Open Access
ARTICLE
Quercetin regulates depression-like behavior in CUMS rat models via TLR4/NF-κB signaling
1 College of Basic Medicine, Hebei University of Chinese Medicine, Shijiazhuang, 050200, China
2 Hebei Key Laboratory of Integrated Chinese and Western Medicine for Lung Disease Research, Shijiazhuang, 050091, China
* Corresponding Author: SHAOXIAN WANG. Email:
BIOCELL 2024, 48(5), 731-744. https://doi.org/10.32604/biocell.2024.048820
Received 19 December 2023; Accepted 07 March 2024; Issue published 06 May 2024
Abstract
Background: Depression is becoming increasingly prevalent around the world, imposing a substantial burden on individuals, families, as well as society. Quercetin is known to be highly effective in treating depression. However, additional research is needed to dissect the mechanisms of its anti-depressive effects. Methods: For this study, Sprague-Dawley (SD) rats were randomized into the control, model, quercetin, or fluoxetine group. The latter three groups were exposed to chronic unpredictable mild stress (CUMS) for 42 d. The first two groups received saline solution daily via oral gavage. Meanwhile, the quercetin group was orally administered a quercetin suspension (52.08 mg/kg) every day, while the fluoxetine group was orally administered a fluoxetine solution (2.08 mg/kg). Here, fluoxetine served as the positive control drug to compare the therapeutic effects of quercetin. The experimental period was 6 weeks. Depressive behaviors in rats were assessed through various physiological and behavioral measures. Additionally, pathological changes in hippocampal tissues were examined using Nissl staining. Serum cytokines were detected using an enzyme-linked immunosorbent assay (ELISA), and immunohistochemistry was employed to quantify the levels and integral optical density (IOD) values of ionized calcium binding adaptor molecule-1 (Iba-1) expression in the brain. Real-time fluorescence quantitative PCR (RT-qPCR) was utilized to evaluate the mRNA levels of inflammatory indicators as well as toll-like receptor 4 (TLR4), and nuclear factor-κappa B P65 (NF-κB P65) in hippocampus. Western blot (WB) technique was employed to observe the protein levels of TLR4, NF-κB P65, and phospho-NF-κB P65 (p-NF-κB P65). Results: After 42 d of exposure to CUMS, rats exhibited a slow increase in body weight, a reduction in food intake, an abnormal preference for sugar water, and aberrant open-field behaviors. Pathological analysis revealed the disintegration, rupture, interruption, and disorganization of hippocampal neuronal cells after CUMS exposure, along with a decrease in Nissl bodies in the CA1 region. This was accompanied by the elevated expression of interleukin-1β (IL-1β), tumor necrosis factor-α (TNF-α), and interleukin-6 (IL-6) in the serum and the upregulation of IL-1β, IL-6, and TNF-α mRNA expression in the hippocampus. Increases in Iba-1-positive cells and the IOD values of Iba-1 were detected in hippocampal microglia. Furthermore, TLR4 and NF-κB P65 mRNA and protein levels were upregulated in hippocampal tissues. Quercetin, an antidepressant, could alleviate depression-like symptoms in rats and downregulate inflammatory factors associated with the TLR4/NF-κB signaling pathway in hippocampal microglia, and its therapeutic effect was comparable to fluoxetine. Conclusion: In rat models of CUMS, quercetin may act as an antidepressant by inhibiting inflammation in hippocampal microglia via TLR4/NF-κB signaling pathway. These results offer experimental and theoretical support for applying quercetin in the clinical management of depression.Keywords
Detailed investigations by World Health Organization (WHO) have revealed that depression is a global health problem. According to their estimates, nearly 1 billion people globally have psychological disorders, and death by suicide accounts for more than one in every 100 deaths. Epidemiological studies have revealed that cases of depression and anxiety spiked by more than 25% in the year following the start of the COVID-19 pandemic [1]. By the year 2030, depression is expected to emerge as the foremost health disorder globally [2].
Various synthetic second-generation antidepressants, such as selective 5-hydroxytryptamine (5-HT) reuptake inhibitors, selective norepinephrine (NE) reuptake inhibitors, and other second-generation antidepressants, are currently employed in the clinical treatment of depression. However, these drugs typically exhibit heightened toxicity, and the risk of drug resistance is often high. As a consequence, the therapeutic effectiveness and prognostic outcomes of these agents have so far been limited in individuals with depression [3].
Quercetin is a natural flavonoid that is found in many commonly consumed foods, and it is a key bioactive component of many herbs. This compound is highly safe and has many potential health benefits. Thus, it is often prescribed as a health supplement and used in the production of nutraceuticals. A series of retrospective studies and reviews have demonstrated the efficacy and safety of quercetin [4,5]. Furthermore, clinical observations and experimental studies have also suggested that quercetin can effectively relieve depression and improve neurological health [6,7]. Nevertheless, at present, the relevant research in this area remains insufficient.
Accumulating evidence from several studies has indicated that the immune imbalance in the brain is a key driver of the pathogenesis of depression. Hippocampal microglia are believed to be the initial line of defense against this imbalance. These microglia perform macrophage-like functions in the brain, participating in neuroimmune regulation through the modulation of organismal immune function [8]. Ionized calcium binding adaptor molecule-1 (Iba-1) serves as a marker of microglial activation, and its expression levels in combination with microglial morphology can indicate the degree of microglial activation [9–11]. However, whether microglia are over-activated under a state of depression warrants further investigation. Additionally, the mechanisms underlying the antidepressant effects of quercetin also need to be explored.
To this end, we prepared an animal model of depression using a chronic unpredictable mild stress (CUMS) paradigm for 42 consecutive days. This study examined whether quercetin can effectively treat depression in rats by dynamically analyzing various physiological and behavioral parameters in rats treated with quercetin. Additionally, it explored the mechanism of microglial activation in the hippocampus by focusing on a key inflammatory pathway of toll-like receptor 4 (TLR4)/nuclear factor-κappa B (NF-κB). The overarching goal of the study was to provide an experimental and theoretical foundation for using quercetin to treat depression and improve the clinical management of this prevalent disorder.
The animal model of depression was established in male Sprague-Dawley (SD) rats (Beijing Vital River Laboratory Animal Technology Co., Ltd., China) (6 weeks old, 180 to 220 g in weight). The animals were housed in a standard animal room under a 12-h light/dark cycle, with humidity levels of 30% to 50% and a temperature of 23°C to 25°C. Animals were housed in clean living conditions and received water and standard feed as needed. All procedures used for animal experiments and sample handling strictly adhered to the relevant regulations regarding animal care and welfare. Additionally, we ensured that animal usage and suffering were minimized. The Experimental Animal Ethics Committee of Hebei University of Chinese Medicine (Shijiazhuang, China, Approval No. DWLL202203145) approved all animal experiments and procedures.
The depression model was established using a CUMS paradigm, as described previously [12,13]. CUMS stimulation consisted of a range of stress-inducing methods, which included: 3-h restraint, 3-min tail clipping, 5-min swimming in hot water at 45°C, 5-min swimming in cold water at 4°C, 12-h cage tilting exposure, 24-h reversal of day and night, 24-h of placement on wet bedding, 24-h fasting, 24-h water deprivation, 3-h noise exposure, 30-min of horizontal oscillations, and 5-min foot shocks (0.85 mA for 15 s, repeated after a 15-s interval). Two of these stimulation methods were randomly administered every day, without repeating the same stimulus within a period of 48 h. CUMS was applied for 6 weeks. The specific stimulations provided daily are shown in Table 1.
Animal groups and treatment protocols
Following a period of acclimation that lasted 1 week, 40 rats underwent random assignment into four groups (n = 10 per group): the control, model, quercetin, and fluoxetine groups. All animals from the latter three groups underwent CUMS stimulation. The control and model groups received saline daily via oral gavage. Meanwhile, the quercetin group received quercetin daily (Sigma-Aldrich, Inc., 117-39-5, St. Louis, MO, USA) via suspension gavage. The standard for quercetin administration in Chinese adults is 500 mg/d orally. Hence, based on a weight conversion coefficient of 6.25, the quercetin group rats received a dose of 52.08 mg/kg quercetin daily. Meanwhile, the fluoxetine group received a daily dose of fluoxetine (Lilly Suzhou Pharmaceutical Co., Ltd., J20160029, Jiangsu, China) solution. The standard for fluoxetine administration in Chinese adults is 20 mg/d. Accordingly, based on a weight conversion coefficient of 6.25, the fluoxetine dose for rats was calculated to be 2.08 mg/kg daily. The treatment period was 6 weeks. Here, fluoxetine served as the positive control. Fig. 1 shows the study’s workflow.
Figure 1: Flow chart showing the experimental design.
Following the intraperitoneal administration of 3% short-acting pentobarbital sodium (50 mg/kg), blood samples were obtained from the femoral artery of the rats. The samples were then placed at room temperature for an hour. Serum samples were subjected to centrifugation at a low temperature and refrigerated at −80°C in order to facilitate the subsequent enzyme-linked immunosorbent assay (ELISA). Whole brains of four decapitated rats from each experimental group were preserved in a 10% neutral formaldehyde solution prepared using 9 parts phosphate-buffered saline (PBS) and 1 part formaldehyde solution (Tianjin Best Chemical Co., Ltd., 20230320, Tianjin, China). Subsequently, these brain samples were used for Nissl staining and immunohistochemical analyses. Additionally, hippocampal tissues were extracted from the remaining brains and frozen in liquid nitrogen before storage at −80°C for subsequent mRNA or protein detection.
The body weights of the rats were first examined on day 0 (the day before the formal experiment). Subsequently, body weight was recorded on days 7, 14, 21, 28, 35, and 42. Based on these data, the trends of changes in body weight were analyzed.
The 10 rats from each group were housed across four cages, with 2~3 rats in each cage. One day before the food intake measurement, the quantity of food in each cage was measured and the quantity remaining after 24 h was also recorded. Accordingly, the average food intake was calculated as follows: Average food intake = (food given – food remaining)/(number of rats).
These measurements were first obtained on day 0 (the day before the formal experiment), followed by days 7, 14, 21, 28, 35, and 42. Based on these data, the trends of changes in food intake were analyzed.
Changes in sugar water preference test
The sugar water preference test was performed as described previously [14]. Prior to conducting this experiment, on the first day, two 200 mL bottles of sucrose water were added inside each cage. On the second day, the rats were randomly presented with bottles containing 200 mL of pure water and sucrose water each and the bottles were exchanged after a 12-h duration. Subsequently, on the third day, following a period of water fasting, the sugar water preference rate was examined. In this experiment, the rats received 200 mL of pure water and 200 mL of 1% sucrose water. The amount of pure water and sucrose water remaining at the end of the 1-h period was subsequently measured. The sugar water preference rate was assessed on both day 0 and day 42, as follows: Sugar water preference rate = (The amount of sucrose water consumed)/(The amount of pure water consumed + The amount of sucrose water consumed) × 100%.
Behavioral changes in the open field test (OFT)
For the OFT [15,16], rats were lifted by lightly grasping their tails and were then placed in an open field box in a quiet environment. Following a 30-s adaptation period, the motion of the rats in the open field was tracked. Specifically, the number of motion squares and standing uprights, as well as the residence time and movement distance in the central region, were synchronously recorded over a 5-min period using an animal movement trajectory tracking system (Noldus Information Technology BV, EthoVision XT, Wageningen, Netherlands). After the test, the rat feces were picked up, and the open field box was wiped with pure water, the next rat was placed in the box, and the same procedure was repeated. Behavioral indicators measured by the OFT were examined on day 0 and day 42 of the experimental period.
In this experiment, fixed whole rat brains were dehydrated, paraffin-embedded, sectioned, and cleared using xylene. The sections were hydrated in an ethanol gradient and stained with Nissl staining solution (Beijing Solarbio Science & Technology Co., Ltd., G1436, Beijing, China). The sections were washed in water, differentiated using a weak acid, and dehydrated using anhydrous ethanol. After further clearing with xylene, they were sealed in neutral gum and examined microscopically (Olympus Corporation, BX53, Tokyo, Japan).
Serum levels of inflammatory factors
We acquired the ELISA kits for interleukin-1β (IL-1β) and tumor necrosis factor-α (TNF-α) from R&D Inc. (RLB00 and RTA00, Minnesota, USA), as well as for interleukin-6 (IL-6) ELISA kit (Neobioscience Technology, Inc., ERC003, Shenzhen, China). The serum levels of the inflammatory factors were assessed using these kits. The assays were performed in strict adherence to the instructions provided in the respective kits.
Iba-1 expression in hippocampal microglia
Fixed hippocampal tissues were sectioned, dewaxed, and then rehydrated using a gradient of ethanol. Antigen repair was performed by soaking in a citrate buffer solution at high temperatures, and the tissue was treated with 3% H2O2 to eliminate endogenous peroxidase activity. Sections were then washed using PBS and blocked in sheep serum (Vector Group Ltd., PK-4001/2, San Francisco, USA) to prevent non-specific reactions. Finally, the sections were incubated with an anti-ionized calcium binding adaptor molecule-1 (Iba-1) antibody (1:400, Cell Signaling Technology Co., Ltd., #17198, Boston, USA) overnight at 4°C in a wet box. The immunoreaction was conducted using an immunohistochemistry kit (Vector Group Ltd., PK-4001/2, San Francisco, USA), followed by incubation at 37°C. The sections were immunostained using DAB (Beijing Zhongshan Jinqiao Biotechnology Co., Ltd., ZLI-9017, Beijing, China) chromatography, and then counterstained with hematoxylin. The stained sections were differentiated using a mixture of hydrochloric acid in alcohol, subjected to gradient dehydration, and sealed in neutral gum before drying. Subsequently, they were observed and imaged using a microscope (Olympus Corporation, BX53, Tokyo, Japan). The expression of Iba-1 and their integral optical density (IOD) values were evaluated using Image-Pro Plus 6.0 (Media Cybernetics, Inc., Maryland, USA).
Changes in hippocampal mRNA expression
We extracted total RNA from each sample (about 50 μg) using a total RNA extraction kit and synthesized cDNA using a reverse transcription kit (Shanghai Promega Biological Products Co., Ltd., LS1040 and A5001, Shanghai, China). Further, 2 μL of cDNA was added to a 20 μL reaction mixture as a template for PCR amplification (Shanghai Promega Biological Products Co., Ltd., A6001, Shanghai, China) on a real-time fluorescent quantitative PCR system (Bio-Rad Corporation, CFX96, California, USA). The methods were: pre-denaturation (95°C, 10 min), denaturation (95°C, 15 s) and annealing (60°C, 1 min) with 45 cycles. During the cyclic annealing stage, signals were detected to obtain Ct values for the target and reference genes. With Glyceraldehyde 3-phosphate dehydrogenase (GAPDH) as the internal reference, the relative quantitative Ct values (RQs) were determined based on the formula RQ = 2−ΔΔCt, and these values were statistically analyzed. All experimental primers are noted in Table 2.
Changes in hippocampal protein expression
First, lysis buffer containing protease inhibitor (Beyotime Biotechnology Co., Ltd., P1045, Shanghai, China), phenylmethyl sulfonyl fluoride (PMSF) (SEVEN Biotechnology Co., Ltd., SW106-01, Beijing, China), and RIPA (Servicebio Biotechnology Co., Ltd., CR2206025, Wuhan, China) was prepared. About 100 mg was prepared for each sample, and lysis buffer was added to the sample at a 1:4 ratio between the sample and the lysate. Hippocampal tissue was homogenized at a low temperature using a manual homogenizer, and the supernatant was extracted after centrifugation (Sigma-Aldrich Chemie GmbH, 3K15, Taufkirchen, Germany) at 4°C and a speed of 8000 rpm for 10 min. Based on kit instructions (Abbkine Scientific Co., Ltd., KTD3010-CN, Wuhan, China), the total protein was quantified by utilizing the rapid BCA method. After protein denaturation at a high temperature, 100 ug of each sample was subjected to electrophoresis, and separated proteins underwent NC membrane transfer (Pall Corporation, #63199956, New York, USA). After sealing at room temperature for 2 h, the membranes with 5% non-fat milk powder mixed in Tris Buffered Saline with Tween 20 (TBST) (Beijing Solarbio Science & Technology Co., Ltd., T1086, Beijing, China) were washed and incubated with a mouse monoclonal anti-GAPDH antibody (1:20,000, Proteintech Group, Inc., 6004-1-1g, Chicago, USA), mouse monoclonal anti-nuclear factor-κappa B P65 (NF-κB P65) antibody (1:1,000, Immunoway Biotechnology Co., Ltd., YM3111, Texas, USA), rabbit monoclonal anti-phospho-NF-κB P65 (p-NF-κB P65) antibody (1:1,000, Cell Signaling Technology Co., Ltd., #3033, Danvers, MA, USA), and rabbit polyclonal anti-TLR4 antibody (1:500, Affinity Biosciences Co., Ltd., AF7017, Jiangsu, China) after washing. The membranes were incubated with the antibodies at 4°C overnight. They were then washed and incubated with the following secondary antibodies on a shaker at room temperature and protection from light for a duration of 1 h: IRDye 800CW Goat anti-Mouse (or anti-Rabbit) IgG secondary antibody (1:10,000, Li-cor Biosciences Co., Ltd., 926-32210 and 926-32211, Nebraska, USA). Subsequently, the IOD of the bands was measured using a two-color infrared laser imaging system (Li-cor Biosciences Co., Ltd., ODYSSEY CLx, Nebraska, USA) after washing the membranes. TLR4, NF-κB P65, and p-NF-κB P65 expression was calculated based on the ratio between the IOD values of these target proteins and the IOD value of the GAPDH protein.
Data were shown as mean ± standard deviation (ˉx ± s). The data were analyzed using SPSS 23.0 and GraphPad Prism 5. Following statistical analysis, the data were found to exhibit a normal distribution. The data pertaining to body weight, food intake, sugar water preference test, and OFT were subjected to analysis through univariate ANOVA utilizing the repeated measurement method within the framework of the general linear model, as well as through multivariate ANOVA employing the Tukey method to compare between-group differences at various time points. For other data, the Tukey test was employed to conduct pairwise comparisons between groups. The threshold of statistical significance was p < 0.05.
Prior to CUMS exposure, the rats in each experimental group exhibited comparable body weights (p > 0.05). However, subsequent to CUMS stimulation, the model rats showed a slow increase in weight, with this parameter differing significantly from that in the control group starting on day 14 (p < 0.01). Furthermore, the rate of weight gain varied between the model group and the quercetin and fluoxetine treatment groups, and statistically significant disparities were observed from day 21 onwards (p < 0.01) (Fig. 2).
Figure 2: Variations in body weight in the four groups (n = 10). *p < 0.05, **p < 0.01 vs. the control group; ##p < 0.01 vs. the model group.
Before exposure to CUMS, rats from the different groups consumed similar amounts of food among groups (p > 0.05). Nevertheless, the model group consumed less food than the control group following CUMS exposure (p < 0.01). Additionally, the amount of food intake was greater in the quercetin and fluoxetine groups than in the model group on days 7, 21, 35, and 42 (p < 0.05 or p < 0.01) (Fig. 3).
Figure 3: Variations in food intake in the four groups (n = 4). *p < 0.05, **p < 0.01 vs. the control group; #p < 0.05, ##p < 0.01 vs. the model group.
Changes in sugar water preference rate
The sugar water preference rate was found to be comparable among the different groups before exposure to CUMS (p > 0.05). However, following CUMS stimulation, the model group exhibited a lower sugar water preference rate than the control group (p < 0.01). Additionally, the quercetin and fluoxetine groups demonstrated a significantly higher sugar water preference rate than the model group (p < 0.01). Hence, the administration of quercetin appeared to alleviate anhedonia in rat models of CUMS-induced depression (Fig. 4).
Figure 4: Changes in sugar water preference rates in the control, model, quercetin, and fluoxetine groups before and after CUMS stimulation (n = 10). **p < 0.01 vs. the control group; ##p < 0.01 vs. the model group.
Changes in behavioral indicators of the OFT
A comparison of behavioral indicators revealed that the number of motion squares, the number of standing uprights, and the residence time and movement distance in the central part of the open field were comparable among the groups before CUMS stimulation (p > 0.05). However, model group rats had fewer motion squares, fewer standing uprights, shorter residence times, and shorter movement distances in the central area after exposure to CUMS than control rats (p < 0.01). Furthermore, the quercetin and fluoxetine groups exhibited a greater number of motion squares and standing uprights, and longer residence times and higher distances traveled in the central region when compared with the model group (p < 0.05 or p < 0.01). Based on the data, quercetin appeared to increase autonomic activity in rat models of CUMS-induced depression (Figs. 5A–5E).
Figure 5: Results of the OFT in the four groups on days 0 and 42. (A) Open field track maps for all groups. (B) Results showing the total number of motion squares (n = 10). (C) Results showing the number of standing uprights (n = 10). (D) Results showing the residence time in the central area (n = 10). (E) Results showing the distance traveled in the center (n = 10). *p < 0.05, **p < 0.01 vs. the control group; #p < 0.05, ##p < 0.01 vs. the model group.
Neurons from control rats appeared to be well-organized and tightly packed. These neurons also showed abundant Nissl bodies with distinct staining, indicating that they possessed robust neuronal function. However, neurons in the model group appeared to be sparsely arranged and disordered. Some of these neurons appeared ruptured, and the Nissl bodies were fewer in number and showed lighter staining. This indicated that the function of these neurons has been disrupted. Notably, the hippocampal neurons of rats treated with quercetin and fluoxetine showed a neat and tight arrangement, abundant Nissl bodies, and stronger staining than those of untreated model rats. This suggested that drug treatment attenuated neuronal dysfunction and promoted the functional recovery of hippocampal neurons (Fig. 6).
Figure 6: Nissl staining of the hippocampal CA1 region (black box) in the control, model, quercetin, and fluoxetine groups. The respective scale bars and magnifications of the images shown in the three rows of the figure are as follows: row 1: 200 μm and 40×; row 2: 50 μm and 200×; row 3: 20 μm and 400×.
Serum levels of inflammatory factors
Serum levels of TNF-α, IL-1β, and IL-6 were elevated in the model group when compared with the levels in the control group (p < 0.01). Conversely, the quercetin and fluoxetine groups demonstrated attenuated serum levels of these factors when compared with the model group (p < 0.05 or p < 0.01). Hence, quercetin appeared to attenuate inflammation in rat models of CUMS-induced depression (Figs. 7A–7C).
Figure 7: Serum levels of inflammatory factors in the four groups. (A) TNF-α (n = 10). (B) IL-1β (n = 10). (C) IL-6 (n = 10). *p < 0.05, **p < 0.01 vs. the control group; #p < 0.05, ##p < 0.01 vs. the model group.
Expression of Iba-1 in hippocampal microglia
The hippocampal microglia in the control group showed typical characteristics, including a normal cell body, and elongated and slender processes. These cells were in a resting state, characterized by the presence of prominent branching. However, microglia with enlarged cell bodies, shortened processes, and reduced branching were observed in the model group. This was indicative of a pronounced amoeboid activation state. Additionally, the number of Iba-1 positive cells and the IOD values of Iba-1 were much higher in the model group than in the control group (p < 0.01). In comparison to the hippocampal microglia in the model group, the hippocampal microglia in the quercetin and fluoxetine groups tended to have a more normal morphology, and this was accompanied by an obvious decrease in the number of Iba-1 positive cells and Iba-1 IOD values (p < 0.05 or p < 0.01). Hence, quercetin appeared to reduce microglial inflammation in rat models of CUMS-induced depression (Figs. 8A–8C).
Figure 8: (A) Immunohistochemical staining for Iba-1 in the hippocampal CA1 region (black box) in the control, model, quercetin, and fluoxetine groups. (B) Results showing the number of Iba-1 positive cells in the CA1 region (black box) in all groups (n = 6). (C) Results showing the Iba-1 IOD values in the CA1 region (black box) in all groups (n = 6). **p < 0.01 vs. the control group; #p < 0.05, ##p < 0.01 vs. the model group. The respective scale bars and magnifications of the images shown in the three rows of the figure are as follows: row 1: 200 μm and 40×; row 2: 50 μm and 200×; row 3: 20 μm and 400×.
Changes in hippocampal mRNA expression
The hippocampal mRNA levels of TNF-α, IL-1β, IL-6, TLR4, and NF-κB P65 were upregulated in the model group (p < 0.01). However, quercetin and fluoxetine attenuated the hippocampal mRNA levels of these factors (p < 0.05 or p < 0.01 vs. model group). Hence, quercetin could prevent inflammation in the hippocampus in rat models of CUMS-induced depression (Figs. 9A–9E).
Figure 9: mRNA expression of various inflammatory and signaling factors in the hippocampal tissues of the control, model, quercetin, and fluoxetine groups. (A) Results showing hippocampal TNF-α mRNA levels (n = 6). (B) Results showing hippocampal IL-1β mRNA levels (n = 6). (C) Results showing hippocampal IL-6 mRNA levels (n = 6). (D) Results showing hippocampal TLR4 mRNA levels (n = 6). (E) Results showing hippocampal NF-κB P65 mRNA levels (n = 6). **p < 0.01 vs. the control group; #p < 0.05, ##p < 0.01 vs. the model group.
Changes in hippocampal protein expression
The model group exhibited augmented protein levels of TLR4 and p-NF-κB P65/NF-κB P65 within the hippocampal region (p < 0.05 or p < 0.01 vs. control rats). Conversely, rats treated with quercetin and fluoxetine demonstrated attenuated levels of these proteins (p < 0.05 or p < 0.01 vs. model rats). Hence, quercetin appeared to inhibit TLR4/NF-κB signaling in CUMS-induced rat models of depression (Figs. 10A–10E).
Figure 10: Hippocampal expression of proteins involved in TLR4/NF-κB signaling in the control, model, quercetin, and fluoxetine groups. (A) Protein band showing TLR4 expression in the hippocampus. (B) Protein band showing NF-κB P65 expression in the hippocampus. (C) Protein band showing p-NF-κB P65 expression in the hippocampus. (D) Quantification of hippocampal TLR4 protein expression (n = 3). (E) Quantification of hippocampal p-NF-κB P65/NF-κB P65 protein expression (n = 3). *p < 0.05, **p < 0.01 vs. the control group; #p < 0.05, ##p < 0.01 vs. the model group.
CUMS is a common paradigm employed to establish reliable animal models of depression and explore the mechanisms underlying this syndrome and other mental health disorders associated with it [17–20]. Animal models of depression typically show slower rates of weight gain, decreased food intake, anhedonia, and other core depression-like symptoms [21,22]. The sugar water preference test is a common method for examining anhedonia in animal models of depression as it can verify the animal’s reward-seeking behavior [23]. Meanwhile, the OFT allows us to observe the spontaneous activity in experimental animals and understand their exploratory behavior. Typically, the higher the severity of depression, the poorer is the autonomous activity in animals, and the lower is their tendency to explore a new environment [15,16]. In our study, CUMS model rats exhibited slower body weight gain, consumed less food, had a lower preference for sugar water, and explored the open field to a lower extent. These indicators were consistent with depression-like behaviors such as the loss of appetite, lack of pleasure-seeking behavior, and decreased spontaneous activity [24–26]. These findings demonstrated that a rat model of depression had been developed successfully.
Based on previous findings, it has been stated that microglia-related dysfunction is linked to the onset and progression of depression [27,28]. The hippocampus is mainly composed of neurons and gliocytes, and 20% of these gliocytes show macrophage-like features [29]. Under normal conditions, microglia remain in a resting phase. However, under conditions of pathological stimulation, these microglia are activated. Activated microglia acquire an amoeba-like morphology and secrete several inflammatory factors (IL-1β, TNF-α, IL-6, etc.), thus triggering inflammation in the nervous system [30]. The protein Iba-1 serves as a valuable marker of microglial activation [31]. Consequently, it is vital to decrease the neuroinflammatory response by preventing the overactivation of microglia.
The TLR4/NF-κB signaling pathway plays a crucial role in the polarization of microglia and the initiation of neuroinflammation [32]. TLR4, a key protein from the TLR family, exhibits high expression levels in microglia. NF-κB serves as a central mediator in the inflammatory response and the subsequent release of inflammatory factors. It is comprised of two subunits, P50 and P65, which form a dimer. The inhibitory protein, I-κB, remains in an inactive state in the cytoplasm. Upon stimulation by external factors, I-κB undergoes phosphorylation, leading to the dissociation of the NF-κB dimer. The NF-κB P65 subunit translocates to the nucleus where it activates the transcription of inflammation-related genes, thereby facilitating the release of inflammatory factors such as IL-1β, TNF-α and IL-6 [33]. Findings from previous studies have demonstrated that inhibiting TLR4 and NF-κB overexpression in microglia can exert protective effects on the nervous system and mitigate neuronal apoptosis [34,35]. In our study, we detected the presence of sparse and disordered neuronal cells as well as a decrease in Nissl bodies in the model group. A substantial elevation in the serum levels of IL-1β, TNF-α, and IL-6 and the hippocampal mRNA expression of these factors was also observed. Furthermore, the microglia in the hippocampus displayed a distinctive amoebic morphology, and this was accompanied by a substantial increase in expression and IOD values of Iba-1. Additionally, a conspicuous augmentation of NF-κB P65 and TLR4 expression, both at the mRNA and protein levels, was noted in the hippocampus. This demonstrated the induction of a neuroinflammatory response in rats from the model group.
Researchers have shown that quercetin is highly effective in treating depression. In the study by Shi et al. [36], the key genes associated with depression were identified, and the Chinese medicines corresponding to these genes with respect to depression management were analyzed. Based on a network pharmacology analysis of these Chinese medicines, the researchers screened out a total of four antidepressant ingredients, and the list included quercetin. In addition, some researchers have also explored the quercetin-mediated treatment of depression from a pharmacological perspective, demonstrating its effects on neurotransmitter regulation, the increased regeneration of hippocampal neurons, improvements in hypothalamic-pituitary-adrenal functions, as well as reductions in inflammation [4]. Meanwhile, other researchers have applied quercetin clinically to improve emotional states in subjects with cognitive impairment, showing that this compound can maintain redox homeostasis in the brain [6], in line with previous findings [4]. As demonstrated by Adeoluwa OA et al., quercetin can downregulate pro-inflammatory factors in the hippocampus and microglia, as well as improve behavioral indexes in rats with depressive-like behaviors [37]. Similarly, Tan et al. reported that quercetin is beneficial for treating neuropsychiatric symptoms associated with vascular dementia, as well as for regulating microglial phenotypes to attenuate the release of pro-inflammatory factors [38].
In this study, following quercetin administration, the experimental rats demonstrated noteworthy alterations in physiological and behavioral parameters, including body weight, food intake, preference for sugar water, motion squares, upright standing, as well as residence time and movement distance in the central region of an open field. Moreover, the organization and compactness of neurons were improved after quercetin administration, and Nissl bodies increased in number. Additionally, both the serum and hippocampus displayed markedly reduced IL-1β, TNF-α, and IL-6 expression. Furthermore, hippocampal microglia did not exhibit any significant amoebic changes and mainly showed branching. The expression and IOD values of Iba-1 were decreased, and TLR4 and NF-κB P65 levels in the hippocampus were decreased. These findings strongly suggested that quercetin exhibits a pronounced potential for ameliorating depression in preclinical models.
Ultimately, our research highlighted the effectiveness of quercetin in ameliorating aberrant behavior and pathological alterations in rats exhibiting depressive symptoms. Additionally, quercetin was found to facilitate morphological enhancements in microglia, lower the levels of Iba-1, and downregulate inflammatory markers in both the serum and hippocampal tissue. These findings underscore the significant contribution of quercetin in exerting antidepressant effects via the modulation of the TLR4/NF-κB inflammatory pathway and the suppression of excessive inflammatory cytokine production in hippocampal microglia.
Limitations and Future Directions
The pathogenesis of depression is complicated and unclear. Consequently, its treatment is associated with many challenges. Current drugs and psychotherapies typically only show benefits after a long duration and can also have several adverse effects. Moreover, the condition often relapses after discontinuing treatment. Therefore, alternative methods and drugs are urgently needed to treat depression. Despite its popularity as a health supplement, quercetin has received limited attention in studies on depression, including clinical and experimental studies.
Therefore, we selected the classical inflammatory pathway of TLR4/NF-κB signaling as the entry point and conducted a preliminary study on the impact of quercetin administration on depression-like behaviors in rats. We found that quercetin can mitigate the symptoms of depression via the regulation of the TLR4/NF-κB signaling pathway in hippocampal microglia and the downregulation of inflammatory factors. However, only key inflammatory factors, markers of microglia polarization, and key indicators of TLR4/NF-κB pathway activities were selected for relevant experiments in this study. Meanwhile, molecules upstream and downstream of these selected indicators were not thoroughly analyzed. Thus, in our future studies, we will further verify the findings of these studies and use blockers and inhibitors for reverse validation.
In addition, the dosage of quercetin employed in this study was only based on the oral dosage recommended for Chinese adults, and different dosage gradients were not employed for comparison. Thus, any potential dose-dependent relationship between the dose of quercetin and its therapeutic effects needs to be explored in detail, and this will be examined in future studies.
Finally, this study was only limited to rats, which are a preclinical model. In further studies, we will verify the results of this study at the cellular level, so as to provide a stronger experimental basis and theoretical basis for applying quercetin for clinically treating depression.
Acknowledgement: None.
Funding Statement: This experimental research work was supported by the National Natural Science Foundation of China (Nos. 81673881 and 81202644), Hebei Province Natural Science Foundation Traditional Chinese Medicine Joint Fund Cultivation Project (No. H2022423375), and Graduate Innovation Project of Hebei University of Chinese Medicine in 2023 (No. XCXZZBS2023003).
Author Contributions: SW and YL conceived and designed the study. YL, BZ, ZC, and PF conducted animal experiments and collected experimental data. YL was responsible for data statistics and analysis of results. YL wrote the paper. SW reviewed the manuscript. All authors reviewed the results and approved the final version of the manuscript.
Availability of Data and Materials: All data sets used and/or analyzed in this study can be made available from the corresponding author upon reasonable request.
Ethics Approval: The study was approved by the Animal Ethics Committee of Hebei University of Chinese Medicine (Shijiazhuang, China, Approval No. DWLL202203145).
Conflicts of Interest: All authors declare that they have no conflicts of interest with respect to this study.
References
1. Lewis WS, Freeman M, van Ommeren M, Chisholm D, Siegl OG, Kestel D. Mental health report: transforming mental health for all. Geneva: World Health Organization. Available from: https://iris.who.int/handle/10665/356119. [Accessed 2022]. [Google Scholar]
2. Secretariat. Global burden of mental disorders and the need for a comprehensive, coordinated response from health and social sectors at the country level. Geneva: World Health Organization. Available from: https://iris.who.int/handle/10665/78898. [Accessed 2012]. [Google Scholar]
3. Shamabadi A, Akhondzadeh S. Advances in alternative and integrative medicine in the treatment of depression: a review of the evidence. Arch Iran Med. 2021;24(5):409–18. doi:10.34172/aim.2021.59. [Google Scholar] [PubMed] [CrossRef]
4. Chen S, Tang Y, Gao Y, Nie K, Wang H, Su H, et al. Antidepressant potential of quercetin and its glycoside derivatives: a comprehensive review and update. Front Pharmacol. 2022;13:865376. doi:100.3389/fphar.2022.865376. [Google Scholar] [PubMed] [CrossRef]
5. Bellavite P. Neuroprotective potentials of flavonoids: experimental studies and mechanisms of action. Antioxidants. 2023;12(2):280. doi:100.3390/antiox12020280. [Google Scholar] [PubMed] [CrossRef]
6. Hayashi Y, Hyodo F, Tana, Nakagawa K, Ishihara T, Matsuo M, et al. Continuous intake of quercetin-rich onion powder may improve emotion but not regional cerebral blood flow in subjects with cognitive impairment. Heliyon. 2023;9(8):e18401. doi:100.1016/j.heliyon.2023.e18401. [Google Scholar] [PubMed] [CrossRef]
7. Ge C, Wang S, Wu X, Lei L. Quercetin mitigates depression-like behavior via the suppression of neuroinflammation and oxidative damage in corticosterone-induced mice. J Chem Neuroanat. 2023;132:102313. doi:100.1016/j.jchemneu.2023.102313. [Google Scholar] [PubMed] [CrossRef]
8. Wang H, He Y, Sun Z, Ren S, Liu M, Wang G, et al. Microglia in depression: an overview of microglia in the pathogenesis and treatment of depression. J Neuroinflamm. 2022;19(1):132. doi:100.1186/s12974-022-02492-0. [Google Scholar] [PubMed] [CrossRef]
9. Hu J, Mo JL, Cheng XL. Microglial TRPV1 in epilepsy: Is it druggable for new antiepileptic treatment? BIOCELL. 2023;47(8):1689–701. doi:100.32604/biocell.2023.029409. [Google Scholar] [CrossRef]
10. Gu JY, Xu YW, Feng LP, Dong J, Zhao LQ, Liu C, et al. Enriched environment mitigates depressive behavior by changing the inflammatory activation phenotype of microglia in the hippocampus of depression model rats. Brain Res Bull. 2021;177:252–62. doi:100.1016/j.brainresbull.2021.10.005. [Google Scholar] [PubMed] [CrossRef]
11. Xie H, Zhang C, Liu D, Yang Q, Tang L, Wang T, et al. Erythropoietin protects the inner blood-retinal barrier by inhibiting microglia phagocytosis via Src/Akt/cofilin signalling in experimental diabetic retinopathy. Diabetologia. 2021;64(1):211–25. doi:100.1007/s00125-020-05299-x. [Google Scholar] [PubMed] [CrossRef]
12. Li P, Huang W, Chen Y, Aslam MS, Cheng W, Huang Y, et al. Acupuncture alleviates CUMS-induced depression-like behaviors by restoring prefrontal cortex neuroplasticity. Neural Plast. 2023;2023:1474841. doi:100.1155/2023/1474841. [Google Scholar] [PubMed] [CrossRef]
13. Chen J, Luo Y, Liang X, Kong X, Xiao Q, Tang J, et al. Alteration in NMDAR subunits in different brain regions of chronic unpredictable mild stress (CUMS) rat model. Transl Neurosci. 2022;13(1):379–89. doi:100.1515/tnsci-2022-0255. [Google Scholar] [PubMed] [CrossRef]
14. Hu Y, Zhao M, Zhao T, Qi M, Yao G, Dong Y. The protective effect of pilose antler peptide on CUMS-induced depression through AMPK/Sirt1/NF-κB/NLRP3-mediated pyroptosis. Front Pharmacol. 2022;13:815413. doi:100.3389/fphar.2022.815413. [Google Scholar] [PubMed] [CrossRef]
15. Wang JY, Zhang Y, Chen Y, Wang Y, Li SY, Wang YF, et al. Mechanisms underlying antidepressant effect of transcutaneous auricular vagus nerve stimulation on CUMS model rats based on hippocampal α7nAchR/NF-κB signal pathway. J Neuroinflamm. 2021;18(1):291. doi:100.1186/s12974-021-02341-6. [Google Scholar] [PubMed] [CrossRef]
16. Shi ZM, Jing JJ, Xue ZJ, Chen WJ, Tang YB, Chen DJ, et al. Stellate ganglion block ameliorated central post-stroke pain with comorbid anxiety and depression through inhibiting HIF-1α/NLRP3 signaling following thalamic hemorrhagic stroke. J Neuroinflamm. 2023;20(1):82. doi:100.1186/s12974-023-02765-2. [Google Scholar] [PubMed] [CrossRef]
17. Antoniuk S, Bijata M, Ponimaskin E, Wlodarczyk J. Chronic unpredictable mild stress for modeling depression in rodents: meta-analysis of model reliability. Neurosci Biobehav Rev. 2019;99:101–16. doi:100.1016/j.neubiorev.2018.12.002. [Google Scholar] [PubMed] [CrossRef]
18. Xin H, Su S, Wu R, Wei L, Su N, Qi L, et al. Chronic unpredictable mild stress in rats based on the mongolian medicine. J Vis Exp. 2023;200. doi:100.3791/65889 10.3791/65889. [Google Scholar] [PubMed] [CrossRef]
19. Tong T, Chen Y, Hao C, Shen J, Chen W, Cheng W, et al. The effects of acupuncture on depression by regulating BDNF-related balance via lateral habenular nucleus BDNF/TrkB/CREB signaling pathway in rats. Behav Brain Res. 2023;451:114509. doi:100.1016/j.bbr.2023.114509. [Google Scholar] [PubMed] [CrossRef]
20. Zhang S, Lu Y, Shi W, Ren Y, Xiao K, Chen W, et al. SIRT1/FOXO1 axis-mediated hippocampal angiogenesis is involved in the antidepressant effect of Chaihu Shugan San. Drug Des Devel Ther. 2022;16:2783–801. doi:100.2147/DDDT.S370825. [Google Scholar] [PubMed] [CrossRef]
21. Pangemanan L, Irwanto I, Maramis MM. Psychological dominant stressor modification to an animal model of depression with chronic unpredictable mild stress. Vet World. 2023;16(3):595–600. doi:100.14202/vetworld.2023.595-600. [Google Scholar] [PubMed] [CrossRef]
22. Dandekar MP, Palepu MSK, Satti S, Jaiswal Y, Singh AA, Dash SP, et al. Multi-strain probiotic formulation reverses maternal separation and chronic unpredictable mild stress-generated anxiety- and depression-like phenotypes by modulating gut microbiome-brain activity in rats. ACS Chem Neurosci. 2022;13(13):1948–65. doi:100.1021/acschemneuro.2c00143. [Google Scholar] [PubMed] [CrossRef]
23. Liu J, Hester K, Pope C. Dose- and time-related effects of acute diisopropylfluorophosphate intoxication on forced swim behavior and sucrose preference in rats. Neurotoxicology. 2021;82:82–8. doi:100.1016/j.neuro.2020.11.007. [Google Scholar] [PubMed] [CrossRef]
24. Guo Q, Lin XM, Di Z, Zhang QA, Jiang S. Electroacupuncture ameliorates CUMS-induced depression-like behavior: involvement of the glutamatergic system and apoptosis in rats. Comb Chem High Throughput Screen. 2021;24(7):996–1004. doi:100.2174/1386207323666201027121423. [Google Scholar] [PubMed] [CrossRef]
25. Liu MY, Yin CY, Zhu LJ, Zhu XH, Xu C, Luo CX, et al. Sucrose preference test for measurement of stress-induced anhedonia in mice. Nat Protoc. 2018;13(7):1686–98. doi:100.1038/s41596-018-0011-z. [Google Scholar] [PubMed] [CrossRef]
26. Tao W, Dong Y, Su Q, Wang H, Chen Y, Xue W, et al. Liquiritigenin reverses depression-like behavior in unpredictable chronic mild stress-induced mice by regulating PI3K/Akt/mTOR mediated BDNF/TrkB pathway. Behav Brain Res. 2016;308:177–86. doi:100.1016/j.bbr.2016.04.039. [Google Scholar] [PubMed] [CrossRef]
27. Yirmiya R, Rimmerman N, Reshef R. Depression as a microglial disease. Trends Neurosci. 2015;38(10):637–58. doi:100.1016/j.tins.2015.08.001. [Google Scholar] [PubMed] [CrossRef]
28. Li B, Yang W, Ge T, Wang Y, Cui R. Stress induced microglial activation contributes to depression. Pharmacol Res. 2022;179:106145. doi:100.1016/j.phrs.2022.106145. [Google Scholar] [PubMed] [CrossRef]
29. Gong Y, Tong L, Yang R, Hu W, Xu X, Wang W, et al. Dynamic changes in hippocampal microglia contribute to depressive-like behavior induced by early social isolation. Neuropharmacol. 2018;135:223–33. doi:100.1016/j.neuropharm.2018.03.023. [Google Scholar] [PubMed] [CrossRef]
30. Jia X, Gao Z, Hu H. Microglia in depression: current perspectives. Sci China Life Sci. 2021;64(6):911–25. doi:100.1007/s11427-020-1815-6. [Google Scholar] [PubMed] [CrossRef]
31. Yin JJ, He Y, An J, Miao Q, Sui RX, Wang Q, et al. Dynamic balance of microglia and astrocytes involved in the remyelinating effect of ginkgolide B. Front Cell Neurosci. 2020;13:572. doi:100.3389/fncel.2019.00572. [Google Scholar] [PubMed] [CrossRef]
32. Chen J, Wang Z, Zheng Z, Chen Y, Khor S, Shi K, et al. Neuron and microglia/macrophage-derived FGF10 activate neuronal FGFR2/PI3K/Akt signaling and inhibit microglia/macrophages TLR4/NF-κB-dependent neuroinflammation to improve functional recovery after spinal cord injury. Cell Death Dis. 2017;8(10):e3090. doi:100.1038/cddis.2017.490. [Google Scholar] [PubMed] [CrossRef]
33. Duan L, Song L, Qiu C, Li J. Effect of the sEH inhibitor AUDA on arachidonic acid metabolism and NF-κB signaling of rats with postpartum depression-like behavior. J Neuroimmunol. 2023;385:578250. doi:100.1016/j.jneuroim.2023.578250. [Google Scholar] [PubMed] [CrossRef]
34. Zou XS, Shi L, Yin HL, Li HP, Wang MH, Song WC, et al. Compound Gaoziban tablet alleviates depression toll-like receptor 4/myeloid differentiation factor 88/nuclear factor-kappa B pathway. J Tradit Chin Med. 2022;42(6):956–64. doi:100.19852/j.cnki.jtcm.20220902.001. [Google Scholar] [PubMed] [CrossRef]
35. Wei H, Yu C, Zhang C, Ren Y, Guo L, Wang T, et al. Butyrate ameliorates chronic alcoholic central nervous damage by suppressing microglia-mediated neuroinflammation and modulating the microbiome-gut-brain axis. Biomed Pharmacother. 2023;160:114308. doi:100.1016/j.biopha.2023.114308. [Google Scholar] [PubMed] [CrossRef]
36. Shi Y, Chen D, Ma S, Xu H, Deng L. Identification of potential biomarkers of depression and network pharmacology approach to investigate the mechanism of key genes and therapeutic traditional chinese medicine in the treatment of depression. Evid Based Complement Alternat Med. 2021;2021:2165632. doi:100.1155/2021/2165632. [Google Scholar] [PubMed] [CrossRef]
37. Adeoluwa OA, Olayinka JN, Adeoluwa GO, Akinluyi ET, Adeniyi FR, Fafure A, et al. Quercetin abrogates lipopolysaccharide-induced depressive-like symptoms by inhibiting neuroinflammation via microglial NLRP3/NFκB/iNOS signaling pathway. Behav Brain Res. 2023;450:114503. doi:100.1016/j.bbr.2023.114503. [Google Scholar] [PubMed] [CrossRef]
38. Tan Z, Yang G, Qiu J, Yan W, Liu Y, Ma Z, et al. Quercetin alleviates demyelination through regulating microglial phenotype transformation to mitigate neuropsychiatric symptoms in mice with vascular dementia. Mol Neurobiol. 2022;59(5):3140–58. doi:100.1007/s12035-021-02712-3. [Google Scholar] [PubMed] [CrossRef]
Cite This Article
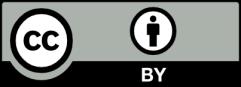
This work is licensed under a Creative Commons Attribution 4.0 International License , which permits unrestricted use, distribution, and reproduction in any medium, provided the original work is properly cited.