Open Access
ARTICLE
Mesenchymal stromal cells modulate unfolded protein response and preserve β-cell mass in type 1 diabetes
Department of Pediatrics, The Sixth Medical Center, Chinese PLA General Hospital, Beijing, 100037, China
* Corresponding Author: ZUO LUAN. Email:
BIOCELL 2024, 48(7), 1115-1126. https://doi.org/10.32604/biocell.2024.050493
Received 08 February 2024; Accepted 19 April 2024; Issue published 03 July 2024
Abstract
Introduction: Transplantation of mesenchymal stromal cells (MSCs) is a promising therapy for type 1 diabetes (T1D). However, whether the infused MSCs affect the endoplasmic reticulum stress or subsequent unfolded protein response in β cells remains unclear. Methods: To investigate this, we induced early-onset T1D in non-obese diabetic mice using streptozotocin. Subsequently, T1D mice were randomly assigned to receive either MSCs or phosphate-buffered saline. We observed the in vivo homing of MSCs and assessed their effectiveness by analyzing blood glucose levels, body weight, histopathology, pancreatic protein expression, and serum levels of cytokines, proinsulin, and C-peptide. Results: Infused MSCs were found in the lungs, liver, spleen, and pancreas of T1D mice. They exhibited various effects, including reducing blood glucose levels, regulating immunity, inhibiting inflammation, increasing β-cell areas, and reducing the expression of key proteins in the unfolded protein response pathway. Fasting serum proinsulin and C-peptide levels were significantly higher in the MSCs treatment group than in the T1D model group. However, there was no significant difference in the biomarker of β-cell endoplasmic reticulum stress, the ratio of fasting serum proinsulin to C-peptide, between the two groups. Conclusion: Our findings reveal that MSCs infusion does not alleviate endoplasmic reticulum stress in β cells directly but modulates the unfolded protein response pathway to preserve β-cell mass and function in T1D mice.Graphic Abstract
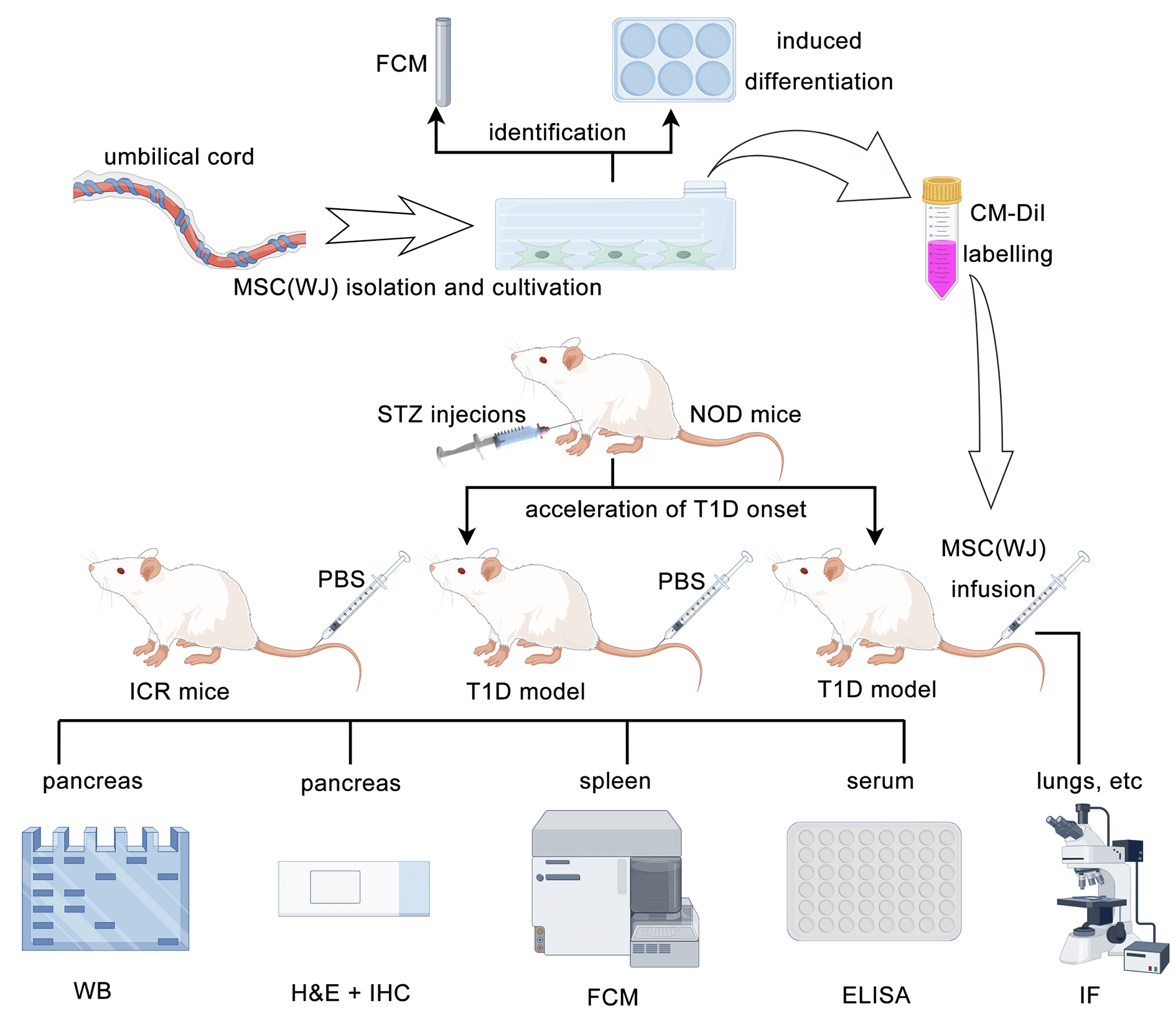
Keywords
Abbreviations
ATF-6 | Activating transcription factor-6 |
BiP | Immunoglobulin heavy-chain binding protein |
CHOP | C/EBP-homologous protein |
ER | Endoplasmic reticulum |
IPGTT | Intraperitoneal glucose tolerance tests |
MSC(WJ) | Mesenchymal stromal cells derived from Wharton’s jelly of the umbilical cord |
NBG | Non-fasting blood glucose |
NOD | Non-obese diabetic |
PI | Proinsulin |
STZ | Streptozotocin |
UPR | Unfolded protein responsess |
Type 1 diabetes (T1D) is the primary form of diabetes affecting children [1]. Over the past three decades, T1D incidence has increased at an average annual rate of 3%–4% worldwide [2]. A cohort study conducted in Sweden based on national registers revealed that children and adolescents with T1D have a reduced average life expectancy of 10–16 years [3]. The necessity for lifelong exogenous insulin administration and the high risk of serious complications highlight the urgent need for innovative therapies to address this condition.
Contrary to the long-held belief that over 90% of the β cells are lost at T1D onset, recent studies have shown that a notable proportion of β cells, ranging from 14% to 60%, can be found in patients after diagnosis, though their function is diminished [4,5]. When hyperglycemia is alleviated through insulin administration, the remaining β cells can recover their function, leading to a so-called honeymoon period with low or no need for exogenous insulin. However, most cells are destroyed within a few months of T1D progression. These findings underline the importance of preserving the residual β cells as a key strategy for preventing or reversing T1D [5].
Owing to their high secretory activity, pancreatic β cells are susceptible to an increased demand for insulin or inflammation [6], resulting in the production of excessive proinsulin (PI) that exceeds the processing capacity of the endoplasmic reticulum (ER). This leads to an accumulation of the unfolded and misfolded proteins in the ER lumen, known as ER stress [7]. In response to physiological ER stress, the unfolded protein response (UPR) is activated to restore ER homeostasis. This is achieved by reducing the synthesis of new proteins, increasing the folding ability of the ER, and degrading the misfolded proteins [8]. However, if ER stress persists, it over-activates the UPR pathway, ultimately leading to β-cell death [9,10]. Therefore, a combined therapy that modulates immunity and alleviates ER stress or UPR in β cells may be preferable to reverse T1D [11,12].
Mesenchymal stromal cells (MSCs) transplantation is a promising therapy for T1D because of its ability to modulate immunity and repair injured tissues [13,14]. Many experimental and clinical studies have shown that cultured MSCs are safe and effective in the treatment of T1D [15–18]. However, the mechanism of action remains incompletely understood. A recent study indicated that MSCs could enhance β-cell function by down-regulating the mRNA expression of key pancreatic UPR pathway proteins, such as immunoglobulin heavy-chain binding protein (BiP), activating transcription factor-6 (ATF-6), and C/EBP-homologous protein (CHOP), in T1D rats [19]. However, it did not analyze the expression level of these proteins or investigate β-cell ER stress.
Therefore, we aimed to explore this aspect by assessing a novel indicator of ER stress in β cells, the ratio of fasting serum PI to C-peptide [20], and the protein level of BiP, ATF-6, and CHOP in the pancreas. After inducing early-onset T1D in non-obese diabetic (NOD) mice, we administered a single dose of MSCs derived from Wharton’s jelly of the umbilical cord (MSC(WJ)) [21] immediately following diagnosis. Our findings suggest that MSC(WJ) infusion has the potential to modulate the UPR pathway and preserve β-cell mass and function.
Isolation, culture, and characterization of MSC(WJ)
A segment of the umbilical cord was obtained from a healthy woman who gave birth at the Chinese PLA General Hospital in February 2023. Written informed consent was obtained from the donor before delivery. The protocol was approved by the Ethics Committee of the Chinese PLA General Hospital (No. S2022-107-19; Date: June 15th, 2022). MSC(WJ) was harvested from Wharton’s jelly of the umbilical cord, as previously described [22], and characterized by phenotype analysis and induced differentiation in vitro [23]. The identification process is as described previously [14]. Briefly, the MSC(WJ) phenotype was determined by labeling with antibodies against CD14 (Biolegend, 325603, San Diego, USA), CD19 (Biolegend, 302205, San Diego, USA), CD34 (Biolegend, 343503, San Diego, USA), CD45 (Biolegend, 384405, San Diego, USA), CD73 (Biolegend, 344015, San Diego, USA), CD90 (Biolegend, 328107, San Diego, USA), CD105 (Biolegend, 323203, San Diego, USA), and HLA-DR (Biolegend, 327005, San Diego, USA), then detected using full-spectrum flow cytometry (Cytek, NL-CLC, San Diego, USA). MSC(WJ) cultures were induced to differentiate into adipocytes, osteocytes, and chondrocytes using an adipogenesis differentiation kit (Applied Cell, AC-1001029, Shanghai, China), osteogenesis differentiation kit (Applied Cell, AC-1001027, Shanghai, China), and chondrogenesis differentiation kit (Applied Cell, AC-1001028, Shanghai, China), respectively. After approximately 3 weeks, adipocytes were identified using Oil Red O (Solarbio, G1262, Beijing, China) staining, osteocytes with Alizarin Red S (Solarbio, G1450, Beijing, China) staining, and chondrocytes with Alcian Blue (Solarbio, G1565, Beijing, China) staining. Primary cells were expanded for 1–2 passages and cryopreserved following the Instructions of Serum-Free Medium for MSCs (version 2019). In this experiment, MSC(WJ) was cultured for approximately 72 h after thawing and used at the third passage. To demonstrate the homing of MSC(WJ) in vivo, the cells were labeled with CellTracker CM-DiI (Invitrogen, C7000, Carlsbad, USA) 2 h before transplantation, according to the manufacturer’s protocol.
All procedures involving animals were performed under the Guide for the Care and Use of Laboratory Animals (8th Edition, USA) and were approved by the Ethics Review Committee for Animal Experiments of the Chinese PLA General Hospital (No. 2022-X18-77; Date: July 20th, 2022). Female NOD and Institute of Cancer Research (ICR) mice, aged 3–4 weeks, were purchased from HFK Biotechnology Co. Ltd. (Beijing, China) and housed in a specific pathogen-free facility at the Medical Laboratory Animal Center of the Chinese PLA General Hospital. Before sampling, the mice were anesthetized with 1% pentobarbital sodium (JHBio Co., Ltd., 57330, Beijing, China) to minimize their suffering.
After a 1-week acclimation period, 4-week-old NOD mice were intraperitoneally injected with streptozotocin (STZ, Sigma-Aldrich, S0130, St. Louis, USA) for 4 consecutive days (45 mg/kg/day) to accelerate T1D onset, which may be similar to childhood-onset T1D. ICR mice served as the control group (n = 6) and were administered the diluent. Non-fasting blood glucose (NBG) levels were monitored every 5 days via the tail vein using a glucometer (Johnson & Johnson, 06940202A, New Brunswick, USA). NOD mice with blood glucose levels >13.9 mmol/L for 2 consecutive days were diagnosed as overt T1D (n = 24) [24,25]. The mice were then randomly assigned to either the MSC(WJ) treatment group (n = 18) or the T1D model group (n = 6). Mice in the MSC(WJ) treatment group received an infusion of 1 × 106 MSC(WJ) suspended in 0.1 mL of phosphate-buffered saline (PBS, Gibco, 20012027, Carlsbad, USA) via tail vein, with a viability of 87.4%, on the same day they were diagnosed with T1D. The T1D model and control groups received 0.1 mL of PBS only. The cell suspension and PBS were infused slowly for more than 10 s.
MSC(WJ) homing and pancreatic histology
To evaluate the homing of MSC(WJ), we randomly selected and sacrificed two mice from the MSC(WJ) treatment group at 12 h and 1, 2, 3, 5, and 7 days after MSC(WJ) infusion. The pancreases were collected and preserved in Tissue-Tek O.C.T. Compound (Sakura Finetek, 4583, Torrance, USA), flash-frozen in liquid nitrogen, and sliced into 5 μm sections with a 300 μm interval. Sections were stained with Hoechst 33342 (Beyotime, G1127, Shanghai, China) following an established protocol and examined using an inverted fluorescence microscope (Olympus, IX-51, Tokyo, Japan).
The remaining mice (six in each group) were sacrificed on day 16 after infusion, and three mice in each group were used for histological analysis. Paraffin-embedded pancreatic tissues were sectioned at 4 μm and stained with hematoxylin (Servicebio, G1004, Wuhan, China) and eosin (Servicebio, G1001, Wuhan, China). Three sections at a 300-μm interval were prepared for each mouse to ensure observation of different islets, with 20 islets per mouse evaluated. Insulitis was scored on a 0–4 scale. Additionally, the insulin and PI contents in the islets were detected by immunohistochemistry, as previously reported [26]. The insulin- and PI-positive areas, islet areas, and average optical density of these areas were measured using ImageJ software (NIH, Bethesda, USA). The sizes of the insulin- or PI-positive areas in each image were quantified by calculating the percentage of the islet area.
Intraperitoneal glucose tolerance tests (IPGTT) and enzyme-linked immunosorbent assay (ELISA)
Before administering STZ and after diagnosing T1D, IPGTT was performed to assess β-cell function in ICR and NOD mice. Six mice in each group were fasted for 7 h and injected intraperitoneally with glucose (1.5 g/kg). Blood glucose levels were measured in the tail vein at 0, 30, 60, 90, and 120 min post injection. The data were used to determine the area under the curve, which was visualized as a bar graph for each group.
Blood samples were obtained from the right ventricle of the mice under anesthesia, and plasma was collected after blood coagulation. The levels of PI, C-peptide, interleukin (IL)-10, and interferon (IFN)-γ were measured with ELISA kits (Mercodia, 10-1232-01, Uppsala, Sweden; ALPCO, 80-CPTMS-E01, Salem, USA; Proteintech, KE10008, Rosemont, USA; Proteintech, KE10094, Rosemont, USA), following the manufacturer’s instructions.
To obtain single-cell suspensions, the spleens were passed through 70-μm cell strainers (Corning Falcon, 352350, Corning, USA), and the remaining red blood cells were lysed with red blood cell lysis buffer (Biolegend, 420301, San Diego, USA). The resulting cells were counted using a Countess Cell Counter (Invitrogen, AMQAX2000, Carlsbad, USA) and resuspended in Roswell Park Memorial Institute 1640 medium (Gibco, 11875093, Carlsbad, USA) at a concentration of 1 × 107 cells/mL for flow cytometry analysis. The cells were then stained with a Zombie NIR Fixable Viability Kit (Biolegend, 423105, San Diego, USA) and resuspended in a cell-staining buffer (Biolegend, 420201, San Diego, USA). Subsequently, the cells were stained with monoclonal antibodies against Brilliant Violet 510-CD3 (Biolegend, 100234, San Diego, USA), PE-CD4 (Biolegend, 100407, San Diego, USA), PE/Cyanine7-CD8 (Biolegend, 100722, San Diego, USA), Brilliant Violet 421-CD25 (Biolegend, 100923, San Diego, USA), FITC-CD45 (Biolegend, 157214, San Diego, USA), and Foxp3 (Thermo Fisher Scientific, 17-5773-82, Waltham, USA). Data were acquired using full-spectrum flow cytometry (Cytek, NL-CLC, San Diego, USA) and analyzed with the FlowJo software (FlowJo, Ashland, USA).
Proteins were extracted from the pancreatic tissues of the remaining three mice in each group. The tail of the pancreas (20 mg) per mouse was added to RIPA lysis buffer (0.2 mL, Baiqiandu, Ba1004, Wuhan, China) and vibrated at 30 Hz for 5 min using TissueLyser (Qiagen, 85300, Hilden, Germany). The homogenate was then placed on ice for 30 min and centrifuged at 4°C and 13,000 × g for 5 min. The supernatant was carefully collected, and the protein content was measured with a bicinchoninic acid protein quantification kit (Baiqiandu, Ba1086, Wuhan, China). Protein samples (200 μg) from each mouse were resuspended in sodium dodecyl sulfate loading buffer (50 μL, Baiqiandu, Ba1011, Wuhan, China) and heated at 100°C for 5 min. After cooling to room temperature (20°C–24°C), the protein solutions were loaded onto five pieces of PAGE gels (Aqlabtech, AQ120-01, Beijing, China) and subjected to electrophoresis. The proteins were then transferred to polyvinylidene difluoride membranes (Millipore, IPVH00010, Burlington, USA). The membranes were blocked in 5% skim milk (Baiqiandu, Ba1033, Wuhan, China) for 1 h at room temperature and incubated overnight at 4°C with one of the following primary antibodies: BiP (Cell Signaling Technology, 3177S, Danvers, USA, 1:1,000), ATF-6 (Cell Signaling Technology, 65880S, Danvers, USA, 1:1,000), CHOP (Cell Signaling Technology, 5554S, Danvers, USA, 1:1,000), and GAPDH (Cell Signaling Technology, 5174S, Danvers, USA, 1:1,000). After three 5-min washes with tris-buffered saline containing 0.1% Tween-20 (Baiqiandu, Ba1100, Wuhan, China), the membranes were incubated with the secondary antibody, horseradish peroxidase-conjugated goat anti-rabbit IgG (1:3,000, Servicebio, GB23303, Wuhan, China), at room temperature for 1 h. After another four washes, the protein bands were visualized in an enhanced chemiluminescence system (Peiqing Technology, JS-1070P, Shanghai, China), and the optical density of the bands was measured using ImageJ software (NIH, Bethesda, USA).
The mean ± SD represents all data presented. Statistical analyses were performed with SPSS 21.0 software (IBM, Armonk, USA). Two sets of data were compared using Student’s t-test, while three sets were analyzed using one-way ANOVA followed by the post hoc Tukey test. If the assumption of homogeneity of variances was violated for three sets of data, they were then subjected to the Kruskal-Wallis test. Statistical significance was set at p < 0.05.
Identification of MSC(WJ) characteristics
To determine the properties of the cultured MSC(WJ), we examined its immunophenotypic features and multi-lineage differentiation potential. Over 98% of the MSC(WJ) showed a significant presence of CD73, CD90, and CD105, with little presence of CD14, CD19, CD34, CD45, and HLA-DR (Fig. A1(A)). Adherent MSC(WJ) exhibited shuttle-shaped and fibroblast-like morphology (Fig. A1(B)) and the capacity to differentiate into osteoblasts, adipocytes, and chondroblasts under appropriate conditions (Figs. A1(C)–A1(E)). The results demonstrate that cultured MSC(WJ) possesses characteristics of MSCs.
Schedule, IPGTT, NBG, and body weight of the mice
In our preliminary experiments, NOD mice were administered 40 mg/kg/day STZ for 4 or 5 days, as described previously [26,27]. However, the blood glucose levels of the mice were either below the diagnostic criteria or excessively high, leading to death and severe weight loss after 2–3 weeks. To address this aspect, we adjusted the dosage to 45 mg/kg/day for 4 days (Fig. 1A), resulting in moderate hyperglycemia, and the mice survived for more than 5 weeks without exogenous insulin. Using this protocol, we administered STZ to 40 NOD mice to accelerate the onset of T1D, of which 24 developed diabetes before 6 weeks of age.
Figure 1: Experimental processes and monitoring indicators. (A) Schedule of the experiment. (B and C) Results of intraperitoneal glucose tolerance tests (IPGTT) and the area under the curve of IPGTT, before and after streptozotocin (STZ) injections. (D and E) Non-fasting blood glucose (NBG) levels and body weight of the mice. MSC(WJ), Mesenchymal stromal cells derived from Wharton’s jelly of the umbilical cord; NOD, non-obese diabetic. Con(4w) and Con(5w), the control groups of 4-week-old and 5-week-old ICR mice; NOD(4w), the group of 4-week-old NOD mice before STZ injections; T1D(5w), the type 1 diabetes model group of 5-week-old NOD mice; MSC, the MSC(WJ) treatment group. Data are presented as mean ± SD; n = 6. ***p < 0.001 vs. T1D; ns, no significant differences.
To assess the success of the induced models, we conducted the IPGTT both before and after T1D diagnosis (Fig. 1B). Before STZ administration, NOD mice displayed a significantly lower area under the curve of blood glucose compared to ICR mice; however, after one week, when diagnosed with T1D, the mice in the T1D model group exhibited a markedly higher area under the curve of blood glucose than those in the control group (Fig. 1C). These results indicate that the basal blood glucose levels of NOD mice are lower than those of ICR mice, but when diagnosed with T1D, the glucose metabolism function of NOD mice is significantly impaired.
NBG levels and body weight were monitored for 25 days. The MSC(WJ) treatment group had significantly lower NBG levels than the T1D model group at 10 and 15 days post-transplantation (Fig. 1D). Although the body weight of the MSC(WJ) treatment group was slightly higher than that of the T1D model group 15 days post-transplantation, no significant disparity was found between the two groups (Fig. 1E). Hence, these findings suggest that infused MSC(WJ) can reduce blood glucose levels in newly diagnosed T1D mice; however, its effect on body weight is not remarkable in the short term.
To track MSC(WJ) in vivo, we systemically infused CM-DiI-labeled cells into T1D mice. After 12 h, a significant number of MSC(WJ) was identified in the lungs, liver, and spleen, whereas a small quantity was observed in the pancreatic lymph nodes and pancreas. These cells remained present for at least 7 days (Figs. 2 and A2). These findings demonstrate the homing of MSC(WJ) to the lungs, liver, spleen, and pancreas in T1D mice.
Figure 2: Mesenchymal stromal cells derived from Wharton’s jelly of umbilical cord (MSC(WJ)) homing in vivo. (A and B) The morphology of the third-passage MSC(WJ) cultured in vitro and labeled with CM-DiI. Scale bar = 100 μm. (C and D) Representative images of pancreatic lymph nodes of mice with type 1 diabetes at 12 h (Scale bar = 100 μm) and 7 days (Scale bar = 50 μm) after MSC(WJ) infusion. (E–H) Representative micrographs of the pancreas of mice with type 1 diabetes at different time points after MSC(WJ) infusion. The areas marked by the dotted line indicate the presence of pancreatic islets. Scale bar = 100 μm.
Immunomodulatory effects of MSC(WJ) on T1D mice
To investigate the effects of MSC(WJ) on the immune system of mice, we measured insulitis, serum IL-10 and IFN-γ levels, and spleen T-lymphocyte subsets on day 16 post-infusion. In the T1D model group, only 1.67% of the pancreatic islets showed no insulitis, whereas approximately 50.0% displayed severe insulitis (>50% infiltration) or complete monocyte infiltration. In contrast, the MSC(WJ) treatment group showed no monocyte infiltration in 20.0% of the pancreatic islets, whereas severe insulitis was observed in 23.3% of the islets (Figs. 3A, 3B). The T1D model group showed a significantly higher mean insulitis score than that of the MSC(WJ) treatment group (Fig. 3C). Moreover, the MSC(WJ) treatment group exhibited significantly higher levels of IL-10 (Fig. 3D) and lower levels of IFN-γ (Fig. 3E) in peripheral blood, compared with the T1D model group. Although the percentages of CD4+ and CD8+ T cells in the spleen were not significantly different between the two groups (Figs. 3F, 3G), the ratio of CD4+ T cells to CD8+ T cells was significantly lower in the MSC(WJ) treatment group than in the T1D model group (Fig. 3H). In contrast, the frequency of CD4+ CD25+ Foxp3+ regulatory T cells was significantly higher (Fig. 3I). These results indicate that MSC(WJ) treatment modulates the immunity of T1D mice.
Figure 3: Effects of infused Mesenchymal stromal cells derived from Wharton’s jelly of the umbilical cord (MSC(WJ)) on the immune system of mice. (A) representative micrograph of islets stained with hematoxylin and eosin (H&E) on day 16 post-transplantation. Scale bar = 50 μm. (B) Percentage of islets in each infiltration category (grade 0, no insulitis; grade 1, monocyte infiltration ≤25%; grade 2, monocyte infiltration >25%, but ≤50%; grade 3, monocyte infiltration >50%, but <100%; and grade 4, complete monocyte infiltration). Three mice in each group were analyzed, and 20 islets of each mouse were evaluated. (C) Insulitis scores of mice. n = 3. (D and E) Fasting serum IL-10 and IFN-γ levels. (F and G) Percentages of CD4+ T cells and CD8+ T cells in the CD3+ T cell population. (H) The ratio of CD4+ T cells to CD8+ T cells. (I) Percentage of regulatory T cells (Tregs) in the CD4+ T cell subset. Con, the control group; T1D, the type 1 diabetes model group; MSC, the MSC(WJ) treatment group. Data are presented as mean ± SD. n = 5. *p < 0.05, **p < 0.01, ***p < 0.001; ns, no significant differences.
MSC(WJ) preserving β cells in T1D mice
To evaluate the impact of infused MSC(WJ) on β cells in T1D mice, we analyzed the immunohistochemical staining sections of pancreatic islets and measured the levels of fasting serum PI and C-peptide. Both insulin and PI immunohistochemical staining showed that MSC(WJ) infusion preserved β-cell mass in islets on day 16 post-transplantation (Fig. 4A). The MSC(WJ) treatment group showed significantly higher percentages of insulin- and PI-positive areas than did the T1D model group (Figs. 4B, 4C), both indicating an improvement in residual β-cell areas. However, compared with the T1D model group, the average optical density of insulin-positive areas in the MSC(WJ) treatment group was slightly decreased (Fig. 4D), while the average optical density of PI-positive areas was significantly decreased (Fig. 4E), indicating that the decrease of insulin concentration in islets was not significant in the MSC(WJ) treatment group. Additionally, the MSC(WJ) treatment group exhibited significantly higher levels of fasting serum PI and C-peptide than did the T1D model group (Figs. 5A, 5B). These results suggest that infused MSC(WJ) helps to preserve the residual β-cell mass and function.
Figure 4: Effects of Mesenchymal stromal cells derived from Wharton’s jelly of umbilical cord (MSC(WJ)) infusion on β-cell mass. (A) Representative micrographs of islets stained by immunohistochemistry on day 16 post-transplantation. Scale bar = 50 μm. (B and C) Percentage of insulin- and proinsulin (PI)-positive areas in islets. (D and E) Average optical density (AOD) of insulin- and PI-positive areas in islets. The areas and AOD of these areas were measured using ImageJ software. Three mice in each group were analyzed, and 10 islets of each mouse were evaluated. Con, the control group; T1D, the type 1 diabetes model group; MSC, the MSC(WJ) treatment group. Data are presented as mean ± SD. n = 3. **p < 0.01, ***p < 0.001; ns, no significant differences.
Figure 5: Impacts of infused Mesenchymal stromal cells derived from Wharton’s jelly of the umbilical cord (MSC(WJ)) on β-cell function and pancreatic protein expression. (A and B) Fasting serum proinsulin (PI) and C-peptide levels determined by ELISA. n = 5. (C) Ratio of fasting serum PI to C-peptide. (D) Representative western blots of key proteins in the unfolded protein response pathway. n = 3. (E) Expression level of the key proteins. The optical density of the proteins was normalized against GAPDH. Con, the control group; T1D, the type 1 diabetes model group; MSC, the MSC(WJ) treatment group. Data are presented as mean ± SD. *p < 0.05, **p < 0.01, ***p < 0.001; ns, no significant differences.
Effect of MSC(WJ) on ER stress and UPR pathway in β cells
To investigate the impact of infused MSC(WJ) on ER stress and UPR in β cells, we assessed the ratio of the serum PI to C-peptide and examined the expression of key proteins in the UPR pathway. Although the ratio of fasting serum PI to C-peptide was slightly higher in MSC(WJ)-treated mice than in the T1D model mice, there was no significant difference between the two groups (Fig. 5C). However, we observed a significant decrease in the expression of UPR sensors—BiP, ATF-6, and CHOP—in the MSC(WJ) treatment group compared with those in the T1D model group (Figs. 5D, 5E). Consequently, although infused MSC(WJ) may not mitigate ER stress in β cells, it inhibited the UPR pathway.
T1D is a chronic autoimmune disease characterized by the destruction of β cells, which is partly caused by persistent ER stress and over-activated UPR. In this preclinical study, we used a robust early-onset T1D model in NOD mice and observed that MSC(WJ) infusion effectively decreased blood glucose levels, regulated immunity, reduced the expression of sensors in the UPR pathway, and increased residual β-cell mass and insulin production. However, it did not alleviate the stress status of β cells. Our study provides novel evidence for understanding the potential mechanism of MSC(WJ) in T1D treatment. These findings suggest that the UPR pathway may be an important target for MSC(WJ) to preserve β cells in treating T1D and point out a direction for further improving the therapeutic effect of MSC(WJ) on T1D in the future.
Cellular senescence is a critical issue in cytotherapy. Despite researchers having a deep understanding of the mechanisms and signs of MSCs senescence, they are still unable to reverse or prevent it [28]. As the number of passages increases, MSCs cultured in vitro age gradually. The secretome of senescent MSCs loses its beneficial functions and can even negatively impact tissue homeostasis and repair [29,30]. Therefore, this study used young third-passage MSC(WJ) for transplantation.
There are many animal models available for T1D research, but only a few are appropriate for studying childhood-onset T1D [31]. NOD mice injected with multiple low doses of STZ have both a hereditary autoimmune basis and partial β-cell destruction, as well as self-antigen exposure caused by STZ. This modeling method accelerates the original autoimmune response and leads to the early development of T1D [32,33]. Although there are few reports on this modeling method [26,27], it may be suitable for studying childhood-onset T1D. In this experiment, we assessed the practicality of this modeling method by monitoring blood glucose levels, evaluating glucose metabolism ability, and examining pancreatic pathological changes in NOD mice.
Our study demonstrated that the infused MSC(WJ) effectively migrated to the pancreas and spleen in T1D mice, where they remained for at least 7 days. This infusion significantly reduced insulitis, increased serum IL-10 levels, decreased serum IFN-γ levels, lowered the ratio of CD4+ T cells to CD8+ T cells, and enhanced the frequency of regulatory T cells in T1D mice. These findings align with studies that also reported infused MSC(WJ) homing to the pancreas and spleen in diabetic mice [34,35]. This homing mechanism of MSC(WJ) enables it to exert immune-modulating effects through direct inter-cellular contact, paracrine secretion, and the induction of M2 polarization of macrophages [36]. Other studies have reported similar results, where intravenously infused MSC(WJ) has attenuated insulitis, reduced T helper 17 cells, and increased regulatory T cells in T1D mice [26,37].
Equal amounts of insulin and C-peptide are produced through PI cleavage. To accurately assess basal insulin production, we measured fasting serum C-peptide levels, as they remain more stable in peripheral blood. Our findings indicate that the infusion of MSC(WJ) effectively preserved β-cell mass and increased insulin production. These results align with previous findings and support the notion that systemic administration of MSC(WJ) can maintain secretory function by preserving the remaining β-cell mass [26,38].
As an immunomodulator [39], MSC(WJ) has shown potential in preserving β-cell function in clinical trials [38,40]. However, its effect on ER stress or UPR pathway in β cells has not been examined. Immunomodulatory therapies for T1D are being transitioned from the bench to the clinic [41], making it crucial to assess their impact on β-cell stress. Our results demonstrated that treatment with MSC(WJ) significantly reduced the expression of BiP, ATF-6, and CHOP in the pancreas of T1D mice. This is consistent with a recent report on pancreatic mRNA expression, which suggests that MSCs enhance β-cell function by modulating inflammatory, UPR, and apoptosis signaling pathways in T1D rats [19]. Another study on mice with type 2 diabetes (T2D) showed that MSCs can preserve pancreatic islets and their function by modulating the UPR pathway [42]. Inflammatory cytokines can induce the translocation of BiP to the plasma membrane in β cells, thereby triggering apoptosis [43]. Over-activated UPR pathway leads to the upregulation of the transcription factor CHOP, leading to apoptosis of β cells in T2D [44]. However, modulating the UPR sensors, such as BiP, ATF-6, and CHOP, can protect β cells against immune assault in T1D [10]. MSCs can protect endothelial cells from hypoxic injury by suppressing the expression of BiP, ATF-6, and CHOP [45]. Therefore, this result explains why infused MSC(WJ) improved the survival of residual β cells in T1D mice.
Our results suggest that MSC(WJ) alone may not alleviate ER stress in β cells. However, some studies have reported that MSCs could relieve β-cell ER stress in the context of diabetes [19,46]. The controversy may be attributed to differences in biomarkers. Our study used a newly established biomarker of β-cell ER stress, the ratio of fasting serum PI to C-peptide [20], whereas the previous studies used the expression of sensors in UPR that cannot directly reflect the stress status of β cells. This new biomarker of β-cell stress has been applied in high-quality clinical trials to evaluate β-cell function in patients with T1D. For example, in a multicenter, double-blind, randomized controlled trial involving 84 youth with newly diagnosed T1D, after 52 weeks of treatment and evaluation, golimumab was found to stabilize β-cell stress in patients compared with controls [47]. Another trial conducted on 76 high-risk individuals in stage 2 of T1D [48] found that one course of teplizumab treatment did not affect the ER stress of β cells after 72 months of follow-up [49].
Inflammation and increased insulin demand can lead to ER stress in β cells [10]. Although infused MSCs regulate inflammation, they cannot differentiate into insulin-producing cells in vivo [50]. Consequently, the burden of insulin secretion remains heavy on the residual β cells. Therefore, our findings provide MSC(WJ) infusion alone may not alleviate ER stress in β cells.
The UPR pathway and inflammatory signaling share common regulators in cells. For example, the expression of BiP increases in coxsackievirus B3-induced myocarditis, possibly due to elevated levels of inflammatory cytokines such as IL-6 and tumor necrosis factor-α [51]. Additionally, IL-22 administration has been shown to suppress inflammation and ER stress in pancreatic β cells, leading to the reversal of hyperglycemia in T2D mice [52]. The anti-inflammatory cytokine IL-10 aids in protein folding and ER-associated degradation, thereby preventing protein misfolding [53]. Conversely, the inflammatory cytokine IFN-γ induces CHOP expression and downstream pro-apoptotic signaling in spinal cord neurons of patients with sporadic amyotrophic lateral sclerosis through the induction of inducible nitric oxide synthase [54]. Our findings indicate that MSC(WJ) regulates the BiP/ATF-6/CHOP pathway by upregulating IL-10 and downregulating IFN-γ, thereby preserving residual β-cell mass.
Recent clinical trials have shown promising early results in using verapamil and imatinib to treat patients with recent-onset T1D [55,56]. However, it is important to consider the potential harm to other cells when using these UPR inhibitors, as they primarily target β cells. Combining immunotherapies with β-cell stress therapies in T1D, specifically targeting β cells, could potentially provide an opportunity for prevention or treatment of the disease.
In clinical trials, exogenous insulin has been used as a standard treatment to improve glucose metabolism in patients with T1D, however, this approach may interfere with the evaluation of β-cell stress. Our experiment focused on the direct impact of infused MSC(WJ) on ER stress and UPR in β cells, providing valuable insights into potential therapies for childhood-onset T1D.
Our study has several limitations. First, the small sample size and short observation period may have led to subtle variations within the experiments, potentially yielding false-negative outcomes. Second, although repeated dosing with MSC(WJ) may offer additional benefits or prolong therapeutic efficacy [57], we did not test this strategy. Third, due to the limited volume of blood obtained from mice, our study only assessed fasting serum PI and C-peptide levels at the end of the experiment; this may restrict the comprehensive evaluation of β-cell function and ER stress. Fourth, to accurately reflect changes in the UPR pathways of β cells, protein expression in islets or β cells should be assessed. However, isolating islets or β cells can significantly alter their microenvironment [58], thereby interfering with ER stress and UPR in β cells. Therefore, we directly analyzed the islet-rich pancreatic tail. Finally, the body weight and the ratio of fasting serum PI to C-peptide in mice showed no significant difference between the MSC(WJ) treatment group and the T1D model group. This lack of difference could be attributed to the limited number of cells transfused in our study, as existing literature suggests that MSCs exhibit a dose-dependent effect within a specific range [59,60]. In future experiments, we intend to increase the quantity of transplanted cells and the frequency of infusion to assess their impact. Additionally, we plan to conduct further validation experiments using spontaneous T1D models such as BB rats or NOD mice.
In conclusion, by assessing the novel marker of β-cell ER stress and the expression level of key proteins in the UPR pathway, we demonstrate that MSC(WJ) infusion cannot alleviate ER stress in β cells. However, it does modulate the UPR pathway, leading to the preservation of remaining β-cell mass and function in newly-onset T1D mice. These findings indicate that MSC(WJ) transplantation is a cytotherapy with multiple targets (modulating immunity and UPR pathway) in treating T1D. Further research is necessary to validate our findings and clarify the molecular mechanisms by which MSC(WJ) affects the UPR pathway, ultimately improving its efficacy. Clinical trials will be essential to determine the impact of MSC(WJ) infusion on the stress status of β cells in patients with T1D. In the future, the incorporation of this cytotherapy as a supplementary treatment for childhood-onset T1D is anticipated to enhance the long-term prognosis of pediatric patients.
Acknowledgement: None.
Funding Statement: The authors received no specific funding for this study.
Author Contributions: The authors confirm their contribution to the paper as follows: study conception and design: ZL, SYL; data collection: YZ, YY; analysis and interpretation of results: DY, QW, ZYW; draft manuscript preparation: ZL, SYL. All authors reviewed the results and approved the final version of the manuscript.
Availability of Data and Materials: The datasets generated during the current study are available in the (Mendeley) repository, https://data.mendeley.com/preview/svyjsn9b69?a=ca03a699-4ef7-4293-8ab8-955a41a50dff.
Ethics Approval: A segment of the umbilical cord was obtained from a healthy woman who gave birth at the Chinese PLA General Hospital in February 2023. Written informed consent was obtained from the donor before delivery. The protocol was approved by the Ethics Committee of the Chinese PLA General Hospital (No. S2022-107-19; Date: June 15th, 2022). All procedures involving animals were performed under the Guide for the Care and Use of Laboratory Animals (8th Edition, USA) and were approved by the Ethics Review Committee for Animal Experiments of the Chinese PLA General Hospital (No. 2022-X18-77; Date: July 20th, 2022).
Conflicts of Interest: The authors declare that they have no conflicts of interest to report regarding the present study.
References
1. Quattrin T, Mastrandrea LD, Walker LSK. Type 1 diabetes. Lancet. 2023;401(10394):2149–62. doi:10.1016/S0140-6736(23)00223-4. [Google Scholar] [PubMed] [CrossRef]
2. Norris JM, Johnson RK, Stene LC. Type 1 diabetes-early life origins and changing epidemiology. Lancet Diabetes Endocrinol. 2020;8(3):226–38. doi:10.1016/S2213-8587(19)30412-7. [Google Scholar] [PubMed] [CrossRef]
3. Rawshani A, Sattar N, Franzén S, Rawshani A, Hattersley AT, Svensson AM, et al. Excess mortality and cardiovascular disease in young adults with type 1 diabetes in relation to age at onset: a nationwide, register-based cohort study. Lancet. 2018;392(10146):477–86. doi:10.1016/S0140-6736(18)31506-X. [Google Scholar] [PubMed] [CrossRef]
4. Rodriguez-Calvo T, Richardson SJ, Pugliese A. Pancreas pathology during the natural history of type 1 diabetes. Curr Diab Rep. 2018;18(11):124. doi:10.1007/s11892-018-1084-3. [Google Scholar] [PubMed] [CrossRef]
5. Greenbaum CJ, Speake C, Krischer J, Buckner J, Gottlieb PA, Schatz DA, et al. Strength in numbers: opportunities for enhancing the development of effective treatments for type 1 diabetes—The TrialNet experience. Diabetes. 2018;67(7):1216–25. doi:10.2337/db18-0065. [Google Scholar] [PubMed] [CrossRef]
6. Andreone L, Fuertes F, Sétula C, Barcala Tabarrozzi AE, Orellano MS, Dewey RA, et al. Compound a attenuates proinflammatory cytokine-induced endoplasmic reticulum stress in beta cells and displays beneficial therapeutic effects in a mouse model of autoimmune diabetes. Cell Mol Life Sci. 2022;79(12):587. doi:10.1007/s00018-022-04615-5. [Google Scholar] [PubMed] [CrossRef]
7. Yong J, Johnson JD, Arvan P, Han J, Kaufman RJ. Therapeutic opportunities for pancreatic β-cell ER stress in diabetes mellitus. Nat Rev Endocrinol. 2021;17(8):455–67. doi:10.1038/s41574-021-00510-4. [Google Scholar] [PubMed] [CrossRef]
8. Wiseman RL, Mesgarzadeh JS, Hendershot LM. Reshaping endoplasmic reticulum quality control through the unfolded protein response. Mol Cell. 2022;82(8):1477–91. doi:10.1016/j.molcel.2022.03.025. [Google Scholar] [PubMed] [CrossRef]
9. Sharma RB, Landa-Galván HV, Alonso LC. Living dangerously: protective and harmful ER stress responses in pancreatic β-cells. Diabetes. 2021;70(11):2431–43. doi:10.2337/dbi20-0033. [Google Scholar] [PubMed] [CrossRef]
10. Sahin GS, Lee H, Engin F. An accomplice more than a mere victim: the impact of β-cell ER stress on type 1 diabetes pathogenesis. Mol Metab. 2021;54:101365. doi:10.1016/j.molmet.2021.101365. [Google Scholar] [PubMed] [CrossRef]
11. Von Scholten BJ, Kreiner FF, Gough SCL, Von Herrath M. Current and future therapies for type 1 diabetes. Diabetologia. 2021;64(5):1037–48. doi:10.1007/s00125-021-05398-3. [Google Scholar] [PubMed] [CrossRef]
12. Smigoc Schweiger D. Recent advances in immune-based therapies for type 1 diabetes. Horm Res Paediatr. 2023;96(6):631–45. doi:10.1159/000524866. [Google Scholar] [PubMed] [CrossRef]
13. Han Y, Yang J, Fang J, Zhou Y, Candi E, Wang J, et al. The secretion profile of mesenchymal stem cells and potential applications in treating human diseases. Signal Transduct Target Ther. 2022;7(1):92. doi:10.1038/s41392-022-00932-0. [Google Scholar] [PubMed] [CrossRef]
14. Tian X, He X, Qian S, Zou R, Chen K, Zhu C, et al. Immunoregulatory effects of human amniotic mesenchymal stem cells and their exosomes on human peripheral blood mononuclear cells. Biocell. 2023;47(5):1085–93. doi:10.32604/biocell.2023.027090 [Google Scholar] [CrossRef]
15. Thompson M, Mei SHJ, Wolfe D, Champagne J, Fergusson D, Stewart DJ, et al. Cell therapy with intravascular administration of mesenchymal stromal cells continues to appear safe: an updated systematic review and meta-analysis. EClinicalMedicine. 2020;19:100249. doi:10.1016/j.eclinm.2019.100249. [Google Scholar] [PubMed] [CrossRef]
16. Zhuang WZ, Lin YH, Su LJ, Wu MS, Jeng HY, Chang HC, et al. Mesenchymal stem/stromal cell-based therapy: mechanism, systemic safety and biodistribution for precision clinical applications. J Biomed Sci. 2021;28(1):28. doi:10.1186/s12929-021-00725-7. [Google Scholar] [PubMed] [CrossRef]
17. Izadi M, Sadr Hashemi Nejad A, Moazenchi M, Masoumi S, Rabbani A, Kompani F, et al. Mesenchymal stem cell transplantation in newly diagnosed type-1 diabetes patients: a phase I/II randomized placebo-controlled clinical trial. Stem Cell Res Ther. 2022;13(1):264. doi:10.1186/s13287-022-02941-w. [Google Scholar] [PubMed] [CrossRef]
18. Zha L, Li X, Meng X, Liu Y, Liu W, Wang Y, et al. Effects of early MSC intervention on preventing the Streptozotocin-induced T1DM progression in mice. Oxid Med Cell Longev. 2020;2020:5438951. [Google Scholar]
19. Khamis T, Abdelalim AF, Saeed AA, Edress NM, Nafea A, Ebian HF, et al. Breast milk MSCs upregulated β-cells PDX1, Ngn3, and PCNA expression via remodeling ER stress/inflammatory/apoptotic signaling pathways in type 1 diabetic rats. Eur J Pharmacol. 2021;905:174188. doi:10.1016/j.ejphar.2021.174188. [Google Scholar] [PubMed] [CrossRef]
20. Sims EK, Evans-Molina C, Tersey SA, Eizirik DL, Mirmira RG. Biomarkers of islet β-cell stress and death in type 1 diabetes. Diabetologia. 2018;61(11):2259–65. doi:10.1007/s00125-018-4712-1. [Google Scholar] [PubMed] [CrossRef]
21. Viswanathan S, Ciccocioppo R, Galipeau J, Krampera M, Le Blanc K, Martin I, et al. Consensus international council for commonality in blood banking automation-international society for cell & gene therapy statement on standard nomenclature abbreviations for the tissue of origin of mesenchymal stromal cells. Cytotherapy. 2021;23(12):1060–3. doi:10.1016/j.jcyt.2021.04.009. [Google Scholar] [PubMed] [CrossRef]
22. Li K, Yan G, Huang H, Zheng M, Ma K, Cui X, et al. Anti-inflammatory and immunomodulatory effects of the extracellular vesicles derived from human umbilical cord mesenchymal stem cells on osteoarthritis via M2 macrophages. J Nanobiotechnol. 2022;20(1):38. doi:10.1186/s12951-021-01236-1. [Google Scholar] [PubMed] [CrossRef]
23. Dominici M, Le Blanc K, Mueller I, Slaper-Cortenbach I, Marini F, Krause D, et al. Minimal criteria for defining multipotent mesenchymal stromal cells. Int Soc Cell Ther Position statement. Cytotherapy. 2006;8(4):315–7. [Google Scholar]
24. Kawada-Horitani E, Kita S, Okita T, Nakamura Y, Nishida H, Honma Y, et al. Human adipose-derived mesenchymal stem cells prevent type 1 diabetes induced by immune checkpoint blockade. Diabetologia. 2022;65(7):1185–97. doi:10.1007/s00125-022-05708-3. [Google Scholar] [PubMed] [CrossRef]
25. Gaudreau MC, Gudi RR, Li G, Johnson BM, Vasu C. Gastrin producing syngeneic mesenchymal stem cells protect non-obese diabetic mice from type 1 diabetes. Autoimmunity. 2022;55(2):95–108. doi:10.1080/08916934.2021.2012165. [Google Scholar] [PubMed] [CrossRef]
26. Zhang W, Ling Q, Wang B, Wang K, Pang J, Lu J, et al. Comparison of therapeutic effects of mesenchymal stem cells from umbilical cord and bone marrow in the treatment of type 1 diabetes. Stem Cell Res Ther. 2022;13(1):406. doi:10.1186/s13287-022-02974-1. [Google Scholar] [PubMed] [CrossRef]
27. Reddy S, Chang M, Robinson E. Young NOD mice show increased diabetes sensitivity to low doses of streptozotocin. Ann N Y Acad Sci. 2006;1079:109–13. doi:10.1196/annals.1375.015. [Google Scholar] [PubMed] [CrossRef]
28. Al-Azab M, Safi M, Idiiatullina E, Al-Shaebi F, Zaky MY. Aging of mesenchymal stem cell: machinery, markers, and strategies of fighting. Cell Mol Biol Lett. 2022;27(1):69. doi:10.1186/s11658-022-00366-0. [Google Scholar] [PubMed] [CrossRef]
29. Lyamina S, Baranovskii D, Kozhevnikova E, Ivanova T, Kalish S, Sadekov T, et al. Mesenchymal stromal cells as a driver of inflammaging. Int J Mol Sci. 2023;24(7):6372. doi:10.3390/ijms24076372. [Google Scholar] [PubMed] [CrossRef]
30. Siraj Y, Galderisi U, Alessio N. Senescence induces fundamental changes in the secretome of mesenchymal stromal cells (MSCsimplications for the therapeutic use of MSCs and their derivates. Front Bioeng Biotechnol. 2023;11:1148761. doi:10.3389/fbioe.2023.1148761. [Google Scholar] [PubMed] [CrossRef]
31. Peng X, Rao G, Li X, Tong N, Tian Y, Fu X. Preclinical models for type 1 diabetes mellitus—A practical approach for research. Int J Med Sci. 2023;20(12):1644–61. doi:10.7150/ijms.86566. [Google Scholar] [PubMed] [CrossRef]
32. Zhu BT. Pathogenic mechanism of autoimmune diabetes mellitus in humans: potential role of streptozotocin-induced selective autoimmunity against human islet β-cells. Cells. 2022;11(3):492. doi:10.3390/cells11030492. [Google Scholar] [PubMed] [CrossRef]
33. Pearson JA, Wong FS, Wen L. The importance of the non obese diabetic (NOD) mouse model in autoimmune diabetes. J Autoimmun. 2016;66:76–88. doi:10.1016/j.jaut.2015.08.019. [Google Scholar] [PubMed] [CrossRef]
34. Yin Y, Hao H, Cheng Y, Gao J, Liu J, Xie Z, et al. The homing of human umbilical cord-derived mesenchymal stem cells and the subsequent modulation of macrophage polarization in type 2 diabetic mice. Int Immunopharmacol. 2018;60:235–45. doi:10.1016/j.intimp.2018.04.051. [Google Scholar] [PubMed] [CrossRef]
35. Maldonado M, Huang T, Yang L, Xu L, Ma L. Human umbilical cord Wharton jelly cells promote extra-pancreatic insulin formation and repair of renal damage in STZ-induced diabetic mice. Cell Commun Signal. 2017;15(1):43. doi:10.1186/s12964-017-0199-5. [Google Scholar] [PubMed] [CrossRef]
36. Krampera M, Le Blanc K. Mesenchymal stromal cells: putative microenvironmental modulators become cell therapy. Cell Stem Cell. 2021;28(10):1708–25. doi:10.1016/j.stem.2021.09.006. [Google Scholar] [PubMed] [CrossRef]
37. Madec AM, Mallone R, Afonso G, Abou Mrad E, Mesnier A, Eljaafari A, et al. Mesenchymal stem cells protect NOD mice from diabetes by inducing regulatory T cells. Diabetologia. 2009;52(7):1391–9. doi:10.1007/s00125-009-1374-z. [Google Scholar] [PubMed] [CrossRef]
38. Carlsson PO, Espes D, Sisay S, Davies LC, Smith CIE, Svahn MG. Umbilical cord-derived mesenchymal stromal cells preserve endogenous insulin production in type 1 diabetes: a phase I/II randomised double-blind placebo-controlled trial. Diabetologia. 2023;66(8):1431–41. doi:10.1007/s00125-023-05934-3. [Google Scholar] [PubMed] [CrossRef]
39. Pittenger MF, Discher DE, Péault BM, Phinney DG, Hare JM, Caplan AI. Mesenchymal stem cell perspective: cell biology to clinical progress. NPJ Regen Med. 2019;4:22. doi:10.1038/s41536-019-0083-6. [Google Scholar] [PubMed] [CrossRef]
40. Lu J, Shen SM, Ling Q, Wang B, Li LR, Zhang W, et al. One repeated transplantation of allogeneic umbilical cord mesenchymal stromal cells in type 1 diabetes: an open parallel controlled clinical study. Stem Cell Res Ther. 2021;12(1):340. doi:10.1186/s13287-021-02417-3. [Google Scholar] [PubMed] [CrossRef]
41. Carvalho T. FDA approves first drug to delay type 1 diabetes. Nat Med. 2023;29(2):280. doi:10.1038/d41591-022-00115-y. [Google Scholar] [PubMed] [CrossRef]
42. Lee Y, Shin SH, Cho KA, Kim YH, Woo SY, Kim HS, et al. Administration of tonsil-derived mesenchymal stem cells improves glucose tolerance in high fat diet-induced diabetic mice via insulin-like growth factor-binding Protein 5-mediated endoplasmic reticulum stress modulation. Cells. 2019;8(4):368. doi:10.3390/cells8040368. [Google Scholar] [PubMed] [CrossRef]
43. Vig S, Buitinga M, Rondas D, Crèvecoeur I, van Zandvoort M, Waelkens E, et al. Cytokine-induced translocation of GRP78 to the plasma membrane triggers a pro-apoptotic feedback loop in pancreatic beta cells. Cell Death Dis. 2019;10(4):309. doi:10.1038/s41419-019-1518-0. [Google Scholar] [PubMed] [CrossRef]
44. Laybutt DR, Preston AM, Akerfeldt MC, Kench JG, Busch AK, Biankin AV, et al. Endoplasmic reticulum stress contributes to beta cell apoptosis in type 2 diabetes. Diabetologia. 2007;50(4):752–63. doi:10.1007/s00125-006-0590-z. [Google Scholar] [PubMed] [CrossRef]
45. Keese M, Zheng J, Yan K, Bieback K, Yard BA, Pallavi P, et al. Adipose-derived mesenchymal stem cells protect endothelial cells from hypoxic injury by suppressing terminal UPR in vivo and in vitro. Int J Mol Sci. 2023;24(24):17197. doi:10.3390/ijms242417197. [Google Scholar] [PubMed] [CrossRef]
46. Li B, Cheng X, Aierken A, Du J, He W, Zhang M, et al. Melatonin promotes the therapeutic effect of mesenchymal stem cells on type 2 diabetes mellitus by regulating TGF-β pathway. Front Cell Dev Biol. 2021;9:722365. doi:10.3389/fcell.2021.722365. [Google Scholar] [PubMed] [CrossRef]
47. Quattrin T, Haller MJ, Steck AK, Felner EI, Li Y, Xia Y, et al. Golimumab and beta-cell function in youth with new-onset type 1 diabetes. N Engl J Med. 2020;383(21):2007–17. doi:10.1056/NEJMoa2006136. [Google Scholar] [PubMed] [CrossRef]
48. Insel RA, Dunne JL, Atkinson MA, Chiang JL, Dabelea D, Gottlieb PA, et al. Staging presymptomatic type 1 diabetes: a scientific statement of JDRF, the endocrine society, and the American diabetes association. Diabetes Care. 2015;38(10):1964–74. doi:10.2337/dc15-1419. [Google Scholar] [PubMed] [CrossRef]
49. Sims EK, Bundy BN, Stier K, Serti E, Lim N, Long SA, et al. Teplizumab improves and stabilizes beta cell function in antibody-positive high-risk individuals. Sci Transl Med. 2021;13(583):eabc8980. doi:10.1126/scitranslmed.abc8980. [Google Scholar] [PubMed] [CrossRef]
50. de Klerk E, Hebrok M. Stem cell-based clinical trials for diabetes mellitus. Front Endocrinol. 2021;12:631463. doi:10.3389/fendo.2021.631463. [Google Scholar] [PubMed] [CrossRef]
51. Zha X, Yue Y, Dong N, Xiong S. Endoplasmic reticulum stress aggravates viral myocarditis by raising inflammation through the IRE1-associated NF-κB pathway. Can J Cardiol. 2015;31(8):1032–40. doi:10.1016/j.cjca.2015.03.003. [Google Scholar] [PubMed] [CrossRef]
52. Hasnain SZ, Borg DJ, Harcourt BE, Tong H, Sheng YH, Ng CP, et al. Glycemic control in diabetes is restored by therapeutic manipulation of cytokines that regulate beta cell stress. Nat Med. 2014;20(12):1417–26. doi:10.1038/nm.3705. [Google Scholar] [PubMed] [CrossRef]
53. Hasnain SZ, Tauro S, Das I, Tong H, Chen AC, Jeffery PL, et al. IL-10 promotes production of intestinal mucus by suppressing protein misfolding and endoplasmic reticulum stress in goblet cells. Gastroenterol. 2013;144(2):357–68.e9. doi:10.1053/j.gastro.2012.10.043. [Google Scholar] [PubMed] [CrossRef]
54. Cao SS, Luo KL, Shi L. Endoplasmic reticulum stress interacts with inflammation in human diseases. J Cell Physiol. 2016;231(2):288–94. doi:10.1002/jcp.v231.2. [Google Scholar] [CrossRef]
55. Ovalle F, Grimes T, Xu G, Patel AJ, Grayson TB, Thielen LA, et al. Verapamil and beta cell function in adults with recent-onset type 1 diabetes. Nat Med. 2018;24(8):1108–12. doi:10.1038/s41591-018-0089-4. [Google Scholar] [PubMed] [CrossRef]
56. Gitelman SE, Bundy BN, Ferrannini E, Lim N, Blanchfield JL, DiMeglio LA, et al. Imatinib therapy for patients with recent-onset type 1 diabetes: a multicentre, randomised, double-blind, placebo-controlled, phase 2 trial. Lancet Diabetes Endocrinol. 2021;9(8):502–14. doi:10.1016/S2213-8587(21)00139-X. [Google Scholar] [PubMed] [CrossRef]
57. Li L, Hui H, Jia X, Zhang J, Liu Y, Xu Q, et al. Infusion with human bone marrow-derived mesenchymal stem cells improves β-cell function in patients and non-obese mice with severe diabetes. Sci Rep. 2016;6:37894. doi:10.1038/srep37894. [Google Scholar] [PubMed] [CrossRef]
58. O’Dowd JF, Stocker CJ. Isolation and purification of rodent pancreatic islets of langerhans. Methods Mol Biol. 2020;2076:179–84. doi:10.1007/978-1-4939-9882-1. [Google Scholar] [CrossRef]
59. Kobayashi K, Maeda T, Ayodeji M, Tu SC, Chen A, Rajtboriraks M, et al. Dose effect of mesenchymal stromal cell delivery through cardiopulmonary bypass. Ann Thorac Surg. 2023;116(6):1337–45. doi:10.1016/j.athoracsur.2022.07.035. [Google Scholar] [PubMed] [CrossRef]
60. Byrnes D, Masterson CH, Gonzales HE, McCarthy SD, O’Toole DP, Laffey JG. Multiple dosing and preactivation of mesenchymal stromal cells enhance efficacy in established pneumonia induced by antimicrobial-resistant klebsiella pneumoniae in rodents. Int J Mol Sci. 2023;24(9):8055. doi:10.3390/ijms24098055. [Google Scholar] [PubMed] [CrossRef]
Appendix
Figure A1: Characterizations of Mesenchymal stromal cells derived from Wharton’s jelly of umbilical cord (MSC(WJ)). (A) Expression levels of CD73, CD90, CD105, CD14, CD19, CD34, CD45, and HLA-DR on the surface of MSC(WJ) analyzed using flow cytometry. (B) Representative picture of cultured MSC(WJ) observed under a microscope. Scale bar = 100 μm. (C) Micrograph of adipogenic differentiation identified by Oil Red O staining. Scale bar = 50 μm. (D and E) Representative pictures of osteogenic differentiation identified by Alizarin Red S staining, and chondrogenic differentiation identified by Alcian Blue staining. Scale bar = 100 μm.
Figure A2: Mesenchymal stromal cells derived from Wharton’s jelly of umbilical cord (MSC(WJ)) homing in vivo. Representative micrographs of the lungs, liver, and spleen of mice with type 1 diabetes at 12 h (A–C) and 7 days (D–F) after MSC(WJ) infusion. Scale bar = 100 μm.
Cite This Article
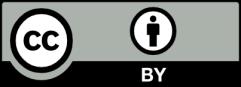
This work is licensed under a Creative Commons Attribution 4.0 International License , which permits unrestricted use, distribution, and reproduction in any medium, provided the original work is properly cited.