [PubMed] [CrossRef]
2. Zhao L, Leung LL, Morser J. Chemerin forms: their generation and activity. Biomedicines. 2022;10(8):2018. doi:10.3390/biomedicines10082018. [Google Scholar] [PubMed] [CrossRef]
3. Ferland DJ, Watts SW. Chemerin: a comprehensive review elucidating the need for cardiovascular research. Pharmacol Res. 2015;99:351–61. doi:10.1016/j.phrs.2015.07.018. [Google Scholar] [PubMed] [CrossRef]
4. Helfer G, Wu QF. Chemerin: a multifaceted adipokine involved in metabolic disorders. J Endocrinol. 2018;238(2):R79–94. doi:10.1530/JOE-18-0174. [Google Scholar] [PubMed] [CrossRef]
5. Liu R, Han Y, Huang C, Hou M, Cheng R, Wang S, et al. Adipocyte-derived chemerin rescues lipid overload-induced cardiac dysfunction. iScience. 2023;26(4):106495. doi:10.1016/j.isci.2023.106495. [Google Scholar] [PubMed] [CrossRef]
6. Chen Y, Song Y, Wang Z, Lai Y, Yin W, Cai Q, et al. The chemerin-CMKLR1 axis in keratinocytes impairs innate host defense against cutaneous Staphylococcus aureus infection. Cell Mol Immunol. 2024. doi:10.1038/s41423-024-01152-y. [Google Scholar] [CrossRef]
7. Yamamoto A, Sagara A, Otani K, Okada M, Yamawaki H. Chemerin-9 stimulates migration in rat cardiac fibroblasts in vitro. Eur J Pharmacol. 2021;912:174566. doi:10.1016/j.ejphar.2021.174566. [Google Scholar] [PubMed] [CrossRef]
8. Pankiewicz K, Issat T. Understanding the role of chemerin in the pathophysiology of pre-eclampsia. Antioxid. 2023;12(4):830. doi:10.3390/antiox12040830. [Google Scholar] [PubMed] [CrossRef]
9. Pohl R, Feder S, Haberl EM, Rein-Fischboeck L, Weiss TS, Spirk M, et al. Chemerin overexpression in the liver protects against inflammation in experimental non-alcoholic steatohepatitis. Biomedicines. 2022;10(1):132. doi:10.3390/biomedicines10010132. [Google Scholar] [PubMed] [CrossRef]
10. Lavis P, Bondue B, Cardozo AK. The dual role of chemerin in lung diseases. Cells. 2024;13(2):171. doi:10.3390/cells13020171. [Google Scholar] [PubMed] [CrossRef]
11. Kennedy AJ, Davenport AP. International union of basic and clinical pharmacology CIII: chemerin receptors CMKLR1 (Chemerin1) and GPR1 (Chemerin2) nomenclature, pharmacology, and function. Pharmacol Rev. 2018;70(1):174–96. doi:10.1124/pr.116.013177. [Google Scholar] [PubMed] [CrossRef]
12. Liu H, Xiong W, Liu Q, Zhang J, Dong S. Chemokine-like receptor 1 regulates the proliferation and migration of vascular smooth muscle cells. Med Sci Monit. 2016;22:4054–61. doi:10.12659/MSM.897832. [Google Scholar] [PubMed] [CrossRef]
13. Pachynski RK, Wang P, Salazar N, Zheng Y, Nease L, Rosalez J, et al. Chemerin suppresses breast cancer growth by recruiting immune effector cells into the tumor microenvironment. Front Immunol. 2019;10:983. doi:10.3389/fimmu.2019.00983. [Google Scholar] [PubMed] [CrossRef]
14. Rennier K, Shin WJ, Krug E, Virdi G, Pachynski RK. Chemerin reactivates PTEN and suppresses PD-L1 in tumor cells via modulation of a novel CMKLR1-mediated signaling cascade. Clin Cancer Res. 2020;26(18):5019–35. doi:10.1158/1078-0432.CCR-19-4245. [Google Scholar] [PubMed] [CrossRef]
15. Ernst MC, Sinal CJ. Chemerin: at the crossroads of inflammation and obesity. Trends Endocrinol Metab. 2010;21(11):660–7. doi:10.1016/j.tem.2010.08.001. [Google Scholar] [PubMed] [CrossRef]
16. Léniz A, González M, Besné I, Carr-Ugarte H, Gómez-García I, Portillo MP. Role of chemerin in the control of glucose homeostasis. Mol Cell Endocrinol. 2022;541:111504. doi:10.1016/j.mce.2021.111504. [Google Scholar] [PubMed] [CrossRef]
17. Nakamura N, Naruse K, Kobayashi Y, Miyabe M, Saiki T, Enomoto A, et al. Chemerin promotes angiogenesis in vivo. Physiol Rep. 2018;6(24):e13962. doi:10.14814/phy2.13962. [Google Scholar] [PubMed] [CrossRef]
18. Ben Dhaou C, Mandi K, Frye M, Acheampong A, Radi A, de Becker B, et al. Chemerin regulates normal angiogenesis and hypoxia-driven neovascularization. Angiogenes. 2022;25(2159–79. doi:10.1007/s10456-021-09818-1. [Google Scholar] [PubMed] [CrossRef]
19. Zhang Y, Shen WJ, Qiu S, Yang P, Dempsey G, Zhao L, et al. Chemerin regulates formation and function of brown adipose tissue: ablation results in increased insulin resistance with high fat challenge and aging. Faseb J. 2021;35(7):e21687. [Google Scholar] [PubMed]
20. Banas M, Zabieglo K, Kasetty G, Kapinska-Mrowiecka M, Borowczyk J, Drukala J, et al. Chemerin is an antimicrobial agent in human epidermis. PLoS One. 2013;8(3):e58709. doi:10.1371/journal.pone.0058709. [Google Scholar] [PubMed] [CrossRef]
21. Godlewska U, Bilska B, Zegar A, Brzoza P, Borek A, Murzyn K, et al. The antimicrobial activity of chemerin-derived peptide p4 requires oxidative conditions. J Biol Chem. 2019;294(4):1267–78. doi:10.1074/jbc.RA118.005495. [Google Scholar] [PubMed] [CrossRef]
22. Li J, Lu Y, Li N, Li P, Wang Z, Ting W, et al. Chemerin: a potential regulator of inflammation and metabolism for chronic obstructive pulmonary disease and pulmonary rehabilitation. Biomed Res Int. 2020;2020:4574509. [Google Scholar] [PubMed]
23. Gonzalez-Ponce F, Gamez-Nava JI, Perez-Guerrero EE, Saldaña-Cruz AM, Vazquez-Villegas ML, Ponce-Guarneros JM, et al. Serum chemerin levels: a potential biomarker of joint inflammation in women with rheumatoid arthritis. PLoS One. 2021;16(9):e0255854. doi:10.1371/journal.pone.0255854. [Google Scholar] [PubMed] [CrossRef]
24. Jacenik D, Fichna J. Chemerin in immune response and gastrointestinal pathophysiology. Clin Chim Acta. 2020;504:146–53. doi:10.1016/j.cca.2020.02.008. [Google Scholar] [PubMed] [CrossRef]
25. Hu B, Song W, Tang Y, Shi M, Li H, Yu D. Induction of chemerin on autophagy and apoptosis in dairy cow mammary epithelial cells. Anim. 2019;9(10):848. [Google Scholar]
26. Makowczenko KG, Jastrzebski JP, Paukszto L, Dobrzyn K, Kiezun M, Smolinska N, et al. Chemerin impact on alternative mRNA transcription in the porcine luteal cells. Cells. 2022;11(4):715. doi:10.3390/cells11040715. [Google Scholar] [PubMed] [CrossRef]
27. Xie Y, Huang Y, Ling X, Qin H, Wang M, Luo B. Chemerin/CMKLR1 axis promotes inflammation and pyroptosis by activating NLRP3 inflammasome in diabetic cardiomyopathy rat. Front Physiol. 2020;11:381. doi:10.3389/fphys.2020.00381. [Google Scholar] [PubMed] [CrossRef]
28. Tkachenko A. Apoptosis and eryptosis: similarities and differences. Apoptosis. 2024;29(3–4):482–502. doi:10.1007/s10495-023-01915-4. [Google Scholar] [PubMed] [CrossRef]
29. Bissinger R, Bhuyan AAM, Qadri SM, Lang F. Oxidative stress, eryptosis and anemia: a pivotal mechanistic nexus in systemic diseases. Febs J. 2019;286(5):826–54. doi:10.1111/febs.14606. [Google Scholar] [PubMed] [CrossRef]
30. Restivo I, Attanzio A, Tesoriere L, Allegra M. Suicidal erythrocyte death in metabolic syndrome. Antioxid. 2021;10(2):154. doi:10.3390/antiox10020154. [Google Scholar] [PubMed] [CrossRef]
31. Onishchenko AI, Prokopiuk VY, Chumachenko VA, Virych PA, Tryfonyuk LY, Kutsevol NV, et al. Hemocompatibility of dextran-graft-polyacrylamide/zinc oxide nanosystems: hemoly-sis or eryptosis? Nanotechnology. 2023;35(3):035102. [Google Scholar]
32. Alfhili MA, Alsalmi E, Aljedai A, Alsughayyir J, Abudawood M, Basudan AM. Calcium-oxidative stress signaling axis and casein kinase 1α mediate eryptosis and hemolysis elicited by novel p53 agonist inauhzin. J Chemother. 2022;34(4):247–57. doi:10.1080/1120009X.2021.1963616. [Google Scholar] [PubMed] [CrossRef]
33. Zangeneh AR, Takhshid MA, Ranjbaran R, Maleknia M, Meshkibaf MH. Diverse effect of vitamin C and N-acetylcysteine on aluminum-induced eryptosis. Biochem Res Int. 2021;2021:6670656. [Google Scholar] [PubMed]
34. Klymchenko AS. Fluorescent probes for lipid membranes: from the cell surface to organelles. Accounts Chem Res. 2023;56(1):1–12. doi:10.1021/acs.accounts.2c00586. [Google Scholar] [PubMed] [CrossRef]
35. Pyrshev KA, Yesylevskyy SO, Mély Y, Demchenko AP, Klymchenko AS. Caspase-3 activation decreases lipid order in the outer plasma membrane leaflet during apoptosis: a fluorescent probe study. Biochim Biophys Acta Biomembr. 2017;1859(10):2123–32. doi:10.1016/j.bbamem.2017.08.002. [Google Scholar] [PubMed] [CrossRef]
36. Lang KS, Lang PA, Bauer C, Duranton C, Wieder T, Huber SM, et al. Mechanisms of suicidal erythrocyte death. Cell Physiol Biochem. 2005;15(5):195–202. doi:10.1159/000086406. [Google Scholar] [PubMed] [CrossRef]
37. Dreischer P, Duszenko M, Stein J, Wieder T. Eryptosis: programmed death of nucleus-free, iron-filled blood cells. Cells. 2022;11(3):503. doi:10.3390/cells11030503. [Google Scholar] [PubMed] [CrossRef]
38. Fink M, Bhuyan AAM, Nürnberg B, Faggio C, Lang F. Triggering of eryptosis, the suicidal erythrocyte death, by phenoxodiol. Naunyn Schmiedebergs Arch Pharmacol. 2019;392(10):1311–8. doi:10.1007/s00210-019-01681-8. [Google Scholar] [PubMed] [CrossRef]
39. Ferland DJ, Darios ES, Neubig RR, Sjögren B, Truong N, Torres R, et al. Chemerin-induced arterial contraction is Gi-and calcium-dependent. Vascul Pharmacol. 2017;88:30–41. doi:10.1016/j.vph.2016.11.009. [Google Scholar] [PubMed] [CrossRef]
40. Matarrese P, Straface E, Pietraforte D, Gambardella L, Vona R, Maccaglia A, et al. Peroxynitrite induces senescence and apoptosis of red blood cells through the activation of aspartyl and cysteinyl proteases. Faseb J. 2005;19(3):416–8. [Google Scholar] [PubMed]
41. Attanzio A, Frazzitta A, Cilla A, Livrea MA, Tesoriere L, Allegra M. 7-Keto-Cholesterol and Cholestan-3beta, 5alpha, 6beta-triol induce eryptosis through distinct pathways leading to NADPH oxidase and nitric oxide synthase activation. Cell Physiol Biochem. 2019;53(6):933–47. doi:10.33594/000000018. [Google Scholar] [CrossRef]
42. Azarov I, Huang KT, Basu S, Gladwin MT, Hogg N, Kim-Shapiro DB. Nitric oxide scavenging by red blood cells as a function of hematocrit and oxygenation. J Biol Chem. 2005;280(47):39024–32. doi:10.1074/jbc.M509045200. [Google Scholar] [PubMed] [CrossRef]
43. Watts SW. Trash talk by fat: chemerin as a reactive oxygen species provocateur in the vasculature. Hypertens. 2015;66(3):466–8. doi:10.1161/HYPERTENSIONAHA.115.05738. [Google Scholar] [PubMed] [CrossRef]
44. Wang Y, Huo J, Zhang D, Hu G, Zhang Y. Chemerin/ChemR23 axis triggers an inflammatory response in keratinocytes through ROS-sirt1-NF-κB signaling. J Cell Biochem. 2019;120(4):6459–70. doi:10.1002/jcb.v27936. [Google Scholar] [CrossRef]
45. Xie Q, Deng Y, Huang C, Liu P, Yang Y, Shen W, et al. Chemerin-induced mitochondrial dysfunction in skeletal muscle. J Cell Mol Med. 2015;19(5):986–95. doi:10.1111/jcmm.2015.19.issue-5. [Google Scholar] [CrossRef]
46. Neves KB, Nguyen Dinh Cat A, Lopes RA, Rios FJ, Anagnostopoulou A, Lobato NS, et al. Chemerin regulates crosstalk between adipocytes and vascular cells through nox. Hypertens. 2015;66(3):657–66. doi:10.1161/HYPERTENSIONAHA.115.05616. [Google Scholar] [PubMed] [CrossRef]
47. Repsold L, Joubert AM. Eryptosis: an erythrocyte’s suicidal type of cell death. Biomed Res Int. 2018;2018:9405617. [Google Scholar] [PubMed]
48. Fang M, Xia F, Chen Y, Shen Y, Ma L, You C, et al. Role of eryptosis in hemorrhagic stroke. Front Mol Neurosci. 2022;15:932931. doi:10.3389/fnmol.2022.932931. [Google Scholar] [PubMed] [CrossRef]
49. Turpin C, Meilhac O, Bourdon E, Canonne-Hergaux F, Rondeau P. Methodologies and tools to shed light on erythrophagocytosis. Biochimie. 2022;202:166–79. doi:10.1016/j.biochi.2022.07.017. [Google Scholar] [PubMed] [CrossRef]
50. Tkachenko A, Onishchenko A. Casein kinase 1α mediates eryptosis: a review. Apoptosis. 2023;28(1–2):1–19. [Google Scholar] [PubMed]
51. Dias GF, Grobe N, Rogg S, Jörg DJ, Pecoits-Filho R, Moreno-Amaral AN, et al. The role of eryptosis in the pathogenesis of renal anemia: insights from basic research and mathematical modeling. Front Cell Dev Biol. 2020;8:598148. doi:10.3389/fcell.2020.598148. [Google Scholar] [PubMed] [CrossRef]
52. Kort DH, Kostolias A, Sullivan C, Lobo RA. Chemerin as a marker of body fat and insulin resistance in women with polycystic ovary syndrome. Gynecol Endocrinol. 2015;31(2):152–5. doi:10.3109/09513590.2014.968547. [Google Scholar] [PubMed] [CrossRef]
53. Yang S, Wang Q, Huang W, Song Y, Feng G, Zhou L, et al. Are serum chemerin levels different between obese and non-obese polycystic ovary syndrome women? Gynecol Endocrinol. 2016;32(1):38–41. doi:10.3109/09513590.2015.1075501. [Google Scholar] [PubMed] [CrossRef]
54. Zhou Z, Chen H, Ju H, Sun M. Circulating chemerin levels and gestational diabetes mellitus: a systematic review and meta-analysis. Lipids Health Dis. 2018;17(1):169. doi:10.1186/s12944-018-0826-1. [Google Scholar] [PubMed] [CrossRef]
55. Alkady MM, Abdel-Messeih PL, Nosseir NM. Assessment of serum levels of the adipocytokine chemerin in colorectal cancer patients. J Med Biochem. 2018;37(3):313–9. doi:10.1515/jomb-2017-0062. [Google Scholar] [PubMed] [CrossRef]
56. Sochal M, Fichna J, Gabryelska A, Talar-Wojnarowska R, Białasiewicz P, Małecka-Wojciesko E. Serum levels of chemerin in patients with inflammatory bowel disease as an indicator of anti-TNF treatment efficacy. J Clin Med. 2021;10(19):4615. doi:10.3390/jcm10194615. [Google Scholar] [PubMed] [CrossRef]
57. Lang F, Bissinger R, Abed M, Artunc F. Eryptosis—The neglected cause of anemia in end stage renal disease. Kidney Blood Press Res. 2017;42(4):749–60. doi:10.1159/000484215. [Google Scholar] [PubMed] [CrossRef]
58. Zhao L, Yamaguchi Y, Ge X, Robinson WH, Morser J, Leung LLK. Chemerin 156F, generated by chymase cleavage of prochemerin, is elevated in joint fluids of arthritis patients. Arthritis Res Ther. 2018;20(1):132. doi:10.1186/s13075-018-1615-y. [Google Scholar] [PubMed] [CrossRef]
Cite This Article
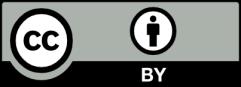
This work is licensed under a Creative Commons Attribution 4.0 International License , which permits unrestricted use, distribution, and reproduction in any medium, provided the original work is properly cited.