Open Access
REVIEW
Three-dimensional cell-based strategies for liver regeneration
1 Liver Transplant Center, Transplant Center, West China Hospital, Sichuan University, Chengdu, 610041, China
2 Frontiers Medical Center, Tianfu Jincheng Laboratory, Chengdu, 610212, China
* Corresponding Authors: XI XIA. Email: ; JIAN YANG. Email:
(This article belongs to the Special Issue: Perspectives on Stem Cells and Regenerative Medicine)
BIOCELL 2024, 48(7), 1023-1036. https://doi.org/10.32604/biocell.2024.051095
Received 27 February 2024; Accepted 28 March 2024; Issue published 03 July 2024
Abstract
Liver regeneration and the development of effective therapies for liver failure remain formidable challenges in modern medicine. In recent years, the utilization of 3D cell-based strategies has emerged as a promising approach for addressing these urgent clinical requirements. This review provides a thorough analysis of the application of 3D cell-based approaches to liver regeneration and their potential impact on patients with end-stage liver failure. Here, we discuss various 3D culture models that incorporate hepatocytes and stem cells to restore liver function and ameliorate the consequences of liver failure. Furthermore, we explored the challenges in transitioning these innovative strategies from preclinical studies to clinical applications. The collective insights presented herein highlight the significance of 3D cell-based strategies as a transformative paradigm for liver regeneration and improved patient care.Keywords
The liver is a crucial organ in the human body that performs various essential functions for maintaining overall health and well-being. However, despite its importance, the liver is highly susceptible to damage and disease [1]. Factors such as drugs, aging, inflammation, fibrosis, and cirrhosis can impair liver regeneration and cause irreversible liver failure [2,3]. For patients with end-stage liver failure, the only definitive treatment is orthotopic liver transplantation (OLT), which has been the only therapeutic option since 1983. Nevertheless, the demand for OLT consistently outpaces the available supply of donor organs, leading to post-operative complications such as thrombosis, biliary disorders, graft rejection, and elevated morbidity and mortality rates among patients [4,5]. Therefore, much hope has shifted to alternative therapies that can prevent or reverse liver failure.
Over the years, researchers and medical professionals have explored various strategies to address this condition. These approaches have been extensively examined in previous reviews [6–8], In Fig. 1, we provide a concise overview of the advantages and drawbacks of these strategies leading up to the era of 3D cell technologies. Subsequently, we present current 3D cell-based technologies for liver regeneration, as well as the challenges and future perspectives of using 3D cell-based strategies for liver therapy.
Figure 1: Advantages and drawbacks of liver therapy and regeneration approaches prior to the emergence of 3D cell technologies. The strategies include orthotopic liver transplantation, artificial and bio-artificial liver devices, cell transplantation, decellularized liver scaffolds.
Liver Disease Requiring Regenerative Therapy
The liver possesses an extraordinary capacity to regenerate after injury, however, this capacity can be overwhelmed by a wide range of liver diseases, typically categorized as chronic liver diseases and acute liver failure. Chronic liver diseases such as non-alcoholic fatty liver disease (NAFLD), alcoholic liver disease, viral hepatitis, and genetic disorders may induce persistent hepatic injury, leading to liver fibrosis. Liver-related mortality escalates significantly with advancing fibrosis [9], paving the way for the development of severe liver cirrhosis or hepatocellular carcinoma, ultimately culminating in end-stage liver failure [10]. In recent years, acute-on-chronic liver failure (ACLF) in hospitalized patients with chronic liver disease has been increasing globally, leading to a significant rise in mortality rates [11]. Furthermore, acute liver failure induced by drugs, toxins, and other factors may progress to end-stage liver failure. In such cases, regenerative therapy holds the potential to facilitate the restoration of liver function.
Cell Sources for 3D Cell Cultures
Hepatocytes, as the principal functional entities of the liver, comprising a substantial proportion of the liver mass, representing up to 80%. They possess a repertoire of functional characteristics reflective of the liver and can be transplanted into recipients for the restoration of hepatic function, obviating the need for intricate surgical procedures [12,13]. Despite being widely regarded as the gold standard in research, primary hepatocytes in 2D cultures possess drawbacks that significantly restrict their utilization in clinical applications, as their susceptibility to loss of viability, and function during in vitro cultivation or subsequent cryopreservation. Although some scientists have made successful attempts in long-term cultivation of hepatocytes in 3D culture environments [14,15], a consistent cell source remains elusive primarily due to the scarcity of available donor livers. Therefore, it is essential to define and validate novel sources of functional hepatic cells (Fig. 2).
Figure 2: Schematic representation of various cell sources in 3D cell cultures for liver therapy and regeneration. Primary hepatocytes can be converted to liver progenitor-like cells to enable expansion, while stem cells can be isolated, reprogrammed, and differentiated into hepatic cells. HSCs: hepatic stellate cells; HPCs: hepatic progenitor cells; UCFTs: human umbilical cord fibroblasts; hiHeps: Human-induced hepatocytes; MSCs: mesenchymal stem cells; iPSCs: induced pluripotent stem cells; ESCs: embryonic stem cells.
To address the scarcity of hepatocytes, researchers are actively engaged in the pursuit of generating functional hepatocytes from diverse stem cell populations, including mesenchymal stem cells (MSCs), embryonic stem cells (ESCs), and induced pluripotent stem cells (iPSCs) [16].
MSCs represent a multipotent type of stem cells that can be obtained from a variety of tissues. They express major histocompatibility complex class I (MHC-I) and class II (MHC-II) molecules, making them well-suited for allogeneic transplantation without causing rejection [17,18], in addition to their immunomodulatory properties [19–21], making them highly suitable for transplantation purposes. The mechanisms underlying the therapeutic effects mediated by MSCs involves their differentiation into multiple cell lineages, migration towards damaged tissues, immunomodulatory actions, and a wide array of biologically active factors, including cytokines, chemokines, hormones, growth factors, and miRNAs that promote cell survival and proliferation [22,23]. However, the constrained migration and suboptimal viability of transplanted MSCs [24,25] have prompted investigators to delve into alternative stem cell sources for the purpose of liver regeneration.
ESCs originate from the inner cell mass of a blastocyst that possess unique pluripotency and self-renewal capabilities [26]. These cells possess the exceptional capability of undergoing indefinite division in vitro while retaining their potential to differentiate into all types of cells present in an adult body [27,28]. Hence, ESCs hold the potential to offer an inexhaustible supply of transplantable cells to replace or regenerate damaged tissue. Although the clinical application of ESCs for extensive expansion and differentiation into hepatocytes remains a significant challenge owing to lingering uncertainties concerning their acquisition and potential tumorigenic risks, scientists have developed several effective strategies, such as growing ESCs in 3D fibrous matrices or organoids system [29,30]. Another concern is that ESCs are unfortunately mired by ethical controversies.
iPSCs have been demonstrated to exhibit remarkable similarity to ESCs in regard to their transcriptional programs, chromatin modification profiles and overall chromatin configurations [31–33]. As iPSCs can be derived directly from adult tissues, they present the advantage of bypassing the need for embryo destruction and can be personalized for each patient. The advent of iPSCs has thus emerged as a focal point in the realm of stem cell research [34]. Numerous studies have documented the successful differentiation of iPSCs into diverse array of liver cell types, encompassing hepatocytes, endothelial cells, cholangiocytes, and stellate cells [35–37]. Concurrently, the growing fascination with self-assembling or matrix-guided 3D organoids has opened new avenues towards the development of functional bioartificial livers [38]. However, it is crucial to note that while initially believed to eliminate the risk of immune rejection in transplants, accumulating evidence suggests that certain iPSC-derived cells may still provoke immune responses [39,40].
Hepatic progenitor cells (HPCs) represent a class of bipotential cells that are renowned for their exceptional capacity to simultaneously proliferate and differentiate into both hepatocytes and cholangiocytes [41]. HPCs are regarded as a promising cellular reservoir for liver regeneration. Nevertheless, the clinical application of HPCs is impeded by the potential risk of hepatocellular carcinoma development [42,43]. Human-induced hepatocytes (hiHeps) are derived through the direct reprogramming of human fibroblasts. These hiHeps could be easily expanded and maintained hepatocyte-specific functions, including the secretion of plasma proteins and activity of P450 enzymes [44]. The hiHep-based bioartificial liver system demonstrated promising outcomes in both a porcine liver failure model and patients undergoing extended liver resection [45]. Hepatic stellate cells (HSCs) comprise 15% of all hepatocytes and play a pivotal role in the synthesis and secretion of collagen and other extracellular matrix components. HSCs orchestrate liver regeneration by producing a diverse array of growth factors, cytokines, and glycoproteins that are activated by proinflammatory cytokines and transformed into myofibroblasts [46]. Kupffer cells, referred to as hepatic macrophages, play a pivotal role as the liver’s primary immune defense against injurious particles and chemical agents that infiltrate the portal vein. Kupffer cells are responsible for releasing crucial bioactive factors for regulating hepatocytes [47,48].
Development and Application of 3D Cell-Based Strategies for Liver Regeneration
Over the past decades, the aforementioned stem or progenitor cells have exhibited the potential to undergo differentiation into hepatocyte progenitor-like cells or hepatocyte-like cells, thus presenting a potential avenue for treating liver diseases through both preclinical and clinical investigations [49–52]. Traditional two-dimensional (2D) cell cultures have provided crucial insights into liver biology, yet they often fail to accurately replicate the intricate spatial and biochemical cues present in the native liver microenvironment [53]. In contrast, 3D cell-based strategies have the ability to mimic crucial interactions between cell-cell and cell-matrix, leading to a transformative paradigm shift as they successfully replicate the dynamic complexity of liver tissue. The integration of primary hepatocytes, stem cells, and innovative culture techniques provides novel opportunities to restore liver function and improve patient outcomes.
Spheroids, which represent dense 3D cell aggregates, serve as optimal building blocks for regenerative medicine. Given their capacity to facilitate tissue formation, spheroids hold potential applications in areas such as wound healing or correcting congenital defects [54]. Approaches to guide spheroid behavior can be categorized as ex vivo or in situ [55]. Ex vivo manipulation of spheroids typically begins during the monolayer culture phase prior to spheroid formation. During this stage, cells undergo priming through exposure to pharmacological agents or other soluble bioactive factors, while the modulation of microenvironmental factors, like cell confluency and localized oxygen levels, can prompt differentiation and enhance cell survival. In situ instruction of spheroids often experience limited viability, swift dedifferentiation, and propensity for migration from the injection site [56]. Biomaterials play a pivotal role in facilitating the localization of cells at the targeted delivery site, while also providing instructive cues to regulate and guide cellular behavior. These biomaterials can be customized to emulate the characteristics of the extracellular matrix (ECM), thereby eliciting stimulation of cell proliferation and enhancement of cell survival. This allows for quicker delivery of therapies and conservation of valuable resources [57].
In preclinical studies using animal models, researchers have explored the prospective capacity of spheroids to amplify and potentiate regenerative processes within the liver. Sun et al. demonstrated that spheroids of human umbilical cord-derived MSCs (hUC-MSCs) exhibited enhanced migratory capabilities towards the injured liver when contrasted with hUC-MSCs cultured in a two-dimensional (2D) manner. Notably, these hUC-MSCs were found to effectively stimulate liver regeneration and facilitate repair in mice afflicted by liver injury [58]. Hepatocyte spheroids of human were effectively engrafted into the livers of mice with viral hepatitis following intrasplenic injection [59]. Collagen fiber-based 3D spheroids comprising adipose-derived stem cells effectively maintained cellular functionality and the capacity for paracrine secretion. Furthermore, transplantation of these spheroids mitigated thioacetamide (TAA)-induced liver cirrhosis in murine models [60]. Spheroids derived from stem cells of human exfoliated deciduous teeth were successfully grafted into the livers of mice afflicted with carbon tetrachloride (CCl4)-induced liver fibrosis. In this transplantation, the spheroids engrafted well, leading to enhanced liver function, and demonstrating antifibrotic efficacy in mice [61]. Innovatively, some researchers have utilized 3D printing to construct hepatocyte spheroids, thereby fabricating functional liver tissue that replicates intricate duct and sinusoid microanatomy [62,63]. Zhang et al. devised a method for fabricating spheroids with precise quantities of distinct cell types using microfluidic flow cytometric printing. These precision spheroids exhibit enhanced variability and functional consistency in comparison to randomly formed spheroids [64].
Organoids represent a condensed and simplified rendition of an organ, often originating from stem cells. They may encompass diverse cell types that spontaneously self-organize in vitro, recapitulating the morphology and intricate biological interplays within a living organism [65]. Here, we elucidate the recent endeavors directed toward constructing next-generation organoids and outline the current potential of utilizing mature organoids in liver regeneration.
ESCs and iPSCs are preferred candidates for liver organoid generation due to their proliferation and differentiation characteristics. For instance, Wang et al. presented a study detailing the generation of hepatic organoids derived from ESCs with expandable attributes, utilizing entirely defined medium devoid of serum and feeder cells. Remarkably, these organoids were capable of expanding for up to 20 passages, facilitating large-scale growth. Furthermore, upon transplantation, they exhibit a notable capacity for repopulating injured livers within Fah-/-/Rag2-/-/Il2rg-/- (FRG) mice, subsequently differentiating into mature hepatocytes in vivo [66]. In 2022, Messina and colleagues successfully developed human iPSC-derived hepatocytes organoids. Specifically, the process involved the initial differentiation of human iPSCs into hepatoblasts through the application of growth factors and cytokines. Subsequently, hepatoblasts were developed into iPSC-derived hepatocytes organoids using hepatic growth factor (HGF), oncostatin M, vitamin K and dexamethasone [67].
There are some parameters that are crucial to control during the construction of 3D organoids. Firstly, liver organoids require exposure to specific growth factors, including Wnt, fibroblast growth factor, HGF, and bone morphogenetic protein, at precise timings to effectively promote hepatic progenitor survival and their differentiation [68]. The ECM also plays a critical role in the formation of organoids. While Matrigel has been widely employed for organoid culture, its application is limited by factors like complexity, batch-to-batch variability, and its origin from mouse tumor cells. Consequently, various alternatives are under exploration, such as decellularized liver ECM, diverse synthetic hydrogels, as well as peptide and recombinant protein matrices [69]. Lastly, designed geometries like micropatterning or microfluid dynamics represent strategies that can be harnessed to facilitate the growth of cells and organoids [70].
Co-culture of the liver organoids
Employing a single cell type in organoids culture ensures the proliferation and self-organization of a homogenous cellular population, thereby simplifying the formation process. However, co-culturing multiple cell types, including endothelial cells, HSCs and MSCs can significantly enhance the functional maturity and complexity of the organoids, leading to a better mimicry of the liver organ structure [71,72]. In 2013, Takebe et al. made the pioneering report of generating a three-dimensional vascularized and functionally active human liver using human iPSCs. Specifically, they demonstrated that iPSCs, co-cultured alongside MSCs and human umbilical vein endothelial cells (HUVECs), could spontaneously self-organize into three-dimensional iPSC-liver buds (organoids) which exhibited an endothelial network and expressed hepatic-specific marker genes [71]. In 2019, Pettinato et al. interlaced iPSCs with human adipose microvascular endothelial cells, resulting in an increased differentiation yield and significant enhancements across a diverse spectrum of hepatic functions [73]. In 2021, Tanimizu et al. generated a functional hepatobiliary tubular organoid through the co-cultivation of mouse hepatocyte progenitors and cholangiocytes. Remarkably, this organoid exhibited the acquisition and sustenance of metabolic functions, including the secretion of albumin and activities of cytochrome P450, persisting over an extended duration [74] (Fig. 3).
Figure 3: Generation of hepatic organoids for transplantation. In single cell type culture, stem cells were differentiated in the presence of several factors like wnt, FGF, HGF and BMP, and developed into hepatic organoids using HGF, OSM, vitamin K and Dex. In multi-cell type culture, co-culturing multiple cell types, including stem cell-derived hepatic cells, and endothelial cells, can significantly enhance the functional maturity and complexity of the organoids. FGF: fibroblast growth factor; HGF: hepatic growth factor; BMP: bone morphogenetic protein; ECM: extracellular matrix; Vit K: vitamin K; OSM: oncostatin M; DeX: dexamethasone; HUVEC: human umbilical vein endothelial cells.
Applications of organoids in regenerative medicine
Principally, organoids serve as valuable exploration tools, acting as platforms for drug screening and providing insights into the intricate processes underlying human development and diseases [75,76]. For regenerative medicine, the ultimate objective is to achieve the transplantation of tissue-specific organoids, which will facilitate the recovery or enhancement of tissue function. Despite the extensive exploration of regenerative medicine for liver diseases over numerous decades, several challenges persist. Transplanted organoids must exhibit long-term survival, compatibility with extensive expansion, and genetic stability [77].
Several studies have demonstrated that organoids transplantation is a promising strategy for cell-based therapy for liver regeneration (Table 1). Huch et al. established a durable human liver organoid culture, and the cells exhibit the capability to be readily transformed into functional hepatocytes both in vitro and upon transplantation in vivo [78]. Tsuchida et al. verified the safe transplantation of human iPSC-derived liver organoids via the portal vein, with concurrent ligation of the ductus venosus [52]. Sampaziotis et al. delivered remarkable evidence, showcasing the potential of cholangiocyte organoids to repair human biliary epithelium. This groundbreaking work introduces a therapeutic 3D stem cell-based approach for repairing ischemic bile ducts, potentially providing the potential for organoid-based therapies targeting various cholangiopathies [79]. Furthermore, organoids can be derived from patients with genetic diseases, and subsequently, genetically modified organoids hold the potential for transplantation into patients to alleviate disease phenotypes [80]. An illustrative instance of this strategy involves the application of Retinitis Pigmentosa GTPase Regulator (RPGR) gene-edited organoids in tackling retinitis pigmentosa. This strategy has demonstrated the restoration of defects characteristic of the disease, such as photoreceptor reduction [81]. Here, we summarized the typical studies that performing human liver organoid transplantation in the past decade (Table 1).
Bioscaffolds can mimic the ECM environment of the organ and can effectively induce cell growth and differentiation. As a result, these bioscaffolds possess the capacity to adequately support the colonization of host cells, meeting up the requirements of regeneration and repair. In general, bioscaffolds can be classified into two main groups: natural and synthetic. Natural bioscaffolds, such as collagen, chitosan, silk fibroin, or Polyhydroxyalkanoates (PHAs) are derived from biological sources and demonstrate exceptional biocompatibility and bioactivity. On the other hand, synthetic bioscaffolds, which include polymers like polylactic acid (PLA), polyglycolic acid (PGA), or polycaprolactone (PCL), are artificially produced, allowing for precise manipulation of their physical and chemical characteristics [99–101]. Here, we delve into a discussion of two representative bioscaffolds used in the field of liver regeneration.
3D encapsulation of cells in a collagen hydrogel has shown potential for enhancing maturation compared to 2D culture. Hussein et al. demonstrated that the combining of 3D techniques, namely guided aggregation and microencapsulation, with liver differentiation protocols, constitutes a robust strategy for producing fully mature and functional hepatocytes. This innovative approach presents a sustainable and limitless source of hepatocytes [102]. Song et al. successfully engrafted iPSC-derived hepatocyte-like cells (iPS-H) with stromal cells into immunocompetent mice. This accomplishment was realized through the encapsulation of the cells within biocompatible hydrogel capsules. The secretion of human albumin and α1-antitrypsin by encapsulated iPS-H/stromal cell aggregates attained a level comparable to the primary human hepatocyte/stromal cell control [83].
However, achieving a complete recapitulation of the entire biochemical and architectural intricacies of the natural microenvironment remains a challenge. Decellularized scaffolds from xenogeneic animals and discarded human livers offers a promising approach to tackle this issue. Decellularized liver scaffolds can retain the functional attributes of the native microvascular and bile drainage networks within the liver, along with the essential growth factors required for both angiogenesis and liver regeneration [103]. The techniques for producing decellularized liver scaffolds encompass physical, chemical, and enzymatic treatments, often employed in combination. Currently, substantial strides have been taken towards ensuring safety and clinical applicability. Detailed reviews are available elsewhere [104,105]. The next critical steps are the recellularization of the parenchyma and reendothelialization of the vascular lumen to generate transplantable and functional liver grafts. In the past decade, various proof-of-concept studies have been conducted to assess the viability of repopulation of liver grafts in both small and large animal models (Table 2).
3D bioprinting, a technique that employ computer-aided processes to precisely assemble cells and biomaterials into precise 3D structures, has emerged as a promising avenue for replicating hepatic tissue and facilitating translational endeavors. Bioprinting can be broadly classified as extrusion-based, inkjet-based, or light-assisted bioprinting. Notably, 3D-bioprinted liver models are commonly classified as either static scaffold-based models or dynamic liver-on-chip models [116]. Diverse forms of scaffolds featuring intricate structures have been designed for the exploration of liver regeneration. Kang et al. utilized a preset extrusion bioprinting technique to generate multicellular and multi-material structures comprising hepatic cells, endothelial cells, along with a lumen. This construct, comprising hepatic lobules within a densely vascularized framework, facilitated the fabrication of tissue at both micro-and macro-scales (Fig. 4). Such an approach can be pivotal in developing expansive 3D tissue constructs for multiscale tissue engineering purposes [117]. In a recent study, 3D-bioprinting was employed to construct hepatorganoids using HepaRG cells and bioink. Following transplantation into Fah-deficient mice, these hepatic organoids exhibited the formation of functional vascular systems, which consequently enhanced material transport and liver function. Consequently, the survival rate of the mouse model afflicted with liver injury exhibited significant improvement [118].
Figure 4: Schematic summarizing bioscaffolds and bioprinting strategies for liver therapy and regeneration. 3D encapsulation of cells within biocompatible hydrogel capsules has shown potential for enhancing maturation compared to 2D culture. Decellularized scaffolds from xenogeneic animals and discarded human livers offers a promising approach to recapitulate the microenvironment. 3D-bioprinted liver models, especially liver-on-chip, offers the capacity to recreate more intricate microenvironments like temperature, pH, cell shear stress and waste removal.
Unlike conventional static platforms, the integration of bioprinting with dynamic microfluidic liver chips offers the capacity to recreate intricate microenvironments encompassing factors like temperature, pH, cell shear stress, nutrient supply, oxygen gradient, and waste removal. Although the primary focus of research in the field of liver-on-chip technology is directed towards the application of these platforms for drug screening and disease modeling, a recent study introduced a novel microfluidic liver system that integrates hiPSCs-derived hepatocytes-laden microparticles and semipermeable microtubes. This system exhibited high cell viability, functional regeneration, and effective circulation system. Building upon these merits, a liver chip integrated with multiple microfluidic liver systems was employed in a rabbit model of acute liver failure. This intervention not only mitigated inflammation, but also facilitated the production of serum proteins, ultimately leading to improved survival rates [119].
In recent years, numerous clinical trials focusing on liver transplantation using 2D stem cells have emerged. Concurrently, there are also an increasing number of clinical studies on the application of organoids or liver-on-chips for predicting treatment response. However, to our knowledge, neither organoids nor 3D bioprinted liver constructs have been implemented in clinical trials for liver transplantation. Recent years have witnessed great advances in the application of 3D cell-based strategies for liver regeneration in animal models. A plethora of studies have affirmed the superiority of 3D cell culture over the traditional 2D approach [120]. Consequently, there exists promising potential in the utilization of spheroids, organoids, and 3D liver constructs for advancing the treatment of liver failure. The critical obstacles impede translation from laboratory innovations to clinical reality are discussed blow (Fig. 5).
Figure 5: Challenges of 3D cell-based strategies for liver therapy and regeneration, from laboratory innovations to clinical reality. It is imperative for researchers to substantiate the safety and efficacy of these therapies through comprehensive preclinical studies, rigorous safety evaluation and well-designed clinical trials.
Scale-up and manufacturing challenges
Transitioning from small-scale laboratory experiments to large-scale production of functional liver tissue constructs presents a substantial manufacturing challenge. Consistency in quality, reproducibility, and scalability is essential for guaranteeing the safety and effectiveness of liver constructs on a human scale. For spheroids or organoids, culture conditions that seamlessly integrate with the large-scale manufacturing platforms need to be developed. Indeed, the commonly used Matrigel carries potential risks for tumorigenesis and immune reactions due to its mouse origin, and batch-to-batch variability further complicates its reliable usage. Synthetic hydrogels, like polyethylene glycol (PEG), offer enhanced reliability and safety. However, cells within these hydrogels often exhibit restricted liver functionality [121]. Thus, it is necessary to develop reliable and safety ECM for generating reproducible and functional organoids. Furthermore, ensuring adequate oxygenation and nutrient exchange throughout the entire tissue construct, as well as the complex microenvironment and metabolic demands, are crucial for the viability and functionality of liver constructs.
Cell sources and immunological challenges
The choice between allogeneic and autologous cell sources in clinical applications involves careful consideration of factors, such as immune compatibility, treatment timeline, availability, and ethical considerations. In cases of patients with acute liver failure, the feasibility of utilizing autologous cells for readily available regenerative medicine solutions could be limited. Meanwhile, the use of allogeneic cells carries the inherent risk of inciting immune reactions within the host’s physiology, possibly culminating in graft rejection or analogous immune-mediated intricacies [122]. Genetic engineering techniques can be employed to manipulate allogeneic donor cells to diminish immunogenicity, presenting a promising avenue, albeit accompanied by a spectrum of inherent challenges [123].
Functional maturation and vascularization
Hepatocytes or stem cell-derived cells in 3D cultures often struggle to fully mature and maintain their functionality over time. For instance, the presence of unrelated cells or undifferentiated stem cells or within organoids can give rise to the subsequent development of undesirable and potentially hazardous tissue formations characterized by genetic abnormalities [124]. This issue highlights concerns regarding the safety and potential tumorigenicity associated with organoids. To address this challenge, the genetic stability of organoid cells should undergo regular check. The cells utilized for generating organoids should undergo purification by microdissection methods to ensure separation from their surrounding stromal components. Modulating the microenvironment composition, particularly by manipulating the presence of growth factors, can effectively guide the differentiation of organoids towards the desired pathways [125].
Vascularization is critical for the growth and efficient exchange of factors within organoids and liver constructs. Ensuring efficient vascularization within 3D liver tissue constructs remains a significant challenge. Although the vascularization of bioprinted liver scaffolds has been generated successfully in a few models, there remains a need for the development of advanced technologies that enable more precise bioprinting of small-diameter blood vessels. Currently, spheroids and organoids still encounter limitations in establishing proper vascularization upon engraftment. To address this, incorporating endothelial cells or HUVECs as organoid components may presents a promising strategy [71]. Exploring transplantation and cytokine induction methods for in vivo vascularization should receive more attention.
Ethical and regulatory considerations
Ethical concerns pertaining to the origin, potential hazards, and long-term consequences of transplanted liver grafts introduce intricate questions. Participants may face risks including tumorigenesis, adverse immunogenic responses, and infections [126]. Therefore, the progression of 3D cell-based liver regeneration therapies from laboratory breakthroughs to clinical implementation demands rigorous safety evaluations and regulatory approval. To attain regulatory approval and ensure patient welfare, it is imperative to substantiate the safety and efficacy of these therapies through well-designed clinical trials and comprehensive preclinical studies. At present, no established clinical or ethical guidelines are in place to evaluate the safety of 3D cell-based liver grafts for clinical trials. Researchers could gain insights from analogous fields like cell therapy, for addressing matters such as cell sourcing, risk evaluation, patient selection criteria, trial design and oversight.
Over the last decade, the evolution of 3D cell-based strategies for liver regeneration has been swift and substantial, bearing tremendous potential and breakthroughs in the realms of hepatology and regenerative medicine. As technology continues to evolve and our understanding of cellular interactions deepens, overcoming the aforementioned challenges is only a matter of time. The integration of multiple cell types within 3D constructs will mirror the intricate liver microenvironment, enhancing our understanding of liver diseases and tissue functionality. Biomimetic environments within these cultures will foster cell maturation and function, while breakthroughs in vascularization will ensure an efficient nutrient and oxygen supply. Advanced imaging and gene editing techniques will provide unprecedented insights and control, accelerating the development of safer and more effective treatments. With these advancements, the translation of 3D cell-based strategies into clinical trials will inch closer, potentially reshaping the landscape of liver disease treatment and regeneration.
Acknowledgement: None.
Funding Statement: This work was supported by grants from the Sichuan Science and Technology Program (2023NSFSC1877).
Author Contributions: The authors confirm contribution to the paper as follows: draft manuscript preparation: Dan Guo. Conceptualization, reviewing and editing the manuscript: Xi Xia. Supervision and funding acquisition: Jian Yang. All authors reviewed the results and approved the final version of the manuscript.
Availability of Data and Materials: Data sharing not applicable to this article as no datasets were generated or analyzed during the current study.
Ethics Approval: Not applicable.
Conflicts of Interest: The authors declare that they have no conflicts of interest to report regarding the present study.
References
1. Salete-Granado D, Carbonell C, Puertas-Miranda D, Vega-Rodriguez VJ, Garcia-Macia M, Herrero AB, et al. Autophagy, oxidative stress, and alcoholic liver disease: a systematic review and potential clinical applications. Antioxid. 2023;12(7):1425. [Google Scholar]
2. Clinton JW, Kiparizoska S, Aggarwal S, Woo S, Davis W, Lewis JH. Drug-induced liver injury: highlights and controversies in the recent literature. Drug Saf. 2021;44(11):1125–49. [Google Scholar] [PubMed]
3. Casulleras M, Zhang IW, Lopez-Vicario C, Claria J. Leukocytes, systemic inflammation and immunopathology in acute-on-chronic liver failure. Cells. 2020;9(12):2632. [Google Scholar] [PubMed]
4. Durkin C, Bittermann T. Liver transplantation for alcohol-associated hepatitis. Curr Opin Organ Transplant. 2023;28(2):85–94. [Google Scholar] [PubMed]
5. Liu H, Ashwat E, Humar A. Current status of living donor liver transplantation: impact, advantages, and challenges. Curr Gastroenterol Rep. 2023;25(10):225–31. [Google Scholar] [PubMed]
6. Messina A, Luce E, Hussein M, Dubart-Kupperschmitt A. Pluripotent-stem-cell-derived hepatic cells: hepatocytes and organoids for liver therapy and regeneration. Cells. 2020;9(2):420. [Google Scholar] [PubMed]
7. Morrison MA, Artru F, Trovato FM, Triantafyllou E, McPhail MJ. Potential therapies for acute-on-chronic liver failure. Liver Int. 2023;1–16. [Google Scholar]
8. Furuta T, Furuya K, Zheng YW, Oda T. Novel alternative transplantation therapy for orthotopic liver transplantation in liver failure: a systematic review. World J Transplant. 2020;10(3):64–78. [Google Scholar] [PubMed]
9. Chen L, Guo W, Mao C, Shen J, Wan M. Liver fibrosis: pathological features, clinical treatment and application of therapeutic nanoagents. J Mater Chem B. 2024;12(6):1446–66. [Google Scholar] [PubMed]
10. Huang DQ, Terrault NA, Tacke F, Gluud LL, Arrese M, Bugianesi E, et al. Global epidemiology of cirrhosis-aetiology, trends and predictions. Nat Rev Gastroenterol Hepatol. 2023;20(6):388–98. [Google Scholar] [PubMed]
11. Schulz M, Trebicka J. Acute-on-chronic liver failure: a global disease. Gut. 2022;71(1):5–6. doi:10.1136/gutjnl-2020-323973. [Google Scholar] [PubMed] [CrossRef]
12. Cernigliaro V, Peluso R, Zedda B, Silengo L, Tolosano E, Pellicano R, et al. Evolving cell-based and cell-free clinical strategies for treating severe human liver diseases. Cells. 2020;9(2):386. doi:10.3390/cells9020386. [Google Scholar] [PubMed] [CrossRef]
13. Chen HS, Joo DJ, Shaheen M, Li Y, Wang Y, Yang J, et al. Randomized trial of spheroid reservoir bioartificial liver in porcine model of posthepatectomy liver failure. Hepatol. 2019;69(1):329–42. doi:10.1002/hep.30184. [Google Scholar] [PubMed] [CrossRef]
14. Klaas M, Moll K, Maemets-Allas K, Loog M, Jarvekulg M, Jaks V. Long-term maintenance of functional primary human hepatocytes in 3D gelatin matrices produced by solution blow spinning. Sci Rep. 2021;11(1):20165. doi:10.1038/s41598-021-99659-1. [Google Scholar] [PubMed] [CrossRef]
15. Rose S, Ezan F, Cuvellier M, Bruyere A, Legagneux V, Langouet S, et al. Generation of proliferating human adult hepatocytes using optimized 3D culture conditions. Sci Rep. 2021;11(1):515. doi:10.1038/s41598-020-80019-4. [Google Scholar] [PubMed] [CrossRef]
16. Ang LT, Tan AKY, Autio MI, Goh SH, Choo SH, Lee KL, et al. A roadmap for human liver differentiation from pluripotent stem cells. Cell Rep. 2018;22(8):2190–205. [Google Scholar] [PubMed]
17. Zha S, Tay JC, Zhu S, Li Z, Du Z, Wang S. Generation of mesenchymal stromal cells with low immunogenicity from human PBMC-derived β2 Microglobulin Knockout induced Pluripotent stem cells. Cell Transplant. 2020;29:963689720965529. [Google Scholar] [PubMed]
18. Wu X, Jiang J, Gu Z, Zhang J, Chen Y, Liu X. Mesenchymal stromal cell therapies: immunomodulatory properties and clinical progress. Stem Cell Res Ther. 2020;11(1):345. [Google Scholar] [PubMed]
19. Muller L, Tunger A, Wobus M, von Bonin M, Towers R, Bornhauser M, et al. Immunomodulatory properties of mesenchymal stromal cells: an update. Front Cell Dev Biol. 2021;9:637725. [Google Scholar] [PubMed]
20. Zhou J, Shi Y. Mesenchymal stem/stromal cells (MSCsorigin, immune regulation, and clinical applications. Cell Mol Immunol. 2023;20(6):555–7. [Google Scholar] [PubMed]
21. Li H, Yu S, Chen L, Liu H, Shen C. Immunomodulatory role of mesenchymal stem cells in liver transplantation: status and prospects. Dig Dis. 2024;42(1):41–52. [Google Scholar] [PubMed]
22. Fan XL, Zhang Y, Li X, Fu QL. Mechanisms underlying the protective effects of mesenchymal stem cell-based therapy. Cell Mol Life Sci. 2020;77(14):2771–94. [Google Scholar] [PubMed]
23. Bagno LL, Salerno AG, Balkan W, Hare JM. Mechanism of action of mesenchymal stem cells (MSCsimpact of delivery method. Expert Opin Biol Ther. 2022;22(4):449–63. [Google Scholar] [PubMed]
24. Preda MB, Neculachi CA, Fenyo IM, Vacaru AM, Publik MA, Simionescu M, et al. Short lifespan of syngeneic transplanted MSC is a consequence of in vivo apoptosis and immune cell recruitment in mice. Cell Death Dis. 2021;12(6):566. [Google Scholar] [PubMed]
25. Guo L, Du J, Yuan DF, Zhang Y, Zhang S, Zhang HC, et al. Optimal H2O2 preconditioning to improve bone marrow mesenchymal stem cells’ engraftment in wound healing. Stem Cell Res Ther. 2020;11(1):434. [Google Scholar] [PubMed]
26. Liang G, Zhang Y. Embryonic stem cell and induced pluripotent stem cell: an epigenetic perspective. Cell Res. 2013;23(1):49–69. [Google Scholar] [PubMed]
27. Romito A, Cobellis G. Pluripotent stem cells: current understanding and future directions. Stem Cells Int. 2016;2016:9451492. [Google Scholar] [PubMed]
28. Chen G, Yin S, Zeng H, Li H, Wan X. Regulation of embryonic stem cell self-renewal. Life. 2022;12(8):1151. [Google Scholar] [PubMed]
29. Wuputra K, Ku CC, Wu DC, Lin YC, Saito S, Yokoyama KK. Prevention of tumor risk associated with the reprogramming of human pluripotent stem cells. J Exp Clin Cancer Res. 2020;39(1):100. [Google Scholar] [PubMed]
30. Kuboyama-Sasaki A, Takahashi Y, Xia C, Hiro K, Kobayashi T, Ohdan H, et al. Establishment of a cell culture platform for human liver organoids and its application for lipid metabolism research. Biotechnol J. 2024;19(1):e2300365. [Google Scholar] [PubMed]
31. Aboul-Soud MAM, Alzahrani AJ, Mahmoud A. Induced pluripotent stem cells (iPSCs)-roles in regenerative therapies, disease modelling and drug screening. Cells. 2021;10(9):2319. [Google Scholar] [PubMed]
32. Poetsch MS, Strano A, Guan K. Human induced pluripotent stem cells: from cell origin, genomic stability, and epigenetic memory to translational medicine. Stem Cells. 2022;40(6):546–55. [Google Scholar] [PubMed]
33. Edwards MM, Wang N, Massey DJ, Bhatele S, Egli D, Koren A. Incomplete reprogramming of DNA replication timing in induced pluripotent stem cells. Cell Rep. 2024;43(1):113664. [Google Scholar] [PubMed]
34. Hockemeyer D, Jaenisch R. Induced pluripotent stem cells meet genome editing. Cell Stem Cell. 2016;18(5):573–86. [Google Scholar] [PubMed]
35. Qiu S, Li Y, Imakura Y, Mima S, Hashita T, Iwao T, et al. An efficient method for the differentiation of human iPSC-derived endoderm toward enterocytes and hepatocytes. Cells. 2021;10(4):812. [Google Scholar] [PubMed]
36. Gao X, Li R, Cahan P, Zhao Y, Yourick JJ, Sprando RL. Hepatocyte-like cells derived from human induced pluripotent stem cells using small molecules: implications of a transcriptomic study. Stem Cell Res Ther. 2020;11(1):393. [Google Scholar] [PubMed]
37. Vallverdu J, Martinez Garcia de la Torre RA, Mannaerts I, Verhulst S, Smout A, Coll M, et al. Directed differentiation of human induced pluripotent stem cells to hepatic stellate cells. Nat Protoc. 2021;16(5):2542–63. [Google Scholar] [PubMed]
38. Olgasi C, Cucci A, Follenzi A. iPSC-derived liver organoids: a journey from drug screening, to disease modeling, arriving to regenerative medicine. Int J Mol Sci. 2020;21(17). [Google Scholar]
39. Liu X, Li W, Fu X, Xu Y. The immunogenicity and immune tolerance of pluripotent stem cell derivatives. Front Immunol. 2017;8:645. [Google Scholar] [PubMed]
40. Wang L, Cao J, Wang Y, Lan T, Liu L, Wang W, et al. Immunogenicity and functional evaluation of iPSC-derived organs for transplantation. Cell Discov. 2015;1:15015. [Google Scholar] [PubMed]
41. Bria A, Marda J, Zhou J, Sun X, Cao Q, Petersen BE, et al. Hepatic progenitor cell activation in liver repair. Liver Res. 2017;1(2):81–7. doi:10.1016/j.livres.2017.08.002. [Google Scholar] [PubMed] [CrossRef]
42. Pantelidou P, Sinakos E, Germanidis G, Pagkalidou E, Haidich AB, Akriviadis E, et al. Assessment of histologic risk factors for hepatocellular carcinoma in patients with chronic hepatitis B of advanced stage. Pathol Res Pract. 2023;249:154741. doi:10.1016/j.prp.2023.154741. [Google Scholar] [PubMed] [CrossRef]
43. Holczbauer A, Wangensteen KJ, Shin S. Cellular origins of regenerating liver and hepatocellular carcinoma. JHEP Rep. 2022;4(4):100416. doi:10.1016/j.jhepr.2021.100416. [Google Scholar] [PubMed] [CrossRef]
44. Huang P, Zhang L, Gao Y, He Z, Yao D, Wu Z, et al. Direct reprogramming of human fibroblasts to functional and expandable hepatocytes. Cell Stem Cell. 2014;14(3):370–84. doi:10.1016/j.stem.2014.01.003. [Google Scholar] [PubMed] [CrossRef]
45. Wang Y, Zheng Q, Sun Z, Wang C, Cen J, Zhang X, et al. Reversal of liver failure using a bioartificial liver device implanted with clinical-grade human-induced hepatocytes. Cell Stem Cell. 2023;30(5):617–31. doi:10.1016/j.stem.2023.03.013. [Google Scholar] [PubMed] [CrossRef]
46. Tsuchida T, Friedman SL. Mechanisms of hepatic stellate cell activation. Nat Rev Gastroenterol Hepatol. 2017;14(7):397–411. [Google Scholar] [PubMed]
47. Krenkel O, Tacke F. Liver macrophages in tissue homeostasis and disease. Nat Rev Immunol. 2017;17(5):306–21. [Google Scholar] [PubMed]
48. Elchaninov A, Vishnyakova P, Menyailo E, Sukhikh G, Fatkhudinov T. An eye on kupffer cells: development, phenotype and the macrophage niche. Int J Mol Sci. 2022;23(17):9868. [Google Scholar] [PubMed]
49. Hsia GSP, Esposito J, da Rocha LA, Ramos SLG, Okamoto OK. Clinical application of human induced pluripotent stem cell-derived organoids as an alternative to organ transplantation. Stem Cells Int. 2021;2021:6632160. [Google Scholar] [PubMed]
50. Liang J, Zhang H, Zhao C, Wang D, Ma X, Zhao S, et al. Effects of allogeneic mesenchymal stem cell transplantation in the treatment of liver cirrhosis caused by autoimmune diseases. Int J Rheum Dis. 2017;20(9):1219–26. [Google Scholar] [PubMed]
51. He JL, You YX, Pei X, Jiang W, Zeng QM, Chen B, et al. Tracking of stem cells in chronic liver diseases: current trends and developments. Stem Cell Rev Rep. 2023;20:447–54. [Google Scholar] [PubMed]
52. Tsuchida T, Murata S, Hasegawa S, Mikami S, Enosawa S, Hsu HC, et al. Investigation of clinical safety of human iPS cell-derived liver organoid transplantation to infantile patients in porcine model. Cell Transplant. 2020;29:963689720964384. [Google Scholar] [PubMed]
53. Duval K, Grover H, Han LH, Mou Y, Pegoraro AF, Fredberg J, et al. Modeling physiological events in 2D vs. 3D cell culture. Physiol. 2017;32(4):266–77. [Google Scholar]
54. Chou XY, Cheng KY, Yin WR, Cheng TJ, Chen RLC, Hsu SH, et al. Self-healing hydrogel containing decellularized liver matrix and endothelial cell-covered hepatocyte spheroids for rescue of injured hepatocytes. Macromol Biosci. 2024:e2300411. [Google Scholar]
55. Griffin KH, Fok SW, Kent Leach J. Strategies to capitalize on cell spheroid therapeutic potential for tissue repair and disease modeling. NPJ Regen Med. 2022;7(1):70. [Google Scholar] [PubMed]
56. Kim IG, Cho H, Shin J, Cho JH, Cho SW, Chung EJ. Regeneration of irradiation-damaged esophagus by local delivery of mesenchymal stem-cell spheroids encapsulated in a hyaluronic-acid-based hydrogel. Biomater Sci. 2021;9(6):2197–208. doi:10.1039/D0BM01655A. [Google Scholar] [PubMed] [CrossRef]
57. Leach JK, Whitehead J. Materials-directed differentiation of mesenchymal stem cells for tissue engineering and regeneration. ACS Biomater Sci Eng. 2018;4(4):1115–27. doi:10.1021/acsbiomaterials.6b00741. [Google Scholar] [PubMed] [CrossRef]
58. Sun Y, Wang Y, Zhou L, Zou Y, Huang G, Gao G, et al. Spheroid-cultured human umbilical cord-derived mesenchymal stem cells attenuate hepatic ischemia-reperfusion injury in rats. Sci Rep. 2018;8(1):2518. doi:10.1038/s41598-018-20975-0. [Google Scholar] [PubMed] [CrossRef]
59. Bierwolf J, Volz T, Lutgehetmann M, Allweiss L, Riecken K, Warlich M, et al. Primary human hepatocytes repopulate livers of mice after in vitro culturing and lentiviral-mediated gene transfer. Tissue Eng Part A. 2016;22(9–10):742–53. doi:10.1089/ten.tea.2015.0427. [Google Scholar] [PubMed] [CrossRef]
60. Wu YC, Wu GX, Chen KW, Shiu LY, Kumar S, Liu GS, et al. Transplantation of 3D adipose-derived stem cell/hepatocyte spheroids alleviates chronic hepatic damage in a rat model of thioacetamide-induced liver cirrhosis. Sci Rep. 2022;12(1):1227. doi:10.1038/s41598-022-05174-2. [Google Scholar] [PubMed] [CrossRef]
61. Takahashi Y, Yuniartha R, Yamaza T, Sonoda S, Yamaza H, Kirino K, et al. Therapeutic potential of spheroids of stem cells from human exfoliated deciduous teeth for chronic liver fibrosis and hemophilia A. Pediatr Surg Int. 2019;35(12):1379–88. doi:10.1007/s00383-019-04564-4. [Google Scholar] [PubMed] [CrossRef]
62. Kizawa H, Nagao E, Shimamura M, Zhang G, Torii H. Scaffold-free 3D bio-printed human liver tissue stably maintains metabolic functions useful for drug discovery. Biochem Biophys Rep. 2017;10:186–91. doi:10.1016/j.bbrep.2017.04.004. [Google Scholar] [PubMed] [CrossRef]
63. Yanagi Y, Nakayama K, Taguchi T, Enosawa S, Tamura T, Yoshimaru K, et al. In vivo and ex vivo methods of growing a liver bud through tissue connection. Sci Rep. 2017;7(1):14085. doi:10.1038/s41598-017-14542-2. [Google Scholar] [PubMed] [CrossRef]
64. Zhang P, Li X, Chen JY, Abate AR. Controlled fabrication of functional liver spheroids with microfluidic flow cytometric printing. Biofabrication. 2022;14(4):45011. doi:10.1088/1758-5090/ac8622. [Google Scholar] [PubMed] [CrossRef]
65. Sugawara T, Sasaki K, Akutsu H. Organoids recapitulate organs? Stem Cell Investig. 2018;5:3. [Google Scholar]
66. Wang S, Wang X, Tan Z, Su Y, Liu J, Chang M, et al. Human ESC-derived expandable hepatic organoids enable therapeutic liver repopulation and pathophysiological modeling of alcoholic liver injury. Cell Res. 2019;29(12):1009–26. doi:10.1038/s41422-019-0242-8. [Google Scholar] [PubMed] [CrossRef]
67. Messina A, Luce E, Benzoubir N, Pasqua M, Pereira U, Humbert L, et al. Evidence of adult features and functions of hepatocytes differentiated from human induced pluripotent stem cells and self-organized as organoids. Cells. 2022;11(3):537. doi:10.3390/cells11030537. [Google Scholar] [PubMed] [CrossRef]
68. Gamboa CM, Wang Y, Xu H, Kalemba K, Wondisford FE, Sabaawy HE. Optimized 3D culture of hepatic cells for liver organoid metabolic assays. Cells. 2021;10(12):3280. doi:10.3390/cells10123280. [Google Scholar] [PubMed] [CrossRef]
69. Kozlowski MT, Crook CJ, Ku HT. Towards organoid culture without Matrigel. Commun Biol. 2021;4(1):1387. doi:10.1038/s42003-021-02910-8. [Google Scholar] [PubMed] [CrossRef]
70. Brassard JA, Lutolf MP. Engineering stem cell self-organization to build better organoids. Cell Stem Cell. 2019;24(6):860–76. doi:10.1016/j.stem.2019.05.005. [Google Scholar] [PubMed] [CrossRef]
71. Takebe T, Sekine K, Enomura M, Koike H, Kimura M, Ogaeri T, et al. Vascularized and functional human liver from an iPSC-derived organ bud transplant. Nat. 2013;499(7459):481–4. doi:10.1038/nature12271. [Google Scholar] [PubMed] [CrossRef]
72. Brovold M, Keller D, Devarasetty M, Dominijanni A, Shirwaiker R, Soker S. Biofabricated 3D in vitro model of fibrosis-induced abnormal hepatoblast/biliary progenitors’ expansion of the developing liver. Bioeng Transl Med. 2021;6(3):e10207. doi:10.1002/btm2.10207. [Google Scholar] [PubMed] [CrossRef]
73. Pettinato G, Lehoux S, Ramanathan R, Salem MM, He LX, Muse O, et al. Generation of fully functional hepatocyte-like organoids from human induced pluripotent stem cells mixed with Endothelial cells. Sci Rep. 2019;9(1):8920. doi:10.1038/s41598-019-45514-3. [Google Scholar] [PubMed] [CrossRef]
74. Tanimizu N, Ichinohe N, Sasaki Y, Itoh T, Sudo R, Yamaguchi T, et al. Generation of functional liver organoids on combining hepatocytes and cholangiocytes with hepatobiliary connections ex vivo. Nat Commun. 2021;12(1):3390. doi:10.1038/s41467-021-23575-1. [Google Scholar] [PubMed] [CrossRef]
75. McCracken KW, Cata EM, Crawford CM, Sinagoga KL, Schumacher M, Rockich BE, et al. Modelling human development and disease in pluripotent stem-cell-derived gastric organoids. Nat. 2014;516(7531):400–4. doi:10.1038/nature13863. [Google Scholar] [PubMed] [CrossRef]
76. Schuster B, Junkin M, Kashaf SS, Romero-Calvo I, Kirby K, Matthews J, et al. Automated microfluidic platform for dynamic and combinatorial drug screening of tumor organoids. Nat Commun. 2020;11(1):5271. doi:10.1038/s41467-020-19058-4. [Google Scholar] [PubMed] [CrossRef]
77. Jalan-Sakrikar N, Brevini T, Huebert RC, Sampaziotis F. Organoids and regenerative hepatology. Hepatol. 2023;77(1):305–22. doi:10.1002/hep.32583. [Google Scholar] [PubMed] [CrossRef]
78. Huch M, Gehart H, Van Boxtel R, Hamer K, Blokzijl F, Verstegen MM, et al. Long-term culture of genome-stable bipotent stem cells from adult human liver. Cell. 2015;160(1–2):299–312. doi:10.1016/j.cell.2014.11.050. [Google Scholar] [PubMed] [CrossRef]
79. Sampaziotis F, Muraro D, Tysoe OC, Sawiak S, Beach TE, Godfrey EM, et al. Cholangiocyte organoids can repair bile ducts after transplantation in the human liver. Sci. 2021;371(6531):839–46. doi:10.1126/science.aaz6964. [Google Scholar] [PubMed] [CrossRef]
80. Hendriks D, Brouwers JF, Hamer K, Geurts MH, Luciana L, Massalini S, et al. Engineered human hepatocyte organoids enable CRISPR-based target discovery and drug screening for steatosis. Nat Biotechnol. 2023;41(11):1567–81. [Google Scholar] [PubMed]
81. Deng WL, Gao ML, Lei XL, Lv JN, Zhao H, He KW, et al. Gene correction reverses ciliopathy and photoreceptor loss in iPSC-derived retinal organoids from retinitis pigmentosa patients. Stem Cell Rep. 2018;10(6):2005. doi:10.1016/j.stemcr.2018.05.012. [Google Scholar] [PubMed] [CrossRef]
82. Ogawa M, Ogawa S, Bear CE, Ahmadi S, Chin S, Li B, et al. Directed differentiation of cholangiocytes from human pluripotent stem cells. Nat Biotechnol. 2015;33(8):853–61. doi:10.1038/nbt.3294. [Google Scholar] [PubMed] [CrossRef]
83. Song W, Lu YC, Frankel AS, An D, Schwartz RE, Ma M. Engraftment of human induced pluripotent stem cell-derived hepatocytes in immunocompetent mice via 3D co-aggregation and encapsulation. Sci Rep. 2015;5:16884. doi:10.1038/srep16884. [Google Scholar] [PubMed] [CrossRef]
84. Takebe T, Sekine K, Kimura M, Yoshizawa E, Ayano S, Koido M, et al. Massive and reproducible production of liver buds entirely from human pluripotent stem cells. Cell Rep. 2017;21(10):2661–70. doi:10.1016/j.celrep.2017.11.005. [Google Scholar] [PubMed] [CrossRef]
85. Stevens KR, Scull MA, Ramanan V, Fortin CL, Chaturvedi RR, Knouse KA, et al. In situ expansion of engineered human liver tissue in a mouse model of chronic liver disease. Sci Transl Med. 2017;9(399):115. doi:10.1126/scitranslmed.aah5505. [Google Scholar] [PubMed] [CrossRef]
86. Nie YZ, Zheng YW, Ogawa M, Miyagi E, Taniguchi H. Human liver organoids generated with single donor-derived multiple cells rescue mice from acute liver failure. Stem Cell Res Ther. 2018;9(1):5. doi:10.1186/s13287-017-0749-1. [Google Scholar] [PubMed] [CrossRef]
87. Hu H, Gehart H, Artegiani B, LÖpez-Iglesias C, Dekkers F, Basak O, et al. Long-term expansion of functional mouse and human hepatocytes as 3D organoids. Cell. 2018;175(6):1591–606.e19. doi:10.1016/j.cell.2018.11.013. [Google Scholar] [PubMed] [CrossRef]
88. Akbari S, Sevinc GG, Ersoy N, Basak O, Kaplan K, Sevinc K, et al. Robust, long-term culture of endoderm-derived hepatic organoids for disease modeling. Stem Cell Rep. 2019;13(4):627–41. doi:10.1016/j.stemcr.2019.08.007. [Google Scholar] [PubMed] [CrossRef]
89. Wu F, Wu D, Ren Y, Huang Y, Feng B, Zhao N, et al. Generation of hepatobiliary organoids from human induced pluripotent stem cells. J Hepatol. 2019;70(6):1145–58. doi:10.1016/j.jhep.2018.12.028. [Google Scholar] [PubMed] [CrossRef]
90. Tsuchida T, Murata S, Matsuki K, Mori A, Matsuo M, Mikami S, et al. The regenerative effect of portal vein injection of liver organoids by retrorsine/partial hepatectomy in rats. Int J Mol Sci. 2019;21(1):178. doi:10.3390/ijms21010178. [Google Scholar] [PubMed] [CrossRef]
91. Sampaziotis F, Justin AW, Tysoe OC, Sawiak S, Godfrey EM, Upponi SS, et al. Reconstruction of the mouse extrahepatic biliary tree using primary human extrahepatic cholangiocyte organoids. Nat Med. 2017;23(8):954–63. doi:10.1038/nm.4360. [Google Scholar] [PubMed] [CrossRef]
92. Ogawa M, Jiang JX, Xia S, Yang D, Ding A, Laselva O, et al. Generation of functional ciliated cholangiocytes from human pluripotent stem cells. Nat Commun. 2021;12(1):6504. doi:10.1038/s41467-021-26764-0. [Google Scholar] [PubMed] [CrossRef]
93. Zhang W, Lanzoni G, Hani H, Overi D, Cardinale V, Simpson S, et al. Patch grafting, strategies for transplantation of organoids into solid organs such as liver. Biomat. 2021;277:121067. doi:10.1016/j.biomaterials.2021.121067. [Google Scholar] [PubMed] [CrossRef]
94. Zhang W, Wauthier E, Lanzoni G, Hani H, Yi X, Overi D, et al. Patch grafting of organoids of stem/progenitors into solid organs can correct genetic-based disease states. Biomat. 2022;288:121647. doi:10.1016/j.biomaterials.2022.121647. [Google Scholar] [PubMed] [CrossRef]
95. Nam D, Park MR, Lee H, Bae SC, Gerovska D, Arauzo-Bravo MJ, et al. Induced endothelial cell-integrated liver assembloids promote hepatic maturation and therapeutic effect on cholestatic liver fibrosis. Cells. 2022;11(14):2242. doi:10.3390/cells11142242. [Google Scholar] [PubMed] [CrossRef]
96. Son JS, Park CY, Lee G, Park JY, Kim HJ, Kim G, et al. Therapeutic correction of hemophilia A using 2D endothelial cells and multicellular 3D organoids derived from CRISPR/Cas9-engineered patient iPSCs. Biomaterials. 2022;283:121429. doi:10.1016/j.biomaterials.2022.121429. [Google Scholar] [PubMed] [CrossRef]
97. Wang C, Zhang L, Sun Z, Yuan X, Wu B, Cen J, et al. Dedifferentiation-associated inflammatory factors of long-term expanded human hepatocytes exacerbate their elimination by macrophages during liver engraftment. Hepatology. 2022;76(6):1690–705. [Google Scholar] [PubMed]
98. Harrison SP, Siller R, Tanaka Y, Chollet ME, de la Morena-Barrio ME, Xiang Y, et al. Scalable production of tissue-like vascularized liver organoids from human PSCs. Exp Mol Med. 2023;55(9):2005–24. [Google Scholar] [PubMed]
99. Reddy MSB, Ponnamma D, Choudhary R, Sadasivuni KK. A comparative review of natural and synthetic biopolymer composite scaffolds. Polymers. 2021;13(7):1105. [Google Scholar] [PubMed]
100. Zhou Z, Song P, Wu Y, Wang M, Shen C, Ma Z, et al. Dual-network DNA-silk fibroin hydrogels with controllable surface rigidity for regulating chondrogenic differentiation. Mater Horiz. 2024;11:1465–83. [Google Scholar]
101. Mi CH, Qi XY, Ding YW, Zhou J, Dao JW, Wei DX. Recent advances of medical polyhydroxyalkanoates in musculoskeletal system. Biomater Transl. 2023;4(4):234–47. [Google Scholar] [PubMed]
102. Hussein M, Pasqua M, Pereira U, Benzoubir N, Duclos-Vallee JC, Dubart-Kupperschmitt A, et al. Microencapsulated hepatocytes differentiated from human induced pluripotent stem cells: optimizing 3D culture for tissue engineering applications. Cells. 2023;12(6):865. [Google Scholar] [PubMed]
103. Porzionato A, Stocco E, Barbon S, Grandi F, Macchi V, de Caro R. Tissue-engineered grafts from human decellularized extracellular matrices: a systematic review and future perspectives. Int J Mol Sci. 2018;19(12):4117. [Google Scholar] [PubMed]
104. Dai Q, Jiang W, Huang F, Song F, Zhang J, Zhao H. Recent advances in liver engineering with decellularized scaffold. Front Bioeng Biotechnol. 2022;10:831477. [Google Scholar] [PubMed]
105. Parmaksiz M, Dogan A, Odabas S, Elcin AE, Elcin YM. Clinical applications of decellularized extracellular matrices for tissue engineering and regenerative medicine. Biomed Mater. 2016;11(2):022003. [Google Scholar] [PubMed]
106. Jiang WC, Cheng YH, Yen MH, Chang Y, Yang VW, Lee OK. Cryo-chemical decellularization of the whole liver for mesenchymal stem cells-based functional hepatic tissue engineering. Biomaterials. 2014;35(11):3607–17. [Google Scholar] [PubMed]
107. Nobakht Lahrood F, Saheli M, Farzaneh Z, Taheri P, Dorraj M, Baharvand H, et al. Generation of transplantable three-dimensional hepatic-patch to improve the functionality of hepatic cells in vitro and in vivo. Stem Cells Dev. 2020;29(5):301–13. [Google Scholar] [PubMed]
108. Bruinsma BG, Kim Y, Berendsen TA, Ozer S, Yarmush ML, Uygun BE. Layer-by-layer heparinization of decellularized liver matrices to reduce thrombogenicity of tissue engineered grafts. J Clin Transl Res. 2015;1(1):48–56. [Google Scholar] [PubMed]
109. Takeishi K, Collin de l’Hortet A, Wang Y, Handa K, Guzman-Lepe J, Matsubara K, et al. Assembly and function of a bioengineered human liver for transplantation generated solely from induced pluripotent stem cells. Cell Rep. 2020;31(9):107711. doi:10.1016/j.celrep.2020.107711. [Google Scholar] [PubMed] [CrossRef]
110. Li Y, Wu Q, Yang Z, He Y, Weng C, Gao M, et al. Heterotopic vascularization and functionalization of implantable bio engineered hepatic tissue alleviates liver injury in rats. Liver Int. 2020;40(3):712–26. doi:10.1111/liv.14267. [Google Scholar] [PubMed] [CrossRef]
111. Guo B, Zhou Q, Chen J, Xin J, Jiang L, Yang H, et al. Orthotopic transplantation of functional bioengineered livers in rats. ACS Biomater Sci Eng. 2023;9(4):1940–51. doi:10.1021/acsbiomaterials.2c01213. [Google Scholar] [PubMed] [CrossRef]
112. Ko IK, Peng L, Peloso A, Smith CJ, Dhal A, Deegan DB, et al. Bioengineered transplantable porcine livers with re-endothelialized vasculature. Biomaterials. 2015;40:72–9. doi:10.1016/j.biomaterials.2014.11.027. [Google Scholar] [PubMed] [CrossRef]
113. Ni R, Muenster U, Zhao J, Zhang L, Becker-Pelster EM, Rosenbruch M, et al. Exploring polyvinylpyrrolidone in the engineering of large porous PLGA microparticles via single emulsion method with tunable sustained release in the lung: in vitro and in vivo characterization. J Control Release. 2017;249:11–22. [Google Scholar] [PubMed]
114. Shaheen MF, Joo DJ, Ross JJ, Anderson BD, Chen HS, Huebert RC, et al. Sustained perfusion of revascularized bioengineered livers heterotopically transplanted into immunosuppressed pigs. Nat Biomed Eng. 2020;4(4):437–45. [Google Scholar] [PubMed]
115. Anderson BD, Nelson ED, Joo D, Amiot BP, Katane AA, Mendenhall A, et al. Functional characterization of a bioengineered liver after heterotopic implantation in pigs. Commun Biol. 2021;4(1):1157. [Google Scholar] [PubMed]
116. Ming Z, Tang X, Liu J, Ruan B. Advancements in research on constructing physiological and pathological liver models and their applications utilizing bioprinting technology. Mol. 2023;28(9):3683. [Google Scholar]
117. Kang D, Hong G, An S, Jang I, Yun WS, Shim JH, et al. Bioprinting of multiscaled hepatic lobules within a highly vascularized construct. Small. 2020;16(13):e1905505. [Google Scholar] [PubMed]
118. Yang H, Sun L, Pang Y, Hu D, Xu H, Mao S, et al. Three-dimensional bioprinted hepatorganoids prolong survival of mice with liver failure. Gut. 2021;70(3):567–74. doi:10.1136/gutjnl-2019-319960. [Google Scholar] [PubMed] [CrossRef]
119. Wang J, Ren H, Liu Y, Sun L, Zhang Z, Zhao Y, et al. Bioinspired artificial liver system with hiPSC-derived hepatocytes for acute liver failure treatment. Adv Healthc Mater. 2021;10(23):e2101580. doi:10.1002/adhm.202101580. [Google Scholar] [PubMed] [CrossRef]
120. Carter K, Lee HJ, Na KS, Fernandes-Cunha GM, Blanco IJ, Djalilian A, et al. Characterizing the impact of 2D and 3D culture conditions on the therapeutic effects of human mesenchymal stem cell secretome on corneal wound healing in vitro and ex vivo. Acta Biomater. 2019;99:247–57. doi:10.1016/j.actbio.2019.09.022. [Google Scholar] [PubMed] [CrossRef]
121. Zeshan M, Amjed N, Ashraf H, Farooq A, Akram N, Zia KM. A review on the application of chitosan-based polymers in liver tissue engineering. Int J Biol Macromol. 2024;262:129350. doi:10.1016/j.ijbiomac.2024.129350. [Google Scholar] [PubMed] [CrossRef]
122. Li C, Zhao H, Cheng L, Wang B. Allogeneic vs. autologous mesenchymal stem/stromal cells in their medication practice. Cell Biosci. 2021;11(1):187. doi:10.1186/s13578-021-00698-y. [Google Scholar] [PubMed] [CrossRef]
123. Bashor CJ, Hilton IB, Bandukwala H, Smith DM, Veiseh O. Engineering the next generation of cell-based therapeutics. Nat Rev Drug Discov. 2022;21(9):655–75. [Google Scholar] [PubMed]
124. Nam SA, Seo E, Kim JW, Kim HW, Kim HL, Kim K, et al. Graft immaturity and safety concerns in transplanted human kidney organoids. Exp Mol Med. 2019;51(11):1–13. [Google Scholar]
125. Arjmand B, Rabbani Z, Soveyzi F, Tayanloo-Beik A, Rezaei-Tavirani M, Biglar M, et al. Advancement of organoid technology in regenerative medicine. Regen Eng Transl Med. 2023;9(1):83–96. [Google Scholar] [PubMed]
126. de Jongh D, Massey EK, Cronin AJ, Schermer MHN, Bunnik EM, Consortium V. Early-phase clinical trials of bio-artificial organ technology: a systematic review of ethical issues. Transpl Int. 2022;35:10751. [Google Scholar] [PubMed]
Cite This Article
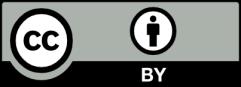
This work is licensed under a Creative Commons Attribution 4.0 International License , which permits unrestricted use, distribution, and reproduction in any medium, provided the original work is properly cited.