Open Access
REVIEW
Molecular and cellular mechanisms of neuroprotection by oligopeptides from snake venoms
Natural and Humanities Sciences Center, Experimental Morphophysiology Laboratory, Federal University of ABC (UFABC), São Bernardo do Campo, São Paulo, Brazil
* Corresponding Author: CARLOS ALBERTO-SILVA. Email:
(This article belongs to the Special Issue: Exploring the Cellular Mechanisms of Neurodegenerative Diseases)
BIOCELL 2024, 48(6), 897-904. https://doi.org/10.32604/biocell.2024.050443
Received 06 February 2024; Accepted 22 March 2024; Issue published 10 June 2024
Abstract
Venom snake-derived peptides have multiple biochemical, pharmacological, and toxicological profiles, allowing for the discovery of new medicinal products and therapeutic applications. This review specifically examines the fundamental elements of neuroprotection offered by different oligopeptides derived from snake venom. It also includes a brief evaluation of short peptides that are being considered as potential therapeutic agents. Proline-rich peptides and tryptophyllin family peptides isolated from the crude venom of Viperidae family snakes, specifically Bothrops atrox, Bothrops jararaca, and Bothrops moojeni, have been shown to have pro-survival properties, the ability to reduce oxidative stress, and the ability to promote cell viability and mitochondrial functions. Three significant mechanisms are related to the neuroprotection mediated by snake venom oligopeptides: (1) Activation of the L-arginine metabolite pathway, such as polyamines from ornithine metabolism, which reduces N-methyl-D-aspartate (NMDA)-type glutamate receptor activity; (2) Enhancement of cell viability by activating the nerve growth factor-signaling pathway; and (3) Activation of the Muscarinic acetylcholine receptor subtype M1 (mAChR-M1). These small peptides show promise as neuroprotective agents against a variety of neurodegenerative disorders.Keywords
Snake venom is secreted from a specific gland positioned above the upper jaw bone [1]. It is released by a compressor muscle surrounding the gland and transported through a duct to the fangs [1]. When injected into an individual’s tissues, usually under accidental circumstances, snakebite envenoming causes considerable annual mortality, and surviving victims suffer ranging from mental and physical issues [2]. It is considering a severe public health issue that the World Health Organization (WHO) classified as a neglected disease [2]. The extensive complexity of venom composition [3] is responsible for prominent local tissue damage and systemic disturbances [2]. Toxins can cause cognitive abnormalities, bleeding, renal modifications, cardiovascular shock, lung and muscle inflammation, and reproductive problems, depending on the location they act [2,4,5].
The specific composition of venom can vary based on factors such as the species of snake, environmental conditions, age, sex, and the sort of prey that is accessible [6]. Snake venom is primarily composed of a variety of components, with bioactive peptides and proteins being the most abundant [7]. Aside from these, lipids, amino acids, carbohydrates, metal ions, nucleosides, and amines can constitute up 5%–10% of snake venom crude [8].
Researchers have developed venomics techniques to characterize venom toxins qualitatively and quantitatively in various areas of venom biology [8]. Most snake toxins are in the mass range of 4 to 100 kDa, while a number of multimeric toxins may exceed a total mass of 250 kDa [8]. Studies have demonstrated the categorization of snake venom protein families into different hierarchical groups based on their occurrence and abundance [7]. Based on a comprehensive analysis of the proteome composition of venom from 179 species (including 68 elapids and 111 vipers), 42 different protein families have been found [7].
They represent a combination of occurrences across many species, together with the proportional abundance of these protein families expressed as a percentage of the overall venom composition [7]. Among them, 4 protein families were classified as dominant: Phospholipase A2 (PLA2) is responsible for acute skeletal, muscle necrosis, flaccid paralysis, oedema, leukocyte influx into tissues, and pain [7]; Snake venom metalloprotease (SVMP) has hemorrhagic activity, as well as the ability to degrade fibrinogen and fibrin by proteolysis, induce apoptosis, and prevent platelet aggregation [7]; Three-finger toxins (3FTx) are non-enzymatic toxins that cause neurotoxicity effects, paralysis, muscle fasciculations, and cardiac failure [7]; And snake venom serine protease (SVSP) affects he coagulation cascade in the fibrinolytic and kallikrein-kinin pathways [7].
Various pharmacologically active peptides derived from the low molecular mass fraction (LMMF) of venom have also been reported, such as disintegrins [9] and proline-rich oligopeptides (PROs), commonly known as bradykinin (Bk) potentiating peptides (BPPs) [10].
Snake venom disintegrins are cysteine-rich non-enzymatic proteins derived from snake venom metalloproteinase, grouped based on sequence size and disulfide bond number [11]. Disintegrins possess a particular arrangement that enables them to recognize integrins within their structure [9]. This recognition facilitates their interaction with transmembrane adhesion receptors, thereby inhibiting the binding of these receptors to proteins found in the extracellular matrix and other cells [9]. Disintegrins possess diverse biological activities, such as the ability to prevent platelet aggregation and exhibit anticancer activity [9]. PROs were the first naturally found inhibitors of Angiotensin I-Converting Enzyme (ACE) described from snake venom studies [12]. The structures and functions of PROs served as the basis for the discovery of antihypertensive medications like Captopril and its derivatives [13].
PROs have 5 to 14 amino acids and are structurally classified as oligopeptides. They have a high proline content, a pyro-glutamic acid residue in the amine-terminal position (<E), a PXP module, and at least one proline residue in the carboxy-terminal [14]. Over 85 snake proteins have been discovered, and analysis of their sequence features has revealed common components like N-terminal pyroglutamic acid and C-terminal QIPP, as well as varying components like AP or WAQ found in some of them [14]. These peptides include multiple proline residues in their interior regions [14–17] resulting in them being resistant to hydrolysis by aminopeptidases, carboxypeptidases, and endopeptidases [18,19] (Table 1).
For many years, it was believed that the antihypertensive effects of various BPPs were due to the suppression of ACE and the enhancement of Bk [15]. However, despite their structural similarity, the different biological function profiles suggest that they change their targets through various mechanisms [23]. These mechanisms include functioning as agonists of the M1 muscarinic acetylcholine receptor (mAChR-M1) [29,30] and activating argininosuccinate synthetase (AsS) [23,25].
Neuroprotective potential in the LMMF components of snake venom
Research has shown that compounds found in snake venom have the potential to be used as treatments for neurological diseases [31,32]. Martins and collaborators found that the LMMF of Bothrops atrox (B. atrox) venom (Ba-LMMF), consisting exclusively of components with a molecular weight less than 14 kDa, is neuroprotective by reducing mitochondrial permeability transition (MPT) [33]. MPT is characterized by the enlargement of mitochondria due to an elevation in reactive oxygen species (ROS) and calcium ion (Ca+2) levels [34]. Although MPT occurs before the initiation of apoptosis, it is believed that Ba-LMMF venom inhibits neuronal apoptosis [33].
Similarly, the LMMF obtained from Bothrops jararaca (B. jararaca) snake venom (Bj-LMMF), containing many bioactive peptides <10 kDa, showed neuroprotective efficacy against H2O2-induced toxicity in primary cultured hippocampus cells (Figs. 1A–1E) [35]. The Bj-LMMF lowered superoxide dismutase (SOD), caspases 3, and 8 levels, indicating that may boost hippocampus cell survival by reducing oxidative stress [35]. On the other hand, Bj-LMMF did not show a protective capacity against H2O2-induced damage in neuronal cell line SH-SY5Y (Fig. 1C) [36]. Querobino and collaborators proposed that Bj-LMMF treatment would elicit distinct responses in the primary culture of hippocampus cells, which comprises various cell types such as neurons, glia cells, and astrocytes, compared to cell lines [36]. Bj-LMMF-mediated neuroprotection was tested against oxidative stress in two different cell types, the PC12 and C6 cell lines (dopaminergic neuronal and astrocyte-like, respectively) [37]. It is interesting to note that Bj-LMMF protected neuronal PC12 cells from oxidative stress caused by H2O2 but not C6 cells (Fig. 1B).
Figure 1: Bj-LMMF-mediated neuroprotection against oxidative stress in different cell lines reported in the literature [35,37]. (A) Studies have investigated the effects of oxidative stress on cells, specifically focusing on neuroprotective models. The cells received a 4 h pre-treatment with Bj-LMMF. Subsequently, the medium was substituted with Bj-LMMF, a novel medium, and/or H2O2 solutions, and left to incubate for an additional 20 h. Neuroprotective effects of Bj-LMMF against H2O2-induced toxicity in C6 (B), SH-SY5Y (C), PC12 (D) and hippocampal (E) cells.
Researchers commonly employ the C6 cell line to investigate various aspects of astrocytic function. It demonstrates rapid responsiveness to external stimuli, including H2O2, which can induce oxidative-nitrosative stress [37] indicating that C6 cells are more vulnerable to the effects of Bj-LMMF compared to PC12 or SH-SY5Y cells (Figs. 1B–1D). The neuroprotective effects of Bj-LMMF in PC12 cells were demonstrated by enhancing cell survival and metabolism (Fig. 1D) in response to H2O2-induced neurotoxicity. This was accomplished by reducing the generation of ROS, the production of NO, and the activity of arginase through the process of urea formation [37]. Studies reveal that the neuroprotection mechanism of this fraction relies on the synthesis route of L-arginine metabolites [37].
Argininosuccinate synthetase (AsS) and nitric oxide synthase (NOS) play crucial roles in the metabolic route of L-arginine, as shown in Fig. 2 [38]. AsS enzyme produces argininosuccinate which is metabolized by argininosuccinate lyase (AsL) to L-arginine [39]. L-arginine enhances several cellular processes and promotes the production of nitric oxide, agmatine, polyamines, and ornithine [38,39]. The neuroprotective properties of Bj-LMMF were diminished when AsS and NOS were suppressed. The mechanism of action is affected by the production pathway of L-arginine metabolites such as specifically NO and polyamines derived from ornithine hydrolysis [37]. The polyamines have well-known for their substantial contribution to the neuroprotection process, as evidenced in studies [40].
Figure 2: Metabolism of L-arginine in mammalian cells, including its involvement with the generation of nitric oxide (NO) and the citrulline-NO cycle [41]. NMMA; NG-monomethyl-L-arginine, ADMA; asymmetric NG, NG-dimethyl-L-arginine.
Neuroprotection mediated by venom-derived peptides
Natural component-derived peptides are being considered as possible therapeutic potential for neuroprotection [42]. Pharmaceutical industries are particularly interested in peptides that possess advantageous characteristics such as low molecular mass, high activity and specificity, effortless absorption, and a good safety profile with minimal toxicity [43]. Venom-derived peptides and venom peptide-inspired molecules contributed to the discovery of new drugs approved by regulatory institutions or undergoing preclinical and clinical studies [44]. Captopril (antihypertensive), Tirofiban (antiplatelet), Eptifibatide (another antiplatelet), Batroxobin (Defibrase, for the care of acute brain injury and stroke), and α-Cobrotoxin (analgesic for moderate to severe pain) are exemplary instances of authorized pharmaceuticals that are generated from peptides found in snake venoms [8]. Some oligopeptides generated from snake venom have been demonstrated to provide neuroprotection using different models. Proline-rich peptides and tryptophyllin family peptides derived from the snake venoms (B. atrox, B. jararaca, and B. moojeni species) have demonstrated pro-survival features, the capacity to reduce oxidative stress, and enhance cell viability and mitochondrial functions.
The neuroprotective properties of several PROs were investigated for H2O2-induced toxicity in different types of neurons in culture, as seen in Table 2 [45,46]. However, the p-BTX-I, PRO-5a, PRO-7a, and PRO-10c peptides showed better neuroprotective effects improving cell viability and decreasing oxidative stress biomarkers against ROS-induced changes [45,46]. Curiously, although they have a similar structure, the biological mechanisms responsible for their neuroprotective activities are distinctive.
PRO-10c-mediated neuroprotection in SH-SY5Y cell cultures can be seen by a reduction of ROS, lipid peroxidation, inducible NOS, NO levels, and total glutathione [36]. PRO-10c was reported as an AsS activator, which improves the enzyme’s ability to bind to ATP and citrulline, while also raising the levels of L-arginine [25]. Elevated levels of L-arginine can enhance the synthesis of agmatine, which is known for its neuroprotective properties [47]. Agmatine blocks the N-methyl-D-aspartate (NMDA) receptor channel in hippocampuss [48]. The neuroprotective impact of PRO-10c is attributed to enhanced L-arginine production, which is accomplished via upregulating AsS (Fig. 3A), producing agmatine, and maintaining mitochondrial membrane integrity [36]. Interestingly, it was observed that the neuroprotective efficacy of PRO-10c against H2O2-induced toxicity in SH-SY5Y cells was attenuated by AsS inhibition using α-Methyl-DL-aspartic acid (MDLA) [36], demonstrating that its neuroprotective effects are dependent on L-arginine metabolism.
Figure 3: Proposals for PROs-mediated mechanisms of neuroprotection. (A) PRO-7a activates mAChR-M1, leading to various consequences through the signaling of PKC and MAPK. These effects occur independently of the rise in L-arginine caused by AsS activation, and they trigger a biological response to the effects of oxidative stress [51]. (B) PRO-10c is taken up into cells by an unknown process, increasing the production of L-arginine by upregulating the expression and activity of AsS. Due to the increased levels of L-arginine and its metabolism (polyamines and agmatine). These compounds have neuroprotective properties and have a role in the response to oxidative stress in neurological disorders [36]. AsL; Argininosuccinate lyase, AsS; Argininosuccinate synthetase, DAG; Diacylglycerol, GSK3β; Glycogen synthase kinase 3β, GTP; Guanosine triphosphate, IP3; Inositol triphosphate, mAChR-M1; M1 muscarinic acetylcholine receptor, MAPK; mitogen-activated protein kinase, PIP2; Phosphatidylinositol 4,5-bisphosphate, PKC; Protein kinase C, PLC; Phospholipase C.
PROs 5a and 7a exhibit neuroprotective effects in SH-SY5Y cells, but distinct molecular mechanisms seem to underlie their neuroprotection [45]. MDLA did not change the improvement of cell viability or decrease oxidative stress biomarkers in PRO-5a and PRO-7a-mediated neuroprotection [45] in contrast to PRO-10c [36]. PRO-7a exhibited a heightened response to increasing NO and prevented the decline in mitochondrial membrane potential against oxidative stress, unlike PRO-5a [45].
A peptide p-BTX-I was obtained from B. atrox venom and presents neuroprotective effects against dopaminergic neurotoxicity induced by 1-methyl-4-phenylpyridinium (MPP+) in neuronal PC12 cells, which reproduces Parkinson’s disease [46]. This peptide enhances cellular differentiation and vitality in cells against MPP+, suggesting that the protective effect operates through the activation of the Nerve Growth Factor (NGF)-signaling pathway [31,49]. An examination of the biotransformation of PRO-5a (also known as EKWAP) has shown that a metabolite (also known as EKW) is found in urine when PRO-5a is injected intraperitoneally. This suggests that PRO-5a is easily broken down by proteolytic enzymes naturally present in animals [18]. PRO-5a is hydrolyzed by ACE, specifically cleaving between WA amino acids [50] (Table 2). It indicates that the metabolized form of PRO-5a could possess similar neuroprotective properties to the <EVW peptide (Table 2).
PROs 5a and 7a are agonists of mAchR-M1 [29,30]. The mAchR-M1 stimulated have neuroprotective effects in the central nervous system (CNS) [52,53], and it is considered when the mechanisms that explain PROs 5a and 7a-mediated neuroprotection are discussed. Indeed, the neuroprotection induced by PRO-7a against oxidative stress in PC12 cells is dependent on mAchR-M1 activation [51].
mAChR-M1 is conventionally associated with the G-protein family to trigger the stimulation of phospholipase C (PLC) and protein kinase C (PKC), which in turn suppress the activity of glycogen synthase kinase 3 beta (GSK 3 β). This suppression leads to oxidative stress reduction [54]. Stimulating the activation of mAchR-M1 receptors enhances brain function and modifies the start or progression of Alzheimer’s disease [55]. Additionally, it has been recognized as a crucial therapeutic focus for neurodegenerative condition [56].
PRO-7a-induced neuroprotection appear to be achieved via activating the mAchR-M1, leading to a decrease in stress markers and neuronal toxicity [51]. PRO-7a also does not affect AsS activity or L-arginine availability, as reported by PRO-10c [51]. Then, it has been proposed that the neuroprotection by PRO-7a may be mediated by unique mAChR-M1 through G-protein/PLC/PKC signaling, in contrast to PRO-10c. This mechanism involves the inhibition of GSK3β, resulting in a reduction of oxidative stress and neuron damage (Fig. 3B).
Antioxidants neutralize free radicals, consequently preventing a chain reaction, and reducing the ROS production [57,58]. Antioxidant peptides have the capacity to decrease the generation of oxidative compounds by activating cellular antioxidant enzymes, such as SOD and catalase, which are accountable for sustaining the antioxidant system within cells [42]. Some PROs of B. jararaca were examined for their potential as antioxidants, but the PROs 5a, 7a, and 10c were incapable of capturing free radicals [36,45]. However, the BmT-2 peptide (FPWIIS-NH2) was identified in B. moojeni and belongs to the tryptophyllin family and showed antioxidant functions [59]. Tryptophyllins are a class of peptides found in the skin secretions of amphibians [59]. The inclusion of aromatic residues in the main structure of tryptophyllins, such as BmT-2, significantly contributes to their antioxidant effects [59,60].
The latest discoveries described in this review demonstrate that various oligopeptides are found in snake venom, each with distinct sequences and functions, have the potential to be a compelling subject of investigation. They may function as promising candidates for novel neuroprotective therapy and function as prototypes for the development of neuroprotective drugs.
Acknowledgement: The author expresses appreciation to the administrative-technical section of the Natural and Humanities Sciences Center for secretarial assistance.
Funding Statement: This work received funding from the State of São Paulo Research Foundation (FAPESP) and the Coordination for the Improvement of Higher Education Personnel (CAPES) under Finance Code 001.
Author Contributions: The authors confirm contribution to the paper as follows: study conception and design: CAS; data collection: BRS and CAS; Data curation: CAS and BRS; writing-original draft: BRS and CAS; writing review & editing: CAS. All authors reviewed the results and approved the final version of the manuscript. All authors reviewed the results and approved the final version of the manuscript.
Availability of Data and Materials: All data generated or analyzed during this study are included in this published article, and original data are available from the corresponding author upon reasonable request.
Ethics Approval: Not applicable.
Conflicts of Interest: The author declares that they have no conflicts of interest to report regarding the present study.
References
1. Mackessy SP. Venom production and secretion in reptiles. J Exp Biol. 2022;225(7):jeb227348. doi:10.1242/JEB.227348 [Google Scholar] [PubMed] [CrossRef]
2. Uko SO, Malami I, Ibrahim KG, Lawal N, Bello MB, Abubakar MB, et al. Revolutionizing snakebite care with novel antivenoms: breakthroughs and barriers. Heliyon. 2024;10(3):e25531. doi:10.1016/J.HELIYON.2024.E25531 [Google Scholar] [PubMed] [CrossRef]
3. Tan CH. Snake Venomics: fundamentals, recent updates, and a look to the next decade. Toxins. 2022;14(4):247. doi:10.3390/TOXINS14040247 [Google Scholar] [PubMed] [CrossRef]
4. Alberto-Silva C, Franzin CS, Gilio JM, Bonfim RS, Querobino SMH. Toxicological effects of bioactive peptide fractions obtained from Bothrops jararaca snake venom on the structure and function of mouse seminiferous epithelium. J Venom Anim Toxins Incl Trop Dis. 2020;26(4):e20200007. doi:10.1590/1678-9199-JVATITD-2020-0007 [Google Scholar] [PubMed] [CrossRef]
5. Alberto-Silva C, Querobino SM, Melo-Silva CA, Costa MS, Franco Oliveira LV, Zamuner SR. Local envenomation caused by a bioactive peptide fraction of Bothrops jararaca snake venom induces leukocyte influx in the lung and changes in pulmonary mechanics. Toxicon. 2022;207:52–9. doi:10.1016/j.toxicon.2022.01.001 [Google Scholar] [PubMed] [CrossRef]
6. Casewell NR, Jackson TNW, Laustsen AH, Sunagar K. Causes and consequences of snake venom variation. Trends Pharmacol Sci. 2020;41(8):570–81. doi:10.1016/j.tips.2020.05.006 [Google Scholar] [PubMed] [CrossRef]
7. Tasoulis T, Isbister GK. A current perspective on snake venom composition and constituent protein families. Arch Toxicol. 2023;97(1):133–53. doi:10.1007/s00204-022-03420-0 [Google Scholar] [PubMed] [CrossRef]
8. Oliveira AL, Viegas MF, da Silva SL, Soares AM, Ramos MJ, Fernandes PA. The chemistry of snake venom and its medicinal potential. Nat Rev Chem. 2022;6(7):451–69. doi:10.1038/s41570-022-00393-7. [Google Scholar] [CrossRef]
9. de Oliveira Almeida G, de Oliveira IS, Arantes EC, Sampaio SV. Snake venom disintegrins update: insights about new findings. J Venom Anim Toxins Incl Trop Dis. 2023;29:e20230039. doi:10.1590/1678-9199-jvatitd-2023-0039 [Google Scholar] [PubMed] [CrossRef]
10. Hayashi MAF, Camargo ACM. The Bradykinin-potentiating peptides from venom gland and brain of Bothrops jararaca contain highly site specific inhibitors of the somatic angiotensin-converting enzyme. Toxicon. 2005;45(8):1163–70. doi:10.1016/j.toxicon.2005.02.017 [Google Scholar] [PubMed] [CrossRef]
11. Vasconcelos AA, Estrada JC, David V, Wermelinger LS, Almeida FCL, Zingali RB. Structure-function relationship of the disintegrin family: sequence signature and integrin interaction. Front Mol Biosci. 2021;8:783301. doi:10.3389/FMOLB.2021.783301 [Google Scholar] [PubMed] [CrossRef]
12. Cushman DW, Ondetti MA. Inhibitors of angiotensin-converting enzyme for treatment of hypertension. Biochem Pharmacol. 1980;29(1):1871–7. doi:10.1016/0006-2952(80)90096-9 [Google Scholar] [PubMed] [CrossRef]
13. Kini RM, Koh CY. Snake venom three-finger toxins and their potential in drug development targeting cardiovascular diseases. Biochem Pharmacol. 2020;181:114105. doi:10.1016/J.BCP.2020.114105 [Google Scholar] [PubMed] [CrossRef]
14. Sciani JM, Pimenta DC. The modular nature of bradykinin-potentiating peptides isolated from snake venoms. J Venom Anim Toxins Incl Trop Dis. 2017;23:45. doi:10.1186/s40409-017-0134-7 [Google Scholar] [PubMed] [CrossRef]
15. Camargo ACM, Ianzer D, Guerreiro JR, Serrano SMT. Bradykinin-potentiating peptides: beyond captopril. Toxicon. 2012;59(4):516–23. doi:10.1016/j.toxicon.2011.07.013 [Google Scholar] [PubMed] [CrossRef]
16. Tashima AK, Zelanis A, Kitano ES, Ianzer D, Melo RL, Rioli V, et al. Peptidomics of three Bothrops snake venoms: insights into the molecular diversification of proteomes and peptidomes. Mol Cell Proteomics. 2012;11(11):1245–62. doi:10.1074/mcp.M112.019331 [Google Scholar] [PubMed] [CrossRef]
17. Ianzer D, Konno K, Marques-Porto R, Vieira Portaro FC, Stöcklin R, Martins de Camargo AC, et al. Identification of five new bradykinin potentiating peptides (BPPs) from Bothrops jararaca crude venom by using electrospray ionization tandem mass spectrometry after a two-step liquid chromatography. Peptides. 2004;25(7):1085–92. doi:10.1016/j.peptides.2004.04.006 [Google Scholar] [PubMed] [CrossRef]
18. Silva CA, Ianzer DA, Portaro FCV, Konno K, Faria M, Fernandes BL, et al. Characterization of urinary metabolites from four synthetic bradykinin potentiating peptides (BPPs) in mice. Toxicon. 2008;52(3):501–7. doi:10.1016/j.toxicon.2008.06.024 [Google Scholar] [PubMed] [CrossRef]
19. Ianzer D, Santos RAS, Etelvino GM, Xavier CH, Santos JDA, Mendes EP, et al. Do the cardiovascular effects of angiotensin-converting enzyme (ACE) I involve ACE-independent mechanisms? New insights from proline-rich peptides of Bothrops jararaca. J Pharmacol Exp Ther. 2007;322(2):795–805. doi:10.1124/jpet.107.120873 [Google Scholar] [PubMed] [CrossRef]
20. Hayashi MAF, Murbach AF, Ianzer D, Portaro FCV, Prezoto BC, Fernandes BL, et al. The C-type natriuretic peptide precursor of snake brain contains highly specific inhibitors of the angiotensin-converting enzyme. J Neurochem. 2003;85(4):969–77. doi:10.1046/j.1471-4159.2003.01743.x [Google Scholar] [PubMed] [CrossRef]
21. Ferreira SH, Bartelt DC, Greene LJ. Isolation of bradykinin-potentiating peptides from Bothrops jararaca venom. Biochemistry. 1970;9(13):2583–93. doi:10.1021/bi00815a005 [Google Scholar] [PubMed] [CrossRef]
22. Ianzer D, Xavier CH, Fraga FC, Lautner RQ, Machado LT, Mendes EP, et al. BPP-5a produces a potent and long-lasting NO-dependent antihypertensive effect. Ther Adv Cardiovasc Dis. 2011;5(6):281–95. doi:10.1177/1753944711427318 [Google Scholar] [PubMed] [CrossRef]
23. Morais KLP, Ianzer D, Miranda JRR, Melo RL, Guerreiro JR, Santos RAS, et al. Proline rich-oligopeptides: diverse mechanisms for antihypertensive action. Peptides. 2013;48:124–33. doi:10.1016/j.peptides.2013.07.016 [Google Scholar] [PubMed] [CrossRef]
24. Ferreira SH, Greene LJ, Alabaster VA, Bakhle YS, Vane JR. Activity of various fractions of bradykinin potentiating factor against Angiotensin I converting enzyme. Cah Rev The. 1970;225(5230):379–80. doi:10.1038/225379a0 [Google Scholar] [PubMed] [CrossRef]
25. Guerreiro JR, Lameu C, Oliveira EF, Klitzke CF, Melo RL, Linares E, et al. Argininosuccinate synthetase is a functional target for a snake venom anti-hypertensive peptide: role in arginine and nitric oxide production. J Biol Chem. 2009;284(30):20022–20033. doi:10.1074/jbc.M109.021089 [Google Scholar] [PubMed] [CrossRef]
26. Silva CA, Portaro FCV, Fernandes BL, Ianzer DA, Guerreiro JR, Gomes CL, et al. Tissue distribution in mice of BPP 10c, a potent proline-rich anti-hypertensive peptide of Bothrops jararaca. Toxicon. 2008;51(4):515–23. doi:10.1016/j.toxicon.2007.11.003 [Google Scholar] [PubMed] [CrossRef]
27. Cotton J, Hayashi MAF, Cuniasse P, Vazeux G, Ianzer D, de Camargo ACM, et al. Selective inhibition of the C-domain of angiotensin I converting enzyme by bradykinin potentiating peptides. Biochemistry. 2002;41(19):6065–71. doi:10.1021/bi012121x [Google Scholar] [PubMed] [CrossRef]
28. Rioli V, Prezoto BC, Konno K, Melo RL, Klitzke CF, Ferro ES, et al. A novel bradykinin potentiating peptide isolated from Bothrops jararacussu venom using catallytically inactive oligopeptidase EP24.15. Febs J. 2008;275(10):2442–54. doi:10.1111/j.1742-4658.2008.06389.x [Google Scholar] [PubMed] [CrossRef]
29. Morais KLP, Hayashi MAF, Bruni FM, Lopes-Ferreira M, Camargo ACM, Ulrich H, et al. Bj-PRO-5a, a natural angiotensin-converting enzyme inhibitor, promotes vasodilatation mediated by both bradykinin B2 and M1 muscarinic acetylcholine receptors. Biochem Pharmacol. 2011;81(6):736–42. doi:10.1016/j.bcp.2010.12.016 [Google Scholar] [PubMed] [CrossRef]
30. Negraes PD, Lameu C, Hayashi MAF, Melo RL, Camargo ACM, Ulrich H. The snake venom peptide Bj-PRO-7a is a M1 muscarinic acetylcholine receptor agonist. Cytometry A. 2011;79(1):77–83. doi:10.1002/cyto.a.20963 [Google Scholar] [PubMed] [CrossRef]
31. Bernardes CP, Santos NAG, Costa TR, Sisti F, Amaral L, Menaldo DL, et al. A synthetic snake-venom-based tripeptide protects PC12 cells from the neurotoxicity of acrolein by improving axonal plasticity and bioenergetics. Neurotox Res. 2020;37(1):227–37. doi:10.1007/s12640-019-00111-0 [Google Scholar] [PubMed] [CrossRef]
32. Abdullah NAH, Sainik NQAV, Esa E, Muhamad Hendri NA, Ahmad Rusmili MR, Hodgson WC, et al. Neuroprotective effect of phospholipase A2 from Malaysian Naja sumatrana venom against H2O2-induced cell damage and apoptosis. Front Pharmacol. 2022;13:935418. doi:10.3389/fphar.2022.935418 [Google Scholar] [PubMed] [CrossRef]
33. Martins N, Ferreira D, Carvalho Rodrigues M, Cintra A, Santos N, Sampaio S, et al. Low-molecular-mass peptides from the venom of the Amazonian viper Bothrops atrox protect against brain mitochondrial swelling in rat: potential for neuroprotection. Toxicon. 2010;56(1):86–92. doi:10.1016/j.toxicon.2010.03.014 [Google Scholar] [PubMed] [CrossRef]
34. Perez-Pinzon MA, Anne Stetler R, Fiskum G. Novel mitochondrial targets for neuroprotection. J Cereb Blood Flow Metab. 2012;32(7):1362–76. doi:10.1038/jcbfm.2012.32 [Google Scholar] [PubMed] [CrossRef]
35. Querobino SM, Carrettiero DC, Costa MS, Alberto-Silva C. Neuroprotective property of low molecular weight fraction from B. Jararaca snake venom in H2O2-induced cytotoxicity in cultured hippocampal cells. Toxicon. 2017;129:134–43. doi:10.1016/j.toxicon.2017.02.015 [Google Scholar] [PubMed] [CrossRef]
36. Querobino SM, Ribeiro CAJ, Alberto-Silva C. Bradykinin-potentiating PEPTIDE-10C, an argininosuccinate synthetase activator, protects against H2O2-induced oxidative stress in SH-SY5Y neuroblastoma cells. Peptides. 2018;103:90–7. doi:10.1016/j.peptides.2018.03.017 [Google Scholar] [PubMed] [CrossRef]
37. Pantaleão HQ, Araujo da Silva JC, Rufino da Silva B, Echeverry MB, Alberto-Silva C. Peptide fraction from B. jararaca snake venom protects against oxidative stress-induced changes in neuronal PC12 cell but not in astrocyte-like C6 cell. Toxicon. 2023;231:107178. doi:10.1016/j.toxicon.2023.107178 [Google Scholar] [PubMed] [CrossRef]
38. Du T, Han J. Arginine metabolism and its potential in treatment of colorectal cancer. Front Cell Dev Biol. 2021;9:658861. doi:10.3389/fcell.2021.658861 [Google Scholar] [PubMed] [CrossRef]
39. Chen CL, Hsu SC, Ann DK, Yen Y, Kung HJ. Arginine signaling and cancer metabolism. Cancers. 2021;13(14):3541. doi:10.3390/cancers13143541 [Google Scholar] [PubMed] [CrossRef]
40. Cervelli M, Averna M, Vergani L, Pedrazzi M, Amato S, Fiorucci C, et al. The involvement of polyamines catabolism in the crosstalk between neurons and astrocytes in neurodegeneration. Biomedicines. 2022;10(7):1756. doi:10.3390/biomedicines10071756 [Google Scholar] [PubMed] [CrossRef]
41. Szeliga M, Albrecht J. Roles of nitric oxide and polyamines in brain tumor growth. Adv Med Sci. 2021;66(1):199–205. doi:10.1016/j.advms.2021.02.006 [Google Scholar] [PubMed] [CrossRef]
42. Perlikowska R. Whether short peptides are good candidates for future neuroprotective therapeutics? Peptides. 2021;140:170528. doi:10.1016/J.PEPTIDES.2021.170528 [Google Scholar] [PubMed] [CrossRef]
43. Robinson SD, Vetter I. Pharmacology and therapeutic potential of venom peptides. Biochem Pharmacol. 2020;181:114207. doi:10.1016/J.BCP.2020.114207 [Google Scholar] [PubMed] [CrossRef]
44. Munawar A, Ali SA, Akrem A, Betzel C. Snake Venom Peptides: tools of Biodiscovery. Toxins. 2018;10(11):474. doi:10.3390/TOXINS10110474 [Google Scholar] [PubMed] [CrossRef]
45. Querobino SM, Costa MS, Alberto-Silva C. Protective effects of distinct proline-rich oligopeptides from B. jararaca snake venom against oxidative stress-induced neurotoxicity. Toxicon. 2019;167:29–37. doi:10.1016/j.toxicon.2019.06.012 [Google Scholar] [PubMed] [CrossRef]
46. Martins N, Santos N, Sartim M, Cintra A, Sampaio S, Santos A. A tripeptide isolated from Bothrops atrox venom has neuroprotective and neurotrophic effects on a cellular model of Parkinson’s disease. Chem Biol Interact. 2015;235:10–6. doi:10.1016/j.cbi.2015.04.004 [Google Scholar] [PubMed] [CrossRef]
47. Saha P, Panda S, Holkar A, Vashishth R, Rana SS, Arumugam M, et al. Neuroprotection by agmatine: possible involvement of the gut microbiome? Ageing Res Rev. 2023;91:102056. doi:10.1016/J.ARR.2023.102056 [Google Scholar] [PubMed] [CrossRef]
48. Peterson CD, Kitto KF, Verma H, Pflepsen K, Delpire E, Wilcox GL, et al. Agmatine requires GluN2B-containing NMDA receptors to inhibit the development of neuropathic pain. Mol Pain. 2021;17:17448069211029171. doi:10.1177/17448069211029171 [Google Scholar] [PubMed] [CrossRef]
49. Bernardes CP, Santos NAG, Sisti FM, Ferreira RS, Santos-Filho NA, Cintra ACO, et al. A synthetic snake-venom-based tripeptide (Glu-Val-Trp) protects PC12 cells from MPP+ toxicity by activating the NGF-signaling pathway. Peptides. 2018;104:24–34. doi:10.1016/j.peptides.2018.04.012 [Google Scholar] [PubMed] [CrossRef]
50. Cheung HS, Cushman DW. Inhibition of homogeneous angiotensin-converting enzyme of rabbit lung by synthetic venom peptides of Bothrops jararaca. Biochim Biophys Acta. 1973;293(2):451–63. doi:10.1016/0005-2744(73)90352-5 [Google Scholar] [PubMed] [CrossRef]
51. Alberto-Silva C, Pantaleão HQ, Silva BR, Silva JCA, Echeverry MB. Activation of M1 muscarinic acetylcholine receptors by proline-rich oligopeptide 7a (<EDGPIPP) from Bothrops jararaca snake venom rescues oxidative stress-induced neurotoxicity in PC12 cells. J Venom Anim Toxins incl Trop Dis. 2024;20:1–13. doi:10.1590/1678-9199-JVATITD-2023-0043 [Google Scholar] [PubMed] [CrossRef]
52. Tanaka H, Negoro K, Koike T, Tsukamoto I, Yokoyama K, Maeda J, et al. Discovery and structure-activity relationships study of positive allosteric modulators of the M3 muscarinic acetylcholine receptor. Bioorg Med Chem. 2020;28(13):115531. doi:10.1016/J.BMC.2020.115531 [Google Scholar] [PubMed] [CrossRef]
53. Yu P, Dong WP, Tang YB, Chen HZ, Cui YY, Bian XL. Huperzine A lowers intraocular pressure via the M3 mAChR and provides retinal neuroprotection via the M1 mAChR: a promising agent for the treatment of glaucoma. Ann Transl Med. 2021;9(4):332–2. doi:10.21037/atm-20-8093 [Google Scholar] [PubMed] [CrossRef]
54. Mao LM, Young L, Chu XP, Wang JQ. Regulation of Src family kinases by muscarinic acetylcholine receptors in heterologous cells and neurons. Front Mol Neurosci. 2024;16:1340725. doi:10.3389/FNMOL.2023.1340725 [Google Scholar] [PubMed] [CrossRef]
55. Fisher A. Cholinergic modulation of amyloid precursor protein processing with emphasis on M1 muscarinic receptor: perspectives and challenges in treatment of Alzheimer’s disease. J Neurochem. 2012;120 Suppl, 1:22–33. doi:10.1111/j.1471-4159.2011.07507.x [Google Scholar] [PubMed] [CrossRef]
56. Dean B. Muscarinic M1 and M4 receptor agonists for schizophrenia: promising candidates for the therapeutic arsenal. Expert Opin Investig Drugs. 2023;32(12):1113–21. doi:10.1080/13543784.2023.2288074 [Google Scholar] [PubMed] [CrossRef]
57. Lü JM, Lin PH, Yao Q, Chen C. Chemical and molecular mechanisms of antioxidants: experimental approaches and model systems. J Cell Mol Med. 2010;14(4):840–60. doi:10.1111/j.1582-4934.2009.00897.x [Google Scholar] [PubMed] [CrossRef]
58. Stoia M, Oancea S. Low-molecular-weight synthetic antioxidants: classification, pharmacological profile, effectiveness and trends. Antioxidants. 2022;11(4):638. doi:10.3390/ANTIOX11040638 [Google Scholar] [PubMed] [CrossRef]
59. Dematei A, Costa SR, Moreira DC, Barbosa EA, Friaça Albuquerque LF, Vasconcelos AG et al. Antioxidant and neuroprotective effects of the first tryptophyllin found in snake venom (Bothrops moojeni). J Nat Prod. 2022;11(4):638. doi:10.1021/ACS.JNATPROD.2C00304 [Google Scholar] [PubMed] [CrossRef]
60. Plácido A, do Pais do Amaral C, Teixeira C, Nogueira A, Brango-Vanegas J, Alves Barbosa E, et al. Neuroprotective effects on microglia and insights into the structure-activity relationship of an antioxidant peptide isolated from Pelophylax perezi. J Cell Mol Med. 2022;26(10):2793–2807. doi:10.1111/jcmm.17292 [Google Scholar] [PubMed] [CrossRef]
Cite This Article
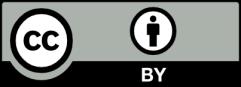
This work is licensed under a Creative Commons Attribution 4.0 International License , which permits unrestricted use, distribution, and reproduction in any medium, provided the original work is properly cited.