Open Access
REVIEW
Exercise and exerkine upregulation: Brain-derived neurotrophic factor as a potential non-pharmacological therapeutic strategy for Parkinson’s disease
Department of Biomedical Sciences, Sri Ramachandra Institute of Higher Education and Research, Chennai, 600116, India
* Corresponding Author: VENKATACHALAM DEEPA PARVATHI. Email:
(This article belongs to the Special Issue: Exploring the Cellular Mechanisms of Neurodegenerative Diseases)
BIOCELL 2024, 48(5), 693-706. https://doi.org/10.32604/biocell.2024.048776
Received 18 December 2023; Accepted 20 February 2024; Issue published 06 May 2024
Abstract
Physical activity and exercise have several beneficial roles in enhancing both physiological and psychological well-being of an individual. In addition to aiding the regulation of aerobic and anaerobic metabolism, exercise can stimulate the synthesis of exerkine hormones in the circulatory system. Among several exerkines that have been investigated for their therapeutic potential, Brain-derived neurotrophic factor (BDNF) is considered the most promising candidate, especially in the management of neurodegenerative diseases. Owing to the ability of physical activity to enhance BDNF synthesis, several experimental studies conducted so far have validated this hypothesis and produced satisfactory results at the pre-clinical level. This review highlights some of the recent animal model studies that have evaluated the efficiency of exercise in enhancing BDNF synthesis and promoting neuroprotective effects. Further, this review focuses on understanding the therapeutic benefits of exercise-induced exerkine synthesis as a non-pharmacological strategy in Parkinson’s disease (PD). Regarding physical activity and exerkine induction, the neuromuscular electrical stimulation (NMES) strategy could be considered as an alternate treatment modality for patients affected with PD.Graphic Abstract

Keywords
Parkinson’s disease (PD) is one of the most prevalent neurological disorders, clinically next to Alzheimer’s disease (AD). It is characterized by rigidity, tremors, and motor deficiency as a result of the death of dopaminergic neurons (DA) at the substantia nigra in the mid-brain [1]. It is commonly observed among the elderly, and with longer lifespans and with an increasing geriatric population, such neurodegenerative diseases are on the rise [2]. As per the Global Burden of Disease Study, the number of PD cases is estimated to reach nearly 15 million by 2040 [3]. It is consequential to bring about a therapeutic strategy to treat and increase the quality of life for patients with PD. With no cure for PD currently, research has shifted to controlling PD symptoms with strategies to supplement, stimulate, and maintain healthy DA neurons [4]. Recently, studies have shown the importance of physical activity and its potential benefits in treating a wide range of neurological disorders [5].
Exerkines are a class of proteins and nucleic acids, which are secreted by multiple body tissues in response to endurance exercise. The term myokines was first used to describe the secretions of interleukin-6 (IL-6) and other cytokines from the skeletal muscles. Apart from myokines, various other tissues also release cytokines into circulation, such as nephrokines from the kidneys, neurokines from the brain, and hepatokines from the liver [6]. Skeletal muscle acts as an endocrine organ by producing and releasing myokines. These later help in the maintenance of nervous, endocrine, and immune systems. Myokines were later defined to include peptides and nucleic acid cytokines, produced, expressed, and secreted from the muscle fibers [7].
The majority of exerkines released into circulation fall into one of two categories. These can either be classified as growth factors or could be nucleic acids like microRNAs (miRNAs). Growth factors such as vascular endothelial growth factor A and fibroblast growth factor 2 (FGF-2), among others, are found in large quantities in blood, following exercise. On the other hand, miRNAs have found to be involved in pathways such as myocardial remodeling and sarcoplasmic hypertrophy. miRNAs were initially thought to be restricted to be within the cell, but have also been detected in blood plasma; hence, these are known as circulating miRNAs. Similar to growth factors, miRNAs are also detected in the blood following intense exercise [8].
Since skeletal muscle contributes to 40% of the total body weight, they are considered to be amongst the largest organs in the body [9]. Hence, exerkines released have great potential to induce changes in multiple peripheral organs. Skeletal muscle serves as a factory for the synthesis of numerous myokines such as IL-6 family proteins, brain-derived neurotrophic factor (BDNF), FGF-2, and insulin-like growth factor 1(IGF-1). IGF-1 and FGF-2 are important in communication between the bone and skeletal muscle. By inducing myotube and myoblasts, BDNF facilitates muscle regeneration [10].
Exercise therapy was generally found to alleviate a few symptoms exhibited by patients with PD, such as a decline in physical and cognitive function. However, the precise factors responsible for such beneficial effects were unknown [11,12]. Besides releasing CO2, water, and energy from carbohydrates, muscles were also found to be secreting other independent cytokines. Although exercise-based cytokines were discovered, they were extracted, isolated, and characterized much later.
Decades later, exercise physiologists could determine the role of these ‘exercise-factors’ in the signaling of central and peripheral organs where the beneficial properties of these cytokines were understood [8,13,14]. Studies have shown a direct link between physical inactivity and the development of metabolic and aging-related diseases like diabetes mellitus, cardiovascular diseases, and other progressive neurodegenerative disorders. This review majorly focuses on understanding the influence of exercise in enhancing exerkine synthesis, particularly with emphasis on the role of BDNF in mediating neurogenesis in the PD-affected brain.
Regulatory Pathways of Exerkines
In humans, exerkines as well as other cytokines, are secreted in one of three ways, namely, exosomes, microvesicles, and apoptotic bodies. While the differentiation between the three classes remains ambiguous, exosomal secretion is widely accepted as they are important in both local and systemic cellular communication. Following aerobic exercises, a notable decrease in exosomes (containing exerkines) is observed within the skeletal muscle while, there is a simultaneous increase in the circulating serum. Thus, it leads to the creation of the term exersomes, referring to exosomes carrying exerkines [15].
The term extracellular vesicles (EV) encompasses different types of secretions, such as exosomes and microvesicles. Cells usually sequester peptides, nucleic acids, and other compounds in the form of intracellular vesicles. These vesicles are called endosomes. Upon fusion with the plasma membrane, they bud out and form vesicles called exosomes. Exosomes are 50–100 nm in diameter and are majorly composed of lipids and protein ligands. The exact composition of each exosome varies with the target tissue and the cargo. The surface-proteins on these EVs have a unique set of ligands, which aids in fingerprinting. This helps identify and characterize each exosome to its respective cargo [16].
Experimental studies have shown that upon AMP-activated protein kinase (AMPK) activation, IL-6 secretion from skeletal muscle increases. Many metabolic and transcriptional responses to exercise have been hypothesized to be regulated by 5-aminoimidazole-4-carboxamide riboside (AICAR) activation. AMPK has been linked to IL-6 expression and secretion in response to contraction. Exercise has been shown to increase AMPK activity in the muscle and IL-6 levels in the blood. Apart from IL-6, the AMPK-dependent pathway aids in determining the intracellular source and depletion kinetics of a variety of other myokines. A research study has found that intact muscle fibers act as endocrine cells by rapidly decreasing exosomal content in response to muscle contractions [17].
Apart from muscle contraction and exercise, studies have also employed mechanisms to study the effect of various synthetic/natural compounds that induce myokine secretion. Many such chemicals targeted induction of myokine production, i.e., upregulation/downregulation of one particular hormone. Only AICAR and leptin could mediate multiple myokine secretion. AICAR is an AMPK activator that plays a pivotal role in the synthesis of many myokines, including BDNF, IL-6, and irisin [18]. Meanwhile, leptin is a peptide that regulates energy balance by majorly acting on the adipocytes. They have the ability to induce the secretion of IL-6, IL-15, and irisin [19]. Proteomic and transcriptomic profiling of these myokines have revealed that they can carry out autocrine, paracrine, and endocrine actions [20].
Myokines have been shown to display autocrine pathways of regulation. Myokines such as IL-6 and BDNF were discovered to be acting on the muscle cells themselves to promote lipolysis, glucose uptake, and lipid oxidation. On the other hand, a few myokines, such as apelin, helped in the inhibition of muscle hypotrophy [21]. It is well known that skeletal muscle expresses a wide range of myokines, but it seems unlikely that AMPK is the only factor responsible for their release. Given the energy-saving nature of AMPK, it is possible that AMPK from the skeletal muscle is involved in the release of myokines that aid in the maintenance of skeletal muscle energy homeostasis. If so, myokines like IL-6, IL-15, BDNF, and leukemia inhibitory factors could be potential candidates [22].
Their paracrine actions include immune cell activation and differentiation, and extracellular matrix production and remodeling. These myokines recruit immune cells to control inflammation and muscle regeneration post-exercise. Apart from the immune system, exercise-derived myokines have been shown to positively impact the vascular and the central nervous systems [20].
Human-exercise studies have shown that the bioavailability of myokines decreases four hours post-exercise. This indicates the rapid absorption of myokines in distant organs. This also confirms the previously hypothesized multiple muscle-organ cross-talk, contributing to rapid metabolism [23]. Further studies are required to explore the mechanisms of muscle-brain cross-talk [21].
Significance of Exercise in Enhancing Exerkine Synthesis
Physical activity is defined as skeletal-muscle-mediated body movement, which burns more energy when compared to the resting state [24]. As per the current estimates, nearly one-third of the world population is physically inactive. The World Health Organization recommends 150 min of moderate-vigorous exercise per week, which is not met by the general population [25]. An unhealthy lifestyle is a consequence of the additive results of physical inactivity and improper diet patterns. These factors can lead to the development of hyperglycemia, hypertension, and obesity, which are the key hallmarks of metabolic disorders [26]. By preventing and treating many metabolic disorders, physical exercise plays a vital role as an ideal non-pharmacological therapeutic strategy. The benefits of physical activity attribute skeletal muscle with the maintenance of body homeostasis. Myokine secretion protects against physical inactivity and other related diseases [27]. Physical inactivity is consistently associated with an increased risk of neurodegenerative diseases in the older population [28]. With the increasing life expectancy due to better healthcare, geriatric care has become vital, and physical inactivity is especially significant in this population [29].
Aerobic as well as anaerobic training can involve isotonic and isometric muscle contractions. Isometric contractions do not cause a change in muscle length. They are not involved in limb/body movement. Whereas isotonic contractions involve a change in muscle length, thereby producing a muscle movement. Isotonic contractions mediate limb movement without change in muscle tension, such as walking, running, and cycling. They are also called endurance training (aerobic) because they involve highly repetitive but low-resistance movements. Contrarily, isometric contractions do not involve any joint movement but still undergo muscle tension. This includes exercises commonly incorporated in strength training regimes [30]. Thus, it is known as resistance training (anaerobic) owing to the high resistance and low-repeating movements. Most physical activities require both types of muscle contractions; however, the extent of their effectiveness is dependent on the type of contraction involved [31,32].
Aerobic exercise ensures the involvement of large muscles of the cardiovascular and respiratory systems, as oxygen is utilized, which is vital for losing fat. On the other hand, anaerobic exercise involves bursts of high-intensity activity independent of oxygen uptake, which helps increase muscle mass [24]. The anaerobic exercise showed increased circulating levels of asprosin, irisin, and IL-6 [33]. Myokines such as omentin, follistatin, and FGF-21 were secreted in response to both low and high-intensity endurance training. They were present in the circulating plasma in significant quantities. They also discovered that higher-intensity exercise induced greater levels of the same myokine when compared to low-intensity training [34]. Another study found the upregulation of myokines like BDNF, follistatin, decorin, and insulin sensitizing agent Metrl, post resistance training [35]. It was proven that growth factors are released after both aerobic exercise and resistance training. These growth factors, which are secreted from the skeletal muscle, include BDNF, IGF-1, and VEGF. Between aerobic exercise and resistance training, the circulating levels of myokines were higher in the case of aerobic/endurance exercise [36].
Cross-talk between muscle and the brain in response to exercise
Skeletal muscles comprise several muscle bundles called fascicles, which in turn, consists of muscle fibers called myofibrils. Hence, skeletal muscles have a complex anatomical organization and exhibit various physiological activities in response to their usage associated with voluntary movements. Being an abundant tissue in the human body, which also acts as a storage site of major biomolecules, such as, glucose, amino acids, and fatty acids, the muscles are, therefore, important regulators of whole-body metabolic homeostasis. Skeletal muscles are involved in a myriad of activities like maintaining body posture, generation of force along with power while during movements that are voluntary, and, most importantly, breathing and thermoregulation [37].
Physical activity in any form particularly exercise, has a plethora of benefits that help enhance the anatomical, physiological, and biochemical characteristics of the skeletal muscle. With special emphasis, several studies conducted in the past have brought some perspectives about the beneficial effects of physical activity in the form of aerobic and resistance exercise on brain health and function [38–40]. Apart from a scientific point of view, perspectives from historical as well as evolutionary aspects tend to suggest that there is a significant association between muscles and the organ–brain [41]. Exercise has several instrumental effects on brain health by alleviating symptoms of stress and depression, reducing the risk of developing neurological disorders like dementia and Alzheimer’s disease, and maintaining metabolic control as well as cognitive function [41,42]. Recently, studies have been conducted using animal models to specifically deconstruct the mechanisms behind the beneficial effects of physical exercise-induced spatial learning and memory improvement, including neurodegenerative conditions [40].
Studies conducted ‘hitherto’ have identified that almost a large network of areas in the brain that is nearly 82% of the net grey matter volume can be modified by the effects of exercise [43]. Studies conducted in mouse models have demonstrated that exercise results in an increased blood supply as well as an increased volume of the hippocampal region of the brain [44]. Similarly, experimental results of several other mouse model studies displayed that exercise leads to increased synaptic plasticity along with the induction of morphological changes in dendrites [38,45]. Given that the brain can sense physical activity, as evidenced by morphological and physiological changes, [43,44] these results suggest that there should be a cross-talk between muscle and the brain through muscle-induced peripheral factors [41].
Vigorous findings related to exercise and brain health indicate the presence and existence of the muscle-brain endocrine loop. However, their clarity regarding which peripheral mechanism brings positive, beneficial effects of exercise is lacking [41]. Nevertheless, studies have elucidated that skeletal muscle functions as an endocrine organ. These muscles have the ability to produce and secrete hormones called as myokines that deploy their function either in autocrine, paracrine, or endocrine ways [46]. Recent advanced studies have portrayed the fact that skeletal muscles process myokines in response to physical exercise, which thereby allows cross-talk between the muscle and brain, including other organs and components such as liver, gut, adipose tissue, bone, pancreas, vasculature bed, skin as well as within the muscle itself [41,46]. In addition to circulating myokines, many other exerkines (including peptides, nucleic acids metabolites) that are released by the skeletal muscle in response to exercise, direct feedback from the muscle through the peripheral nervous system and central nervous system (CNS) to the brain, is involved in muscle-to-brain communication as well [41].
Cross-talk between the brain and the muscle, in turn, is regulated by the blood brain barrier (BBB), which primarily separates components of the CNS- brain and spinal cord from the rest of the peripheral organs [47]. For maintenance of homeostasis in the CNS, BBB takes the lead control and regulates the transfer of nutrients and cells from the blood to the brain and vice-versa. BBB restricts the entry of peripheral inflammatory mediators (antibodies, cytokines) and also aids in the clearance of harmful toxins from the brain to blood, and regulates the volume and composition of the cerebrospinal fluid (CSF) [48]. Similar to the effects of physical activity in enhancing muscle-brain cross-talk, there is a cross-talk between physical activity and BBB permeability. This is of interest because pathological changes caused to the brain due to genetic mutations or secondary to other neurodegenerative conditions can lead to aberrations in the CNS microvasculature, thereby affecting BBB. These factors have the potential to affect the permeability of BBB and can result in its breakdown and cause several neurodegenerative disorders such as AD, multiple sclerosis, and PD [49]. Interestingly, there is a strong theoretical framework suggesting that physical activity is effective in modulating the BBB permeability in several neurodegenerative diseases, owing to its influence on systemic inflammation, the nor-adrenergic systems, and brain renin-angiotensin systems, central autonomic function, and the kynurenine pathway [48].
Synergism between Exercise-Exerkine and Dopaminergic Neurons Regulation
Exercise has been demonstrated through several pre-clinical studies to be invaluable in alleviating oxidative stress and damage caused to DA during PD progression [50,51]. This is partly attributed to the enhanced secretion of exerkines into the circulatory system, which confers neuroprotective effects on the brain [52]. A classic example of exercise in providing neuroprotection to animal models of PD includes the work of Zigmond and his team. The authors have demonstrated the beneficial effects of exercise in attenuating the behavioral deficits caused in a neurotoxic mouse model of PD. Such behavioral deficits are incurred because of the loss of DA neurons along with the effect of dopaminergic toxins [53]. A group of researchers employed a heterozygous knockout (KO) mouse of BDNF +/− and compared the dopaminergic output with respect to wild-type (WT) controls. Both groups of mice were enrolled in a 30-day voluntary exercise regimen by means of wheel-running to examine the exercise dependent DA release. Two regions of the brain were particularly analyzed to test the difference in BDNF-dependent DA secretion. They were the dorsal striatum (dStr) and in the nucleus accumbens (NAc) shell and core. After the 30-day exercise regimen, an enhancement of DA in WT, along with the absence of DA secretion in BDNF +/− KO, pointed to the vital role of BDNF in the synthesis of DA. The results of NAc core and dStr were congruent with the hypothesis due to their involvement in the nigrostriatal pathway [54].
Another similar study concluded two endpoints favoring the positive link between BDNF and DA. Striatal dopaminergic deficient mice models were developed by artificially injecting lipopolysaccharide into the peritoneum. To reverse this induced state, the mice were enrolled in a 4-week exercise regimen, which showed healing effects in the striatum. The first endpoint showed that exercise regimen restored low DA levels and DA neurons. The second endpoint dealt largely with the mechanism behind the restoration of DA neurons. It was shown that when mice were administered with tropomyosin receptor kinase B (TrkB) receptor antagonist, a similar reversal of dopaminergic deficiency was not observed, thus pointing to the importance of the TrkB-BDNF pathway in nigrostriatal maintenance [55]. As shown in Fig. 1, we can observe the BDNF cascade and its mechanism of action via the TrkB and cAMP-response-element-binding protein (CREB) pathway. Additional studies have shown the molecular mechanism behind DA upregulation [56]. When BDNF binds with TrkB receptors, it promotes CREB protein expression, which leads to the production of tyrosine hydroxylase (TH). TH plays a vital role in DA secretion as it converts L-tyrosine to L-DOPA, which serves as the precursor for DA. The resulting DA enhances neuronal survival and differentiation [57].
Figure 1: The muscle-contraction-derived BDNF is upregulated in a proliferator-activated receptor gamma coactivator 1-alpha (PGC-1α)/fibronectin type III domain-containing protein 5 (FNDC-5)-dependent manner. The brain-derived neurotrophic factor (BDNF) thus produced confers neural longevity and memory via the TrKB and CREB pathways, respectively.
Human mesenchymal stem cells (hMSCs) were established from dental pulp, adipose tissue, and bone marrow. Later, when exposed to BDNF, it induced neuronal cell lineages. Differentiated cells were confirmed with the presence of biomarkers like TH and nestin. Among the three tissue sources, dental pulp showed the greatest efficiency in differentiating into DA. Therefore, the dopaminergic cell phenotype was achieved in vitro with minimal induction. hMSCs thus have great potential to develop functional DA models for both drug testing and transplantation purposes [57].
Additional neurotrophic factors (NTFs), particularly, glial cell line-derived neurotrophic factor (GDNF) and cerebral DA neurotrophic factor (CDNF), function similarly to BDNF in many aspects. GDNF functions by activating a canonical receptor tyrosine kinase (RTK) called rearranged during transfection and confers protection to DA from α-synuclein aggregates, as observed in both in vitro and in vivo PD models [58]. α-synuclein build-up leads to the formation of aggregates known as Lewy bodies that affect neurotransmission and is one of the key hallmarks of PD [59]. Whereas, CDNF and its homologous protein mesencephalic astrocyte-derived neurotrophic factor have been linked with neurorestorative function by regulating endoplasmic reticulum stress from within the cells. CDNF knockout was found to decrease dopaminergic neurotransmission in mice striatum, likely due to abnormal DA transporter (DAT) [60]. Both GDNF and CDNF help overcome neurodegeneration by increasing survival via the phosphoinositide 3-kinase/protein kinase B (PI3K/Akt) pathway, as observed in BDNF [61]. Rodent studies have demonstrated that high-intensity endurance and treadmill training can improve CDNF synthesis in the cerebral cortex and PD-induced spinal cord, respectively [62,63]. Further, it was observed that the introduction of exogenous CDNF rescues neurodegeneration at the corpus striatum. Zigmond and colleagues proclaimed that by performing exercise, there is an enhanced expression of neurotrophic factors, especially GDNF. They also indicated that GDNF can lower the damage engendered to DA neurons owing to the activation of important intracellular cascading molecules. Their work signified the intrinsic ability of DA neurons to respond to both intracellular and extracellular stress signals by activating endogenous neuroprotective mechanisms [53].
Exercise-Induced Brain-Derived Neurotrophic Factor Synthesis
Upregulation of brain derived neurotrophic factor
Endurance exercise was found to be beneficial in the prognosis of neurodegenerative diseases with the discovery of the peroxisome proliferator-activated receptor gamma coactivator 1-alpha (PGC-1a)/fibronectin type III domain-containing protein 5 (FNDC5)/irisin/BDNF pathway as shown in Fig. 1. Skeletal muscle undergoing contraction releases PGC-1a, which later synergistically acts along with estrogen-related receptor proteins to induce the expression of FNDC5 gene [64]. FNDC5 is a membrane protein that gets activated in a PGC-1a-dependent manner. It is initially produced in the contractile state of the skeletal muscle and is secreted following proteolytic cleavage [65]. The circulating form is known as irisin, which is one of the newly discovered myokines [66].
Irisin is directly linked with the upregulation of BDNF and improving the overall cognitive and memory function. Studies have shown the ability of irisin to regulate neuroprotective function in the brain directly or via other neurotrophin molecules [67]. Given that the PGC1a/FNDC5/irisin/BDNF pathway has been identified as a critical mechanism in exercise-induced neuroprotection, it can potentially be used for a variety of therapeutic purposes. BDNF is synthesized by neurons and promotes many intracellular signaling events via TrkB receptors, enhancing CREB activity and PGC1a expression. It all usually equates to improved overall brain activity. Numerous neurodegenerative conditions, such as AD and PD, have directly benefited from the neuroprotective effects of exercise. Nonetheless, the mechanisms by which exercise improves the neuro-disease condition are still not clear. It is widely accepted that exercise boosts the production of neurotrophic factors like BDNF and other factors that enhance the survival and activity of neuronal tissues in general [68].
Molecular Pathways Involved in Brain Derived Neurotrophic Factor Action on Dopaminergic Neurons
BDNF is a neurotrophic growth factor synthesized along with neurotrophins. It is initially synthesized in its inactive form (pro-BDNF) at the endoplasmic reticulum. It is later transported to the Golgi apparatus for packaging into vesicles, where it is activated to mature BDNF (mBDNF) [69,70]. This conversion is mediated by metalloproteinases known as carboxypeptidase E (CPE) [71]. BDNF signaling is mediated by both TrKb and CREB pathways and is represented in Fig. 1. While the TrKb pathway promotes neural survival, growth, and synaptic plasticity, the CREB pathway upregulated the mechanism of learning and memory in the hippocampus [72].
BDNF actively binds to TrkB, a member of the RTKs superfamily, and is found in the brain. BDNF binding induces dimerization and auto-phosphorylation of the tyrosine residues, which activates G proteins. These activated G proteins then modulate secondary signaling via Ras/mitogen activated protein kinase (MAPK), PI3-kinase, and phospholipase-C-γ (PLC). Ras acts as a common inducer for the activating MAP kinase, PI3-kinase, and PLC pathways. Ras activates the MAPK/ extracellular signal-regulated kinases (ERK) pathway, which is critical for the survival of the neuron as it inhibits pro-apoptotic factors and promotes the expression of pro-survival genes. Ras also promotes the uptake and capture of pro-apoptotic factors via the PI3K/AKT pathway. Additionally, the Ras phosphorylates PLC-γ protein, which relays the activation of the diacylglycerol/inositol trisphosphate pathway. Throughout life, the BDNF-TrkB signaling pathway in the CNS performs a wide range of functions, including cell survival, migration, synaptogenesis, and synaptic repairing. While the same neurotrophins bind to TrkB, they are involved in many signaling pathways [73–75].
These pathways finally result in the upregulation of transcription factors like CREB and CREB-binding protein (CBP) that promote the expression of pro-survival genes in neuronal cells [71]. In cultured neurons, it was discovered that, upon exposure to BDNF, the neurons upregulate the gene expression via activation of transcription factor CREB.
CREB is phosphorylated by the activation of the calcium-calmodulin-dependent kinase pathway and Ras-dependent pathway. It was observed that BDNF performed the following function via the CREB activation.
BDNF exposure in cortical neurons increased the secretion of neurotransmitters and the formation of new synaptic junctions, and improved axonal sprouting and dendritic growth [76]. The BDNF plays a pivotal role in neurogenesis by controlling the growth of dendrites. As previously seen, BDNF promotes the survival of neurons by several pathways. Further, BDNF also induces Ca2+ signaling, which serves to improve synaptic plasticity. BDNF, through TrkB signaling, has been shown to inhibit the termination of GABAergic neurons in the substantia nigra, which plays a therapeutic role in PD. Moreover, it also causes the enlargement of nigral neurons. Contrarily, the decrease in BDNF levels in plasma has been indicated in many neurological disorders like PD, AD, Huntington’s disease, and bipolar disorders [71].
Brain-Derived Neurotrophic Factor Expression During Aging
In older population the circulating concentration of BDNF declines. This contributes to various neurological symptoms, as observed in geriatric patients. They include cognitive decline, cerebral atrophy, and loss of neurons along with synaptic plasticity [77]. Contrarily, studies have shown that BDNF can protect the brain from neurodegenerative disorders in the aged population. The exerkine particularly protects the hippocampal and the dentate gyrus regions of the brain. However, due to declining levels of BDNF, its protective nature also gets weakened [78]. Similar studies have also proven the multifactorial decline in the BDNF pathway. It was shown that apart from BDNF, FNDC5, PGC-1α, and other signaling peptides were drastically reduced from circulation in aged individuals [79].
Importance of exerkine metabolism in influencing Parkinson’s disease pathophysiology.
Current non-pharmacological treatment strategies for Parkinson’s disease.
Non-pharmacological treatment modalities for PD range from conventional complementary and alternate therapies to modern therapeutic modalities. Complementary therapies are beneficial to patients with PD because they can offer both symptomatic relief along with disease-modifying strategies. Physical activity in the form of exercises has been identified to confer enormous benefits to patients with PD because it can attenuate the impact of motor dysfunction symptoms and improve cognitive abilities [80,81]. Further, recent studies have highlighted the potential of physio-exercises in providing neuroprotective effects to PD animal models by increasing the synthesis of neurotrophic factors, enhancing mitochondrial function, and stimulating neuroplasticity [82]. More precisely, recent clinical trials have further highlighted that aerobic exercise such as aerobic walking and stretching could mitigate some of the PD motor dysfunction symptoms, namely, gait, balance, physical performance, and some non-motor symptoms, such as depression, fatigue, and cognition, but not for the prevention of fall in PD affected patients [83,84]. Treatment modalities such as acupuncture, qi gong, and tai chi practices, mindfulness meditation, yoga, and other relaxation techniques such as dance and music therapy, usage of traditional herbal medicines and utility of nutrition supplements, and life-style habits have also conferred therapeutic benefits to patients with PD. Tai chi, an ancient Chinese physical exercise, combined with conscious breath control, slow movements, and relaxation, has been identified to be beneficial in reducing falls and balance impairments along with additional functional improvement. This combined exercise and conscious breathing strategy has been proven to be a safe and feasible therapeutic strategy that could be used for patients with mild to moderate severity of PD [85].
In addition to the applicability of conventional treatment modalities for improving PD symptoms, recent advancements in non-pharmacological therapies have further revolutionized PD treatment strategies. Deep brain stimulation, a surgical technique that helps provide electrical stimulation to the sub-thalamic nucleus and internal segment of the globus pallidus, helps in the modulation or disrupting patterns of neural signaling. This electro-stimulated therapy was found to ameliorate motor symptoms of PD [86].
Specifically, sub-thalamic nucleus-deep brain stimulation could be preferentially used in the management of patients with advanced PD with predominant posture and gait disorders owing to the improvements in off time [87]. Despite the benefits of DBSs in improving the defects caused in PD-associated motor symptoms, only less than 5% of the population is suitable for undergoing this surgical procedure [88]. With the advancements in the past two decades, repetitive transcranial magnetic stimulation (rTMS) has been monitored closely to be considered as a possible therapeutic modality for patients with PD. As a non-invasive procedure, rTMS therapy aims to deliver repeated magnetic pulses to a specific region in the brain within a short period of time. This procedure is achieved by placing a stimulation coil over the scalp, which does not require any usage of anesthetics or surgical maneuvers. The repeated delivery of magnetic pulses alters the excitability of the local stimulation site but also has the propensity to influence distant brain regions through the cortico-basal ganglia-thalamo-cortical motor circuit [89]. In addition, different types of rTMS aid in causing different responses of cortical excitability.
Although rTMS is beneficial due to its non-invasive technical aspects, the potential risk of developing seizure, as well as a small risk of scalp pain with transient headaches, should be of concern [90]. Near-infrared light (NIr) therapy is selectively used for treating tissue contusion where pre-clinical studies using animal models have revealed that NIr could improvise behavioral deficits and dopaminergic neuronal survivals in PD mouse models [91].
Existing exerkines in the treatment of Parkinson’s disease.
There are several exerkines whose functions have been validated through extensive experimental studies; a list of those few exerkines, along with their source of origin and function, are listed in Table 1.
PD is characterized by the loss of DA neurons via dysregulated autophagy and endoplasmic reticular stress (ERS). Autophagy ensures that abnormal proteins and damaged organelles are properly degraded to maintain normal cell morphology. Failure to undergo autophagy results in the accumulation of intracellular components and reactive oxygen species (ROS). In an earlier study, rat models were artificially induced with dopaminergic neurodegeneration. Later, apelin-13 was administered via intra-nigral injection in rats to test their beneficial effects. It was inferred that α-synuclein protein aggregation was responsible for inducing lysosomal organelle degradation (autophagy) and ERS. Apelin-13 successfully helped prevent the aggregation of α-synuclein by improving its clearance rate. Thus, apelin-13 exhibited neuroprotective behavior against PD [106]. Other studies performed on human neuroblastoma cell lines have showcased the anti-apoptotic and anti-oxidant properties of apelin-13. Exposure to apelin-13 lowered levels of ROS, which in turn, prevented the activation of caspase-3 and cytochrome-c release, required for the onset of apoptotic cascade [107,108].
In PD mouse models, contraction-dependent CatB was found to remove α-synuclein aggregates in the brain due to its autophagic and lysosomal activities, making it an effective myokine [109,110]. CatB enhanced DA secretion by upregulating key transcription factors required for the expression of TH. TH, in turn, amplifies the conversion of tyrosine, thereby upregulating the DA synthesis. Overall, CatB was concluded to be responsible for reversing the artificially induced neurotoxicity [111].
Research studies have discovered that following physical exercise, the uptake of circulating IGF-1 increases tremendously in the brain region. IGF-1 is taken up via the blood-CSF region. Once in the substantia nigra, the IGF-1 exerts a neuroprotective effect by mimicking BDNF function [112]. IGF-1 promotes neuronal survival via PI3K/Akt. The pathway taken by IGF-1 is responsible for motor behavior and DA neuron viability. Thus it improves the PD pathology [113].
FGF-21 was administered to neuroblastoma and primary DA cell line, which was artificially induced to develop PD morphology. FGF-21 treatment was found to inhibit loss of TH and improved mitochondrial function via the PGC-1α pathway activation [114]. In the studies mentioned above, PD morphology was artificially induced by treating with a compound called 1-methyl-4-phenylpyridinium (MPP+). Alternatively, its precursor molecule, 1-methyl-4-phenyl-1, 2, 3, 6-tetrahydropyridine (MPTP) can also be used to cause the symptoms of PD by promoting the loss of DA neurons at the substantia nigra. Both MPP and MPTP are potent neurotoxins, which are used to understand PD pathophysiology in animal and cell culture models better. They induce intracellular oxidative stress, which eventually leads to apoptosis [115].
Musclin is another exercise-derived myokine that is functionally identical to natriuretic peptides (NPs). NPs indicated higher mitochondrial biogenesis via cyclic guanosine monophosphate (cGMP)/PGC-1α pathway. PGC-1α is a known player in dopaminergic neuroprotection. Musclin contributes significantly in the regulation of NPs. One of the major symptoms observed in patients with PD is the oxidative stress of DA neurons associated with improper mitochondrial function. Thus, musclin is of great importance while considering therapeutic solutions to PD [116].
Drawbacks of Alternative Therapies to Treat Parkinson’s Disease (Administration of Neurotrophic Factors, Plasma Transfusion)
NTFs are endogenously produced proteins that are usually secreted in astrocytes, Schwann, and glial cells. The NTF superfamily comprises neurokines, neurotrophins, and other neurotrophic factors. This family of proteins is vital for the survival and differentiation of neurons and in the regulation of synaptic transmission and plasticity. They have also been shown to protect neurons from neurodegeneration in animal models of PD. Although the available animal models accurately recapitulate the pathophysiology of PD, they fail when depicting PD progression timeline in humans. In the case of direct infusion systems, the location of the drug delivery system to be implanted is still highly debated [117].
Currently, the treatment of PD involves the external administration of neurotrophic factors to induce synthesis. Gene/cytokine administration via viral/non-viral vectors has shown to be a viable option to upregulate BDNF within the CNS. However, in humans, this technique is associated with several limitations, like the possibility of viral infection and the elicitation of immune response. It is also well-known that BDNF can be synthesized endogenously by muscles through physical exercise. Exercises like aerobics, endurance training, resistance training or a combination of the two have indicated a decrease in PD symptoms. An increase in serum BDNF levels was discovered only after several weeks of intense exercise regimens. Although an increase was detected after short workouts, the amount of reabsorbed BDNF outweighed the amount initially secreted by the skeletal muscle, hence quickly bringing down the circulating levels back to normal [56]. One study hypothesized the direct intra-putamen delivery of NTFs would likely be very invasive and cause many adverse reactions. Even if the current clinical trials prove effective, NTFs can only be employed as a defensive mechanism in younger patients with PD to provide neuroprotection to the intact remaining nigrostriatal DA. Optimization of delivery methods is vital for the success of NTF treatment [118,119].
Although physical activity has been attributed to improved prognosis of neurodegenerative disorders, it also has several limitations. The major drawback being the inability of geriatric patients to undergo rigorous exercise regimens [120]. A cross-sectional study compared patients with PD who performed physical exercise vs. those who did not. The main reason for inactivity in geriatric patients with PD was found to be due to the dread of falling and the reduced anticipation of favorable outcomes. Whereas, in younger patients with PD, the most prevalent reasons for their inability to exercise was inconvenience and lack of time [121]. To circumvent this age-dependent challenge, studies have attempted to understand the potential of blood transfusion from exercised individuals to patients suffering from such ailments.
In one such study, the transfusion of plasma from both agile and sedentary mice was analyzed to study their therapeutic effect against neurodegenerative disorders. Here, plasma was extracted from exercised young mice population and transferred to the naïve mice population. This was repeated over eight times in the period of 3 weeks. The blood was transferred either by parabiosis or plasma transfusion. It was found that plasma transfusions from exercised mice improved the number of new neurons in the hippocampus region. Additionally, the hippocampal expression of mature neurons also increased in the naïve mice [120].
Brain-Derived Neurotrophic Factor Synthesis through Neuromuscular Electrical Stimulation (NMES) Strategy–Future Perspectives
Exerkines are a group of hormones that are generally synthesized after performing physical activities such as aerobic and endurance training. Among all the exerkines investigated so far, BDNF appears very promising owing to its multi-beneficiary functions. One major advantage with regard to BDNF is that by performing physical activity, the levels of BDNF are further upregulated, which has been clearly evidenced by the sheer number of experimental studies. Studies have established a direct relationship between the intensity of contraction and the BDNF secretion. In studies involving human subjects with early-stage PD, the group undergoing rigorous, intensive exercise had higher levels of BDNF, which in turn also improved the PD prognosis. Although both the control and intervention subjects were prescribed the same medication, no significant changes were seen in the control group, that did not participate in any exercise regimen [122]. Another similar pilot study found increased serum BDNF levels post-high-intensity training compared to no or moderate-intensity training. However, these changes were observed over a longer period of training period, and individual training sessions did not change BDNF levels significantly [123]. Although such studies show a correlation between rigorous exercise and BDNF levels in patients with PD, the exact exercise regimen and modality still remain largely debated. Regardless, the results of such studies are in line with the trend observed in similar animal models [124–126].
In an experimental setup, skeletal muscle cells were cultured in a custom-made humidified chamber and subjected to electrical stimulation. The contracting skeletal muscle upregulated both BDNF mRNA and the BDNF proteins. Additionally, along with the mature form of BDNF, the pro-form was also secreted. This precursor (pro-BDNF) could be activated by the action of matrix metalloproteinases [127].
Similar studies showed that BNDF was secreted in the soleus and medial gastrocnemius muscles in rats when the sciatic nerve was electrically stimulated. The nerve was stimulated at different pulse rates, durations, and frequencies. The quantity of BDNF produced via electrical stimulation was greater than that produced during comparable levels of physical exercise. The amount of BDNF synthesized was found to vary depending on the type of muscle or nerve fiber stimulated. The study concluded that longer duration and higher electrical stimulation frequencies produced greater volumes of BDNF and vice-versa [128]. NMES is a non-invasive electric stimulation technique that applies electric current across the superficial muscles present beneath the skin. It achieves muscular contraction via conductive pads placed on the skin surface. This technique was previously used widely in non-ambulatory patients to promote artificial muscle contraction. The expression of BDNF in plasma was found to be consistently higher in patients who have undergone an NMES regimen. Even a 30-min NMES-treatment induced a marked increase in BDNF levels in the plasma [129].
As evidenced through such experimental studies, such exercise-derived BDNF through electrical stimulation on the skin surface could be employed on human subjects with special emphasis on patients with PD. This is because of the ability of BDNF myokines in enhancing neurogenesis and differentiation of DA.
Currently, the treatment of PD utilizing BDNF involves either the use of gene therapy or the induction of endogenous BDNF synthesis. Gene therapy via viral/non-viral vectors has shown to be a viable option in animal models to upregulate BDNF within the CNS. However, in humans, this technique is associated with several limitations, owing to the possibility of viral infection and elicitation of immune response.
PD is a complex progressive neurodegenerative disorder whose treatment strategy requires a thorough evaluation of underlying molecular pathophysiology. Of all the strategies currently available to treat PD, a growing body of evidence highlights the significance of physical activity in alleviating symptoms of PD. Of note, exercise-induced exerkine synthesis, particularly BDNF, has been identified to be regulating some of the key pathways that are deregulated during PD. In addition, the emergence of an NMES strategy could further pave the way for a non-invasive approach to enhancing therapeutic benefits associated with exercise-induced BDNF synthesis. Translation of research from pre-clinical models (bench) to clinical scenarios (bedside) is inevitable for improvising the existing non-pharmacological modes of therapy for patients with PD.
Acknowledgement: None.
Funding Statement: The authors received no specific funding for this study.
Author Contributions: The authors confirm their contribution to the paper as follows: Study conception, design, and supervision: VDP. Study design and draft manuscript preparation: VVP and JSS. VVP and JSS have contributed equally to the work. All authors reviewed the manuscript and approved the final version of the manuscript.
Availability of Data and Materials: Data sharing is not applicable to this article as no datasets were generated or analyzed during the current study.
Ethics Approval: Not applicable.
Conflicts of Interest: The authors declare that they have no conflicts of interest to report regarding the present study.
References
1. Prasad EM, Hung SY. Current therapies in clinical trials of Parkinson’s disease: a 2021 update. Pharm. 2021;14(8):717. [Google Scholar]
2. Jankovic J, Tan EK. Parkinson’s disease: etiopathogenesis and treatment. J Neurol Neurosurg Psychiatry. 2020;91(8):795–808. [Google Scholar] [PubMed]
3. Ou Z, Pan J, Tang S, Duan D, Yu D, Nong H, et al. Global trends in the incidence, prevalence, and years lived with disability of Parkinson’s disease in 204 countries/territories from 1990 to 2019. Front Public Heal. 2021;9:776847. [Google Scholar]
4. Marino BLB, de Souza LR, Sousa KPA, Ferreira JV, Padilha EC, da Silva CHTP, et al. Parkinson’s disease: a review from pathophysiology to treatment. Mini-Reviews Med Chem. 2020;20(9):754–67. [Google Scholar]
5. Kantawala B, Ramadan N, Hassan Y, Fawaz V, Mugisha N, Nazir A, et al. Physical activity intervention for the prevention of neurological diseases. Heal Sci Rep. 2023;6(8):1–6. doi:10.1002/hsr2.1524. [Google Scholar] [PubMed] [CrossRef]
6. Pedersen BK. Muscles and their myokines. J Exp Biol. 2011;214(2):337–46. [Google Scholar] [PubMed]
7. Pedersen BK, Åkerström TCA, Nielsen AR, Fischer CP. Role of myokines in exercise and metabolism. J Appl Physiol. 2007;103(3):1093–8. doi:10.1152/japplphysiol.00080.2007. [Google Scholar] [PubMed] [CrossRef]
8. Safdar A, Tarnopolsky MA. Exosomes as mediators of the systemic adaptations to endurance exercise. Cold Spring Harb Perspect Med. 2018;8(3):a029827. doi:10.1101/cshperspect.a029827. [Google Scholar] [PubMed] [CrossRef]
9. Legård GE, Pedersen BK. Muscle as an endocrine organ. In: Muscle and exercise physiology. London: Academic Press Ltd-Elsevier Science Ltd.; 2019. p. 285–307. [Google Scholar]
10. Pedersen BK, Febbraio MA. Muscles, exercise and obesity: skeletal muscle as a secretory organ. Nat Rev Endocrinol. 2012;8(8):457–65. doi:10.1038/nrendo.2012.49. [Google Scholar] [PubMed] [CrossRef]
11. Feng YS, Yang SD, Tan ZX, Wang MM, Xing Y, Dong F, et al. The benefits and mechanisms of exercise training for Parkinson’s disease. Life Sci. 2020;245:117345. [Google Scholar] [PubMed]
12. Alberts JL, Rosenfeldt AB. The universal prescription for Parkinson’s disease: exercise. Bloem BR, Brundin P, editors. J Parkinsons Dis. 2020;10(s1):S21–7. doi:10.3233/JPD-202100. [Google Scholar] [PubMed] [CrossRef]
13. Pedersen BK, Steensberg A, Fischer C, Keller C, Keller P, Plomgaard P, et al. Searching for the exercise factor: is IL-6 a candidate? J Muscle Res Cell Motil. 2003;24(2–3):113–9. [Google Scholar] [PubMed]
14. Goldstein MS. Humoral nature of the hypoglycemic factor of muscular work. Diabetes. 1961;10(3):232–4. [Google Scholar] [PubMed]
15. Safdar A, Saleem A, Tarnopolsky MA. The potential of endurance exercise-derived exosomes to treat metabolic diseases. Nat Rev Endocrinol. 2016;12(9):504–17. doi:10.1038/nrendo.2016.76. [Google Scholar] [PubMed] [CrossRef]
16. Gutiérrez-Vázquez C, Villarroya-Beltri C, Mittelbrunn M, Sánchez-Madrid F. Transfer of extracellular vesicles during immune cell-cell interactions. Immunol Rev. 2013;251(1):125–42. doi:10.1111/imr.12013. [Google Scholar] [PubMed] [CrossRef]
17. Lauritzen HPMM, Brandauer J, Schjerling P, Koh HJ, Treebak JT, Hirshman MF, et al. Contraction and AICAR stimulate IL-6 vesicle depletion from skeletal muscle fibers in vivo. Diabetes. 2013;62(9):3081–92. [Google Scholar] [PubMed]
18. Huh JY. The role of exercise-induced myokines in regulating metabolism. Arch Pharm Res. 2018;41(1):14–29. doi:10.1007/s12272-017-0994-y. [Google Scholar] [PubMed] [CrossRef]
19. Obradovic M, Sudar-Milovanovic E, Soskic S, Essack M, Arya S, Stewart AJ, et al. Leptin and obesity: role and clinical implication. Front Endocrinol. 2021;12:1–14. doi:10.3389/fendo.2021.585887. [Google Scholar] [PubMed] [CrossRef]
20. Hoffmann C, Weigert C. Skeletal muscle as an endocrine organ: the role of myokines in exercise adaptations. Cold Spring Harb Perspect Med. 2017;7(11):a029793. doi:10.1101/cshperspect.a029793. [Google Scholar] [PubMed] [CrossRef]
21. Laurens C, Bergouignan A, Moro C. Exercise-released myokines in the control of energy metabolism. Front Physiol. 2020;11:1–8. doi:10.3389/fphys.2020.00091. [Google Scholar] [PubMed] [CrossRef]
22. Kjøbsted R, Hingst JR, Fentz J, Foretz M, Sanz M, Pehmøller C, et al. AMPK in skeletal muscle function and metabolism. FASEB J. 2018;32(4):1741–77. doi:10.1096/fj.201700442R. [Google Scholar] [PubMed] [CrossRef]
23. Trovato E, di Felice V, Barone R. Extracellular vesicles: delivery vehicles of myokines. Front Physiol. 2019;10:1–13. doi:10.3389/fphys.2019.00522. [Google Scholar] [PubMed] [CrossRef]
24. Bansal N, Kotwal N, Kumar S. Aerobic vs resistance exercise—an endocrine perspective. J Med Acad. 2020;3(1):7–10. doi:10.5005/jp-journals-10070-0057. [Google Scholar] [CrossRef]
25. Ruiz-Casado A, Martín-Ruiz A, Pérez LM, Provencio M, Fiuza-Luces C, Lucia A. Exercise and the hallmarks of cancer. Trends Cancer. 2017;3(6):423–41. doi:10.1016/j.trecan.2017.04.007. [Google Scholar] [PubMed] [CrossRef]
26. Forouzanfar MH, Afshin A, Alexander LT, Anderson HR, Bhutta ZA, Biryukov S, et al. Global, regional, and national comparative risk assessment of 79 behavioural, environmental and occupational, and metabolic risks or clusters of risks, 1990–2015: a systematic analysis for the global burden of disease study 2015. Lancet. 2016;388(10053):1659–724. [Google Scholar]
27. Leal LG, Lopes MA, Batista ML. Physical exercise-induced myokines and muscle-adipose tissue crosstalk: a review of current knowledge and the implications for health and metabolic diseases. Front Physiol. 2018;9:1–17. doi:10.3389/fphys.2018.01307. [Google Scholar] [PubMed] [CrossRef]
28. Santiago JA, Potashkin JA. Physical activity and lifestyle modifications in the treatment of neurodegenerative diseases. Front Aging Neurosci. 2023;15:1185671. doi:10.3389/fnagi.2023.1185671. [Google Scholar] [PubMed] [CrossRef]
29. Kwon JH, Moon KM, Min KW. Exercise-induced myokines can explain the importance of physical activity in the elderly: an overview. Healthc. 2020;8(4):378. [Google Scholar]
30. Lum D, Barbosa TM. Brief review: effects of isometric strength training on strength and dynamic performance. Int J Sports Med. 2019;40(6):363–75. doi:10.1055/a-0863-4539. [Google Scholar] [PubMed] [CrossRef]
31. Awtry EH, Balady GJ. Exercise and the heart. In: Cardiology secrets. 3rd ed. Elsevier; 2014. p. 323–8. doi:10.1016/B978-1-4557-4815-0.00044-1. [Google Scholar] [CrossRef]
32. Fletcher GF, Ades PA, Kligfield P, Arena R, Balady GJ, Bittner VA, et al. Exercise standards for testing and training. Circ. 2013;128(8):873–934. doi:10.1161/CIR.0b013e31829b5b44. [Google Scholar] [PubMed] [CrossRef]
33. Wiecek M, Szymura J, Maciejczyk M, Kantorowicz M, Szygula Z. Acute Anaerobic exercise affects the secretion of asprosin, irisin, and other cytokines—a comparison between sexes. Front Physiol. 2018;9:1–12. doi:10.3389/fphys.2018.01782. [Google Scholar] [PubMed] [CrossRef]
34. He Z, Tian Y, Valenzuela PL, Huang C, Zhao J, Hong P, et al. Myokine/adipokine response to aerobic exercise: is it just a matter of exercise load? Front Physiol. 2019;10:1–9. doi:10.3389/fphys.2019.00691. [Google Scholar] [PubMed] [CrossRef]
35. Zunner BEM, Wachsmuth NB, Eckstein ML, Scherl L, Schierbauer JR, Haupt S, et al. Myokines and resistance training: a narrative review. Int J Mol Sci. 2022;23(7):3501. [Google Scholar] [PubMed]
36. Tsai CL, Ukropec J, Ukropcová B, Pai MC. An acute bout of aerobic or strength exercise specifically modifies circulating exerkine levels and neurocognitive functions in elderly individuals with mild cognitive impairment. NeuroImage Clin. 2018;17:272–84. doi:10.1016/j.nicl.2017.10.028. [Google Scholar] [PubMed] [CrossRef]
37. Delezie J, Handschin C. Endocrine crosstalk between skeletal muscle and the brain. Front Neurol. 2018;9:698. doi:10.3389/fneur.2018.00698. [Google Scholar] [PubMed] [CrossRef]
38. Cotman CW, Berchtold NC, Christie LA. Exercise builds brain health: key roles of growth factor cascades and inflammation. Trends Neurosci. 2007;30(9):464–72. [Google Scholar] [PubMed]
39. Mattson MP. Energy intake and exercise as determinants of brain health and vulnerability to injury and disease. Cell Metab. 2012;16(6):706–22. doi:10.1016/j.cmet.2012.08.012. [Google Scholar] [PubMed] [CrossRef]
40. Cassilhas RC, Tufik S, de Mello MT. Physical exercise, neuroplasticity, spatial learning and memory. Cell Mol Life Sci. 2016;73(5):975–83. doi:10.1007/s00018-015-2102-0. [Google Scholar] [PubMed] [CrossRef]
41. Pedersen BK. Physical activity and muscle-brain crosstalk. Nat Rev Endocrinol. 2019;15(7):383–92. doi:10.1038/s41574-019-0174-x. [Google Scholar] [PubMed] [CrossRef]
42. Gregory S, Parker B, Thompson P. Physical activity, cognitive function, and brain health: what is the role of exercise training in the prevention of dementia? Brain Sci. 2012;2(4):684–708. [Google Scholar] [PubMed]
43. Batouli SAH, Saba V. At least eighty percent of brain grey matter is modifiable by physical activity: a review study. Behav Brain Res. 2017;332:204–17. doi:10.1016/j.bbr.2017.06.002. [Google Scholar] [PubMed] [CrossRef]
44. Kobilo T, Liu QR, Gandhi K, Mughal M, Shaham Y, van Praag H. Running is the neurogenic and neurotrophic stimulus in environmental enrichment. Learn Mem. 2011;18(9):605–9. doi:10.1101/lm.2283011. [Google Scholar] [PubMed] [CrossRef]
45. Mattson MP, Maudsley S, Martin B. BDNF and 5-HT: a dynamic duo in age-related neuronal plasticity and neurodegenerative disorders. Trends Neurosci. 2004;27(10):589–94. [Google Scholar] [PubMed]
46. Severinsen MCK, Pedersen BK. Muscle–organ crosstalk: the emerging roles of myokines. Endocr Rev. 2020;41(4):594–609. [Google Scholar] [PubMed]
47. DAniele Bobermin L, Scopel Medeiros L, Weber F, Tomazzoni De Oliveira G, Santi L, Orlando Beys-da-silva W, et al. Impact of SARS-CoV-2 infection during pregnancy on postnatal brain development: the potential role of glial cells. BIOCELL. 2022;46(12):2517–23. doi:10.32604/biocell.2022.021566. [Google Scholar] [CrossRef]
48. Małkiewicz MA, Szarmach A, Sabisz A, Cubała WJ, Szurowska E, Winklewski PJ. Blood-brain barrier permeability and physical exercise. J Neuroinflammation. 2019;16(1):15. doi:10.1186/s12974-019-1403-x. [Google Scholar] [PubMed] [CrossRef]
49. Harilal S, Jose J, Parambi DGT, Kumar R, Unnikrishnan MK, Uddin MS, et al. Revisiting the blood-brain barrier: a hard nut to crack in the transportation of drug molecules. Brain Res Bull. 2020;160(1–2):121–40. doi:10.1016/j.brainresbull.2020.03.018. [Google Scholar] [PubMed] [CrossRef]
50. Sujkowski A, Hong L, Wessells RJ, Todi SV. The protective role of exercise against age-related neurodegeneration. Ageing Res Rev. 2022;74:101543. [Google Scholar] [PubMed]
51. Crotty GF, Schwarzschild MA. Chasing protection in Parkinson’s disease: does exercise reduce risk and progression? Front Aging Neurosci. 2020;12:1–11. doi:10.3389/fnagi.2020.00186. [Google Scholar] [PubMed] [CrossRef]
52. Mahalakshmi B, Maurya N, Lee SD, Bharath Kumar V. Possible neuroprotective mechanisms of physical exercise in neurodegeneration. Int J Mol Sci. 2020;21(16):5895. [Google Scholar] [PubMed]
53. Zigmond MJ, Cameron JL, Leak RK, Mirnics K, Russell VA, Smeyne RJ, et al. Triggering endogenous neuroprotective processes through exercise in models of dopamine deficiency. Parkinsonism Relat Disord. 2009;15(SUPPL. 3):S42–5. doi:10.1016/S1353-8020(09)70778-3. [Google Scholar] [PubMed] [CrossRef]
54. Bastioli G, Arnold JC, Mancini M, Mar AC, Gamallo-Lana B, Saadipour K, et al. Voluntary exercise boosts striatal dopamine release: evidence for the necessary and sufficient role of BDNF. J Neurosci. 2022;42(23):4725–36. doi:10.1523/JNEUROSCI.2273-21.2022. [Google Scholar] [PubMed] [CrossRef]
55. Wu SY, Wang TF, Yu L, Jen CJ, Chuang JI, Wu F, et al. Running exercise protects the substantia nigra dopaminergic neurons against inflammation-induced degeneration via the activation of BDNF signaling pathway. Brain Behav Immun. 2011;25(1):135–46. doi:10.1016/j.bbi.2010.09.006. [Google Scholar] [PubMed] [CrossRef]
56. Palasz E, Wysocka A, Gasiorowska A, Chalimoniuk M, Niewiadomski W, Niewiadomska G. BDNF as a promising therapeutic agent in Parkinson’s disease. Int J Mol Sci. 2020;21(3):1170. [Google Scholar] [PubMed]
57. Singh M, Kakkar A, Sharma R, Kharbanda OP, Monga N, Kumar M, et al. Synergistic effect of BDNF and FGF2 in efficient generation of functional dopaminergic neurons from human mesenchymal stem cells. Sci Rep. 2017;7(1):1–13. doi:10.1038/s41598-017-11028-z. [Google Scholar] [PubMed] [CrossRef]
58. Er S, Airavaara M. Protective mechanisms by glial cell line-derived neurotrophic factor and cerebral dopamine neurotrophic factor against the α-synuclein accumulation in Parkinson’s disease. Biochem Soc Trans. 2023;51(1):245–57. [Google Scholar] [PubMed]
59. Calabresi P, Mechelli A, Natale G, Volpicelli-Daley L, Di Lazzaro G, Ghiglieri V. Alpha-synuclein in Parkinson’s disease and other synucleinopathies: from overt neurodegeneration back to early synaptic dysfunction. Cell Death Dis. 2023;14(3):176. [Google Scholar] [PubMed]
60. Lindahl M, Chalazonitis A, Palm E, Pakarinen E, Danilova T, Pham TD, et al. Cerebral dopamine neurotrophic factor-deficiency leads to degeneration of enteric neurons and altered brain dopamine neuronal function in mice. Neurobiol Dis. 2020;134:104696. [Google Scholar] [PubMed]
61. Lindholm P, Saarma M. Cerebral dopamine neurotrophic factor protects and repairs dopamine neurons by novel mechanism. Mol Psychiatry. 2022;27(3):1310–21. [Google Scholar] [PubMed]
62. Shirvani H, Aslani J, Fallah Mohammadi Z, Arabzadeh E. Short-term effect of low-, moderate-, and high-intensity exercise training on cerebral dopamine neurotrophic factor (CDNF) and oxidative stress biomarkers in brain male Wistar rats. Comp Clin Path. 2019;28(2):369–76. doi:10.1007/s00580-018-2885-0. [Google Scholar] [CrossRef]
63. da Silva WAB, Ferreira Oliveira K, Caroline Vitorino L, Ferreira Romão L, Allodi S, Lourenço Correa C. Physical exercise increases the production of tyrosine hydroxylase and CDNF in the spinal cord of a Parkinson’s disease mouse model. Neurosci Lett. 2021;760:136089. [Google Scholar] [PubMed]
64. Pesce M, La Fratta I, Paolucci T, Grilli A, Patruno A, Agostini F, et al. From exercise to cognitive performance: role of irisin. Appl Sci. 2021;11(15):7120. [Google Scholar]
65. Sánchez B, Muñoz-Pinto MF, Cano M. Irisin enhances longevity by boosting SIRT1, AMPK, autophagy and telomerase. Expert Rev Mol Med. 2023;25:e4. [Google Scholar]
66. Wrann CD, White JP, Salogiannnis J, Laznik-Bogoslavski D, Wu J, Ma D, et al. Exercise induces hippocampal BDNF through a PGC-1α/FNDC5 pathway. Cell Metab. 2013;18(5):649–59. [Google Scholar] [PubMed]
67. Natalicchio A, Marrano N, Biondi G, Dipaola L, Spagnuolo R, Cignarelli A, et al. Irisin increases the expression of anorexigenic and neurotrophic genes in mouse brain. Diabetes Metab Res Rev. 2020;36(3):1–7. doi:10.1002/dmrr.3238. [Google Scholar] [PubMed] [CrossRef]
68. Jodeiri Farshbaf M, Ghaedi K, Megraw TL, Curtiss J, Shirani Faradonbeh M, Vaziri P, et al. Does PGC1α/FNDC5/BDNF elicit the beneficial effects of exercise on neurodegenerative disorders? Neuro Molecular Med. 2016;18(1):1–15. doi:10.1007/s12017-015-8370-x. [Google Scholar] [PubMed] [CrossRef]
69. Miller KM, Mercado NM, Sortwell CE. Synucleinopathy-associated pathogenesis in Parkinson’s disease and the potential for brain-derived neurotrophic factor. npj Park Dis. 2021;7(1):35. doi:10.1038/s41531-021-00179-6. [Google Scholar] [PubMed] [CrossRef]
70. Ribeiro FF, Xapelli S. Intervention of brain-derived neurotrophic factor and other neurotrophins in adult neurogenesis. Adv Exp Med Biol. 2021;95–115. doi:10.1007/978-3-030-74046-7_8. [Google Scholar] [PubMed] [CrossRef]
71. Bathina S, Das UN. Brain-derived neurotrophic factor and its clinical implications. Arch Med Sci. 2015;6(6):1164–78. doi:10.5114/aoms.2015.56342. [Google Scholar] [PubMed] [CrossRef]
72. Schnyder S, Handschin C. Skeletal muscle as an endocrine organ: PGC-1α, myokines and exercise. Bone. 2015;80:115–25. doi:10.1016/j.bone.2015.02.008. [Google Scholar] [PubMed] [CrossRef]
73. Ohira K, Hayashi M. A new aspect of the TrkB signaling pathway in neural plasticity. Curr Neuropharmacol. 2009;7(4):276–85. [Google Scholar] [PubMed]
74. Autry AE, Monteggia LM. Brain-derived neurotrophic factor and neuropsychiatric disorders. Pharmacol Rev. 2012;64(2):238–58. doi:10.1124/pr.111.005108. [Google Scholar] [PubMed] [CrossRef]
75. Jin. Regulation of BDNF-TrkB signaling and potential therapeutic strategies for Parkinson’s disease. J Clin Med. 2020;9(1):257. [Google Scholar]
76. Finkbeiner S, Tavazoie SF, Maloratsky A, Jacobs KM, Harris KM, Greenberg ME. CREB: a major mediator of neuronal neurotrophin responses. Neuron. 1997;19(5):1031–47. [Google Scholar] [PubMed]
77. Li J, Wang Z, Li C, Song Y, Wang Y, Bo H, et al. Impact of exercise and aging on mitochondrial homeostasis in skeletal muscle: roles of ROS and epigenetics. Cells. 2022;11(13):2086. [Google Scholar] [PubMed]
78. Belviranlı M, Okudan N. Exercise training protects against aging-induced cognitive dysfunction via activation of the hippocampal PGC-1α/FNDC5/BDNF pathway. Neuro Molecular Med. 2018;20(3):386–400. doi:10.1007/s12017-018-8500-3. [Google Scholar] [PubMed] [CrossRef]
79. Elia A, Cannavo A, Gambino G, Cimini M, Ferrara N, Kishore R, et al. Aging is associated with cardiac autonomic nerve fiber depletion and reduced cardiac and circulating BDNF levels. J Geriatr Cardiol. 2021;18(7):549–59. doi:10.11909/j.issn.1671-5411.2021.07.009. [Google Scholar] [PubMed] [CrossRef]
80. Tomlinson CL, Herd CP, Clarke CE, Meek C, Patel S, Stowe R, et al. Physiotherapy for Parkinson’s disease: a comparison of techniques. Cochrane Database Syst Rev. 2014;2014(6):1–119. doi:10.1002/14651858. [Google Scholar] [CrossRef]
81. van der Kolk NM, King LA. Effects of exercise on mobility in people with Parkinson’s disease. Mov Disord. 2013;28(11):1587–96. doi:10.1002/mds.25658. [Google Scholar] [PubMed] [CrossRef]
82. Vivar C, Peterson BD, van Praag H. Running rewires the neuronal network of adult-born dentate granule cells. Neuroimage. 2016;131(1):29–41. doi:10.1016/j.neuroimage.2015.11.031. [Google Scholar] [PubMed] [CrossRef]
83. Canning CG, Sherrington C, Lord SR, Close JCT, Heritier S, Heller GZ, et al. Exercise for falls prevention in Parkinson disease: a randomized controlled trial. Neurology. 2015;84(3):304–12. [Google Scholar] [PubMed]
84. Uc EY, Doerschug KC, Magnotta V, Dawson JD, Thomsen TR, Kline JN, et al. Phase I/II randomized trial of aerobic exercise in Parkinson disease in a community setting. Neurology. 2014;83(5):413–25. doi:10.1212/WNL.0000000000000644. [Google Scholar] [PubMed] [CrossRef]
85. Tsang WWN. Tai Chi training is effective in reducing balance impairments and falls in patients with Parkinson’s disease. J Physiother. 2013;59(1):55. doi:10.1016/S1836-9553(13)70148-6. [Google Scholar] [PubMed] [CrossRef]
86. Okun MS. Deep-brain stimulation for Parkinson’s disease. N Engl J Med. 2012;367(16):1529–38. doi:10.1056/NEJMct1208070. [Google Scholar] [PubMed] [CrossRef]
87. Boel JA, Odekerken VJJ, Schmand BA, Geurtsen GJ, Cath DC, Figee M, et al. Cognitive and psychiatric outcome 3 years after globus pallidus pars interna or subthalamic nucleus deep brain stimulation for Parkinson’s disease. Park Relat Disord. 2016;33(Suppl 14):90–5. doi:10.1016/j.parkreldis.2016.09.018. [Google Scholar] [PubMed] [CrossRef]
88. Morgante L, Morgante F, Moro E, Epifanio A, Girlanda P, Ragonese P, et al. How many parkinsonian patients are suitable candidates for deep brain stimulation of subthalamic nucleus? Results of a questionnaire. Parkinsonism Relat Disord. 2007;13(8):528–31. [Google Scholar] [PubMed]
89. González-García N, Armony JL, Soto J, Trejo D, Alegría MA, Drucker-Colín R. Effects of rTMS on Parkinson’s disease: a longitudinal fMRI study. J Neurol. 2011;258(7):1268–80. doi:10.1007/s00415-011-5923-2. [Google Scholar] [PubMed] [CrossRef]
90. VonLoh M, Chen R, Kluger B. Safety of transcranial magnetic stimulation in Parkinson’s disease: a review of the literature. Park Relat Disord. 2013;19(6):573–85. doi:10.1016/j.parkreldis.2013.01.007. [Google Scholar] [PubMed] [CrossRef]
91. Reinhart F, El Massri N, Chabrol C, Cretallaz C, Johnstone DM, Torres N, et al. Intracranial application of near-infrared light in a hemi-parkinsonian rat model: the impact on behavior and cell survival. J Neurosurg. 2016;124(6):1829–41. [Google Scholar] [PubMed]
92. Pedersen BK, Steensberg A, Fischer C, Keller C, Keller P, Plomgaard P, et al. The metabolic role of IL-6 produced during exercise: is IL-6 an exercise factor? Proc Nutr Soc. 2004;63(2):263–7. [Google Scholar] [PubMed]
93. Petersen AMW, Pedersen BK. The anti-inflammatory effect of exercise. J Appl Physiol. 2005;98(4):1154–62. doi:10.1152/japplphysiol.00164.2004. [Google Scholar] [PubMed] [CrossRef]
94. Liu PZ, Nusslock R. Exercise-mediated neurogenesis in the hippocampus via BDNF. Front Neurosci. 2018;12:1–6. doi:10.3389/fnins.2018.00052. [Google Scholar] [PubMed] [CrossRef]
95. Chow LS, Gerszten RE, Taylor JM, Pedersen BK, van Praag H, Trappe S, et al. Exerkines in health, resilience and disease. Nat Rev Endocrinol. 2022;18(5):273–89. [Google Scholar] [PubMed]
96. Fabel K, Fabel K, Tam B, Kaufer D, Baiker A, Simmons N, et al. VEGF is necessary for exercise-induced adult hippocampal neurogenesis. Eur J Neurosci. 2003;18(10):2803–12. doi:10.1111/j.1460-9568.2003.03041.x. [Google Scholar] [PubMed] [CrossRef]
97. Kraus RM, Stallings HW, Yeager RC, Gavin TP. Circulating plasma VEGF response to exercise in sedentary and endurance-trained men. J Appl Physiol. 2004;96(4):1445–50. [Google Scholar] [PubMed]
98. D’Souza RF, Markworth JF, Aasen KMM, Zeng N, Cameron-Smith D, Mitchell CJ. Acute resistance exercise modulates microRNA expression profiles: combined tissue and circulatory targeted analyses. PLoS One. 2017;12(7):e0181594. doi:10.1371/journal.pone.0181594. [Google Scholar] [PubMed] [CrossRef]
99. Russell AP, Lamon S, Boon H, Wada S, Güller I, Brown EL, et al. Regulation of miRNAs in human skeletal muscle following acute endurance exercise and short-term endurance training. J Physiol. 2013;591(18):4637–53. doi:10.1113/jphysiol.2013.255695. [Google Scholar] [PubMed] [CrossRef]
100. Li G, Liu H, Ma C, Chen Y, Wang J, Yang Y. Exosomes are the novel players involved in the beneficial effects of exercise on type 2 diabetes. J Cell Physiol. 2019;234(9):14896–905. doi:10.1002/jcp.28319. [Google Scholar] [PubMed] [CrossRef]
101. Lou J, Wu J, Feng M, Dang X, Wu G, Yang H, et al. Exercise promotes angiogenesis by enhancing endothelial cell fatty acid utilization via liver-derived extracellular vesicle miR-122-5p. J Sport Heal Sci. 2022;11(4):495–508. [Google Scholar] [PubMed]
102. Chaturvedi P, Kalani A, Medina I, Familtseva A, Tyagi SC. Cardiosome mediated regulation of MMP9 in diabetic heart: role of mir29b and mir455 in exercise. J Cell Mol Med. 2015;19(9):2153–61. doi:10.1111/jcmm.12589. [Google Scholar] [PubMed] [CrossRef]
103. Vinel C, Lukjanenko L, Batut A, Deleruyelle S, Pradère JP, Le Gonidec S, et al. The exerkine apelin reverses age-associated sarcopenia. Nat Med. 2018;24(9):1360–71. [Google Scholar] [PubMed]
104. Daskalopoulou SS, Cooke AB, Gomez YH, Mutter AF, Filippaios A, Mesfum ET, et al. Plasma irisin levels progressively increase in response to increasing exercise workloads in young, healthy, active subjects. Eur J Endocrinol. 2014;171(3):343–52. [Google Scholar] [PubMed]
105. Martinez Munoz IY, del Camarillo Romero ES, de Garduno Garcia JJ. Irisin a novel metabolic biomarker: present knowledge and future directions. Int J Endocrinol. 2018;2018:1–8. [Google Scholar]
106. Zhu J, Dou S, Jiang Y, Chen J, Wang C, Cheng B. Apelin-13 protects dopaminergic neurons in MPTP-induced Parkinson’s disease model mice through inhibiting endoplasmic reticulum stress and promoting autophagy. Brain Res. 2019;1715(917–931):203–12. doi:10.1016/j.brainres.2019.03.027. [Google Scholar] [PubMed] [CrossRef]
107. Pouresmaeili-Babaki E, Esmaeili-Mahani S, Abbasnejad M, Ravan H. Protective effect of neuropeptide apelin-13 on 6-hydroxydopamine-induced neurotoxicity in SH-SY5Y dopaminergic cells: involvement of its antioxidant and antiapoptotic properties. Rejuvenation Res. 2018;21(2):162–7. doi:10.1089/rej.2017.1951. [Google Scholar] [PubMed] [CrossRef]
108. Chen P, Wang Y, Chen L, Song N, Xie J. Apelin-13 protects dopaminergic neurons against rotenone—induced neurotoxicity through the AMPK/mTOR/ULK-1 mediated autophagy activation. Int J Mol Sci. 2020;21(21):8376. [Google Scholar] [PubMed]
109. Hwang J, Estick CM, Ikonne US, Butler D, Pait MC, Elliott LH, et al. The role of lysosomes in a broad disease-modifying approach evaluated across transgenic mouse models of Alzheimer’s disease and Parkinson’s disease and models of mild cognitive impairment. Int J Mol Sci. 2019;20(18):4432. [Google Scholar] [PubMed]
110. Lee B, Shin M, Park Y, Won SY, Cho KS. Physical exercise-induced myokines in neurodegenerative diseases. Int J Mol Sci. 2021;22(11):5795. [Google Scholar] [PubMed]
111. Moon HY, Becke A, Berron D, Becker B, Sah N, Benoni G, et al. Running-induced systemic cathepsin B secretion is associated with memory function. Cell Metab. 2016;24(2):332–40. doi:10.1016/j.cmet.2016.05.025. [Google Scholar] [PubMed] [CrossRef]
112. Carro E, Nuñez A, Busiguina S, Torres-Aleman I. Circulating insulin-like growth factor I mediates effects of exercise on the brain. J Neurosci. 2000;20(8):2926–33. doi:10.1523/JNEUROSCI.20-08-02926.2000. [Google Scholar] [PubMed] [CrossRef]
113. Quesada A, Lee BY, Micevych PE. PI3 kinase/Akt activation mediates estrogen and IGF-1 nigral DA neuronal neuroprotection against a unilateral rat model of Parkinson’s disease. Dev Neurobiol. 2008;68(5):632–44. doi:10.1002/dneu.20609. [Google Scholar] [PubMed] [CrossRef]
114. Fang X, Ma J, Mu D, Li B, Lian B, Sun C. FGF21 protects dopaminergic neurons in Parkinson’s disease models via repression of neuroinflammation. Neurotox Res. 2020;37(3):616–27. doi:10.1007/s12640-019-00151-6. [Google Scholar] [PubMed] [CrossRef]
115. Kalivendi SV, Kotamraju S, Cunningham S, Shang T, Hillard CJ, Kalyanaraman B. 1-Methyl-4-phenylpyridinium (MPP+)-induced apoptosis and mitochondrial oxidant generation: role of transferrin-receptor-dependent iron and hydrogen peroxide. Biochem J. 2003;371(1):151–64. [Google Scholar] [PubMed]
116. Subbotina E, Sierra A, Zhu Z, Gao Z, Koganti SRK, Reyes S, et al. Musclin is an activity-stimulated myokine that enhances physical endurance. Proc Natl Acad Sci. 2015;112(52):16042–7. doi:10.1073/pnas.1514250112. [Google Scholar] [PubMed] [CrossRef]
117. Rodrigues TM, Jerónimo-Santos A, Outeiro TF, Sebastião AM, Diógenes MJ. Challenges and promises in the development of neurotrophic factor-based therapies for Parkinson’s disease. Drugs Aging. 2014;31(4):239–61. doi:10.1007/s40266-014-0160-x. [Google Scholar] [PubMed] [CrossRef]
118. Sullivan AM, Toulouse A. Neurotrophic factors for the treatment of Parkinson’s disease. Cytokine Growth Factor Rev. 2011;22(3):157–65. [Google Scholar] [PubMed]
119. Li S, Dong J, Cheng C, Le W. Therapies for Parkinson’s diseases: alternatives to current pharmacological interventions. J Neural Transm. 2016;123(11):1279–99. doi:10.1007/s00702-016-1603-9. [Google Scholar] [PubMed] [CrossRef]
120. Horowitz AM, Fan X, Bieri G, Smith LK, Sanchez-Diaz CI, Schroer AB, et al. Blood factors transfer beneficial effects of exercise on neurogenesis and cognition to the aged brain. Science. 2020;369(6500):167–73. doi:10.1126/science.aaw2622. [Google Scholar] [PubMed] [CrossRef]
121. Ellis T, Boudreau JK, DeAngelis TR, Brown LE, Cavanaugh JT, Earhart GM, et al. Barriers to exercise in people with Parkinson disease. Phys Ther. 2013;93(5):628–36. [Google Scholar] [PubMed]
122. Frazzitta G, Maestri R, Ghilardi MF, Riboldazzi G, Perini M, Bertotti G, et al. Intensive rehabilitation increases BDNF serum levels in Parkinsonian patients. Neurorehabil Neural Repair. 2014;28(2):163–8. doi:10.1177/1545968313508474. [Google Scholar] [PubMed] [CrossRef]
123. O’Callaghan A, Harvey M, Houghton D, Gray WK, Weston KL, Oates LL, et al. Comparing the influence of exercise intensity on brain-derived neurotrophic factor serum levels in people with Parkinson’s disease: a pilot study. Aging Clin Exp Res. 2020;32(9):1731–8. doi:10.1007/s40520-019-01353-w. [Google Scholar] [PubMed] [CrossRef]
124. Rafie F, Rajizadeh MA, Shahbazi M, Pourranjbar M, Nekouei AH, Sheibani V, et al. Effects of voluntary, and forced exercises on neurotrophic factors and cognitive function in animal models of Parkinson’s disease. Neuropeptides. 2023;101:102357. [Google Scholar] [PubMed]
125. Cefis M, Prigent-Tessier A, Quirié A, Pernet N, Marie C, Garnier P. The effect of exercise on memory and BDNF signaling is dependent on intensity. Brain Struct Funct. 2019;224(6):1975–85. doi:10.1007/s00429-019-01889-7. [Google Scholar] [PubMed] [CrossRef]
126. Marino G, Campanelli F, Natale G, De Carluccio M, Servillo F, Ferrari E, et al. Intensive exercise ameliorates motor and cognitive symptoms in experimental Parkinson’s disease restoring striatal synaptic plasticity. Sci Adv. 2023;9(28):eadh1403. [Google Scholar] [PubMed]
127. Matthews VB, Åström MB, Chan MHS, Bruce CR, Krabbe KS, Prelovsek O, et al. Brain-derived neurotrophic factor is produced by skeletal muscle cells in response to contraction and enhances fat oxidation via activation of AMP-activated protein kinase. Diabetologia. 2009;52(7):1409–18. doi:10.1007/s00125-009-1364-1. [Google Scholar] [PubMed] [CrossRef]
128. Park BR, Hwang JH, Kim MS, Lee MY, Rhee JK, Lee SH. Modulation of BDNF expression by electrical stimulation in hindlimb muscles of rats. Neurosci Res Commun. 2004;34(1):10–9. doi:10.1002/nrc.10101. [Google Scholar] [CrossRef]
129. Sanchis-Gomar F, Lopez-Lopez S, Romero-Morales C, Maffulli N, Lippi G, Pareja-Galeano H. Neuromuscular electrical stimulation: a new therapeutic option for chronic diseases based on contraction-induced myokine secretion. Front Physiol. 2019;10:1463. doi:10.3389/fphys.2019.01463. [Google Scholar] [PubMed] [CrossRef]
Cite This Article
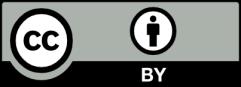
This work is licensed under a Creative Commons Attribution 4.0 International License , which permits unrestricted use, distribution, and reproduction in any medium, provided the original work is properly cited.