Open Access
REVIEW
Therapeutic and regenerative potential of different sources of mesenchymal stem cells for cardiovascular diseases
Department of Basic Medical Sciences, Faculty of Medicine, Yarmouk University, Irbid, Jordan
* Corresponding Author: EJLAL ABU-EL-RUB. Email:
(This article belongs to the Special Issue: Perspectives on Stem Cells and Regenerative Medicine)
BIOCELL 2024, 48(4), 559-569. https://doi.org/10.32604/biocell.2024.048056
Received 26 November 2023; Accepted 16 January 2024; Issue published 09 April 2024
Abstract
Mesenchymal stem cells (MSCs) are ideal candidates for treating many cardiovascular diseases. MSCs can modify the internal cardiac microenvironment to facilitate their immunomodulatory and differentiation abilities, which are essential to restore heart function. MSCs can be easily isolated from different sources, including bone marrow, adipose tissues, umbilical cord, and dental pulp. MSCs from various sources differ in their regenerative and therapeutic abilities for cardiovascular disorders. In this review, we will summarize the therapeutic potential of each MSC source for heart diseases and highlight the possible molecular mechanisms of each source to restore cardiac function.Keywords
Abbreviations and Acronyms
2-DC | 2′-deoxycytidine |
α-SA | α-sarcomeric actinin |
Ad-GSK-3 | GSK-3-containing adenovirus |
AD-MSCs | Adipose tissue–derived mesenchymal stem cells |
ADR-β2 | Adrenergic Receptor β2 |
Akt | Protein Kinase B |
ANF | Atrial Natriuretic Factor |
ANP | Atrial Natriuretic Peptide |
Ang-1 | Angiopoietin-1 |
Ang-2 | Angiopoietin-2 |
ATP | Adenosine Triphosphate |
Bax | Bcl2 Associated X protein |
BM-MSCs | Bone marrow derived -Mesenchymal Stem Cells |
BMP4 | Bone morphogenetic protein 4 |
BNP | Brain Natriuretic Peptide bFGF: basic Fibroblast Growth Factor |
cTnC | Cardiac troponin C |
cTnI | Cardiac troponin I |
cTnT | Cardiac troponin T |
c-kit | Protein kinase Kit |
CVD | Cardiovascular diseases |
Cx43 | Connexin 43 |
DP-MSCs | Dental Pulp–derived Mesenchymal Stem Cells |
DPSCs | Dental Pulp Stem Cells |
EGF | Endothelial Growth Factor |
ErbB | Erythroblastic leukemia viral oncogene Homologue |
ERK | Extracellular-signal-Regulated Kinase |
FGF | Fibroblast Growth Factor |
Flk1 | Fetal liver kinase 1 |
Fstl1 | Follistatin-like 1 |
GATA4 | GATA binding protein 4 |
GATA6 | GATA binding protein 6 |
GSK | Glycogen Synthase Kinase |
H2O2 | Hydrogen peroxide |
HAND2 | Heart And Neural crest Derivative |
Has 1 | Hyaluronan Synthase 1 |
Has2 | Hyaluronan Synthase 2 |
HAT | histone acetyltransferase |
Hes1 | Hairy and enhancer of split-1 |
HGF | Hepatocyte Growth Factor |
HIF-1α | Hypoxia-Inducible Factor-1α |
HMGB1 | High-Mobility Group Box-1 |
HO-1 | Heme oxygenase-1 |
ICA | Icariin |
IGF-1 | Insulin-like Growth Factor-1 |
IL | Interleukin |
I/R | Ischemic/Reperfusion |
KDR | Kinase insert domain receptor |
KLF4 | Kruppel-like factor 4 |
LAD | Left anterior descending artery |
LVEF | Left Ventricular Ejection Fraction |
MEF2C | Myocyte-specific enhancer factor 2c |
MHC | Major Histocompatibility Complex |
MI | Myocardial infarction |
MIF | Macrophage Inhibitory Factor |
miR | microRNA |
MLC | Myosin light chain |
MSCs | Mesenchymal Stem Cells |
MYH | Myosin heavy chain |
NRG1 | Neureugulin-1 |
NRVMs | Neonatal Rat Ventricular Myocytes |
OCT4 | Octamer-binding transcription factor 4 |
ox-LDL | Oxidized low-density lipoprotein |
p38MAPK | p38 mitogen-activated protein kinases |
PDGFR | Platelet derived growth factor receptor |
PGF | Placental growth factor |
PI3K | Phosphoinositide 3-Kinase |
qPCR | Quantitative polymerase chain reaction |
ROS | Reactive oxygen species |
Sal B | Salvianolic acid B |
Sca-1 | Stem cell antigen 1 |
SD | Sprague-Dawley |
SDF-1 | Stromal cell-derived factor 1 |
Serca2α | Sarcoendoplasmic reticulum ATPsase-2A |
SFRP2 | Secreted frizzled-related protein 2 |
SHEDs | Human exfoliated deciduous teeth |
SMA | Smooth muscle actin |
SMAD4 | Mothers against Decapentaplegic homolog 4 |
SOX2 | Sex-determining region Y box 2 |
TBX18 | T-box transcription factor 18 |
TGF-β | Transforming growth factor-β |
TNF | Tumor necrosis factor |
TNNT2 | Troponin T2 |
UC-MSCs | Umbilical cord–derived mesenchymal stem cells |
UTP | Uridine triphosphate |
vWF | Von willebrand factor |
VEGF | Vascular endothelial growth factor |
Cardiovascular diseases (CVD) are among the top five common causes of death worldwide. They require long-term treatment and can significantly impact the quality of life of patients. The most prevalent CVDs are stroke, arrhythmias, myocardial infarction (MI), and valvular diseases [1], which contribute to more than 610,000 deaths annually in the US alone [2]. The most noticeable pathophysiological finding in the majority of CVDs is the damage to myocardial cells and the subsequent changes in the internal microenvironment of the heart. Myocardial cells are well-known for their poor regenerative abilities; thus, they cannot survive intense injuries and stresses. CVDs are usually associated with deterioration in cardiac output and contractility that can exacerbate heart failure [3]. The current treatment guidelines are limited to symptom management using medications, while the surgical strategies to improve heart function are quite invasive and carry a high risk of serious complications. Stem cell transplantation has been considered the leap of hope to repair the damaged heart and restore its function, which is more effective, safer, and less invasive than surgical procedures and heart transplantation [4].
Mesenchymal stem cells (MSCs) are considered the ideal candidate for cardiac regeneration as they can be easily isolated, have multipotent characteristics, possess immune privileged properties, are ethically benign, and have a low risk of carcinogenesis and genotoxicity [3,4]. The ability of MSCs to reinvigorate the damaged heart has been corroborated by many in vitro, preclinical, and clinical studies. These studies explored MSCs isolated from multiple sources, including bone marrow, adipose tissue, umbilical cord, dental pulps, endometrial polyps, menstrual blood, etc. In this mini-review, we will discuss the cardiac regeneration potential of each type of MSCs and explain the molecular mechanisms of their cardiogenesis abilities in a concise and comprehensive way. This will contribute to developing productive approaches that could speed up the incorporation of MSCs in the treatment guidelines for heart diseases by translating the outcomes of these in vitro and preclinical studies in large scale animal studies and clinical trials [5,6].
Bone marrow-derived mesenchymal stem cells and cardiovascular regeneration
Bone marrow-derived mesenchymal stem cells (BM-MSCs) are the most studied stem cell type for regenerating damaged tissues. Many reports have demonstrated the therapeutic potential of BM-MSCs in restoring heart functions through different mechanisms. BM-MSCs can differentiate into cardiomyocytes or cardiomyocyte-like cells, playing a critical role in repairing damaged cardiac tissues by promoting angiogenesis, suppressing inflammation, and terminating fibrosis, which can improve the left ventricular ejection fraction (LVEF) [7]. Despite the positive outcomes of transplanting BM-MSCs in the diseased heart, their therapeutic efficiency has been hampered by poor survival, retention, and engraftment post-transplantation due to the harsh microenvironment that exists in many cardiac diseases. This is characterized by a limited blood supply, a lack of nutrient sources, and the accumulation of reactive oxygen species (ROS) [8]. Several approaches have been applied to improve the survival and therapeutic capabilities of BM-MSCs by modifying their characteristics using growth factors, combining different stem cells, and modulating their genetic composition. 5-Azacytidine, a demethylating agent, has been found to induce cardiomyogenic differentiation of BM-MSCs. The expression of cardiac muscle‑specific markers desmin and cardiac troponin‑T (cTnT), was detected after treating MSCs with a low dose of 5-azacytidine. However, the maintenance of 5-azacytidine-treated BM-MSCs beyond five weeks did not enhance the cardiogenicity of BM-MSCs; rather, it produced negative effects. In the eighth week, for example, 5‑azacytidine-treated BM-MSCs decreased in size, and the number of desmin and cTnT‑positive cells declined hugely [9]. A recent study co-cultured protein kinase kit (c-kit+) BM-MSCs with neonatal rat ventricular myocytes (NRVMs), and it was found that c-kit+ BM-MSCs that were in close contact with NRVMs induced the formation of gap junctions. This enhanced BM-MSCs electrophysiological properties by increasing the level of Connexin43 (Cx43) and increased their contractility upon β adrenergic stimulation [10]. The cardiomyogenic differentiation ability of c-Kit+ BM-MSCs was boosted when treated with L-carnitine [11]. L-carnitine is well-known as a potent free radical scavenger and a regulator of lipid metabolism and oxygen consumption in the heart. An Exogenous supplement of L-carnitine in the cell culture media of c-kit+ BM-MSCs was found to improve their survival, proliferation, and cardiac differentiation, as shown by the increased expression of cardiac markers and cytokines in the resulting cardiomyocytes. These included angiopoietin-1 (Ang-1), angiopoietin-2 (Ang-2), cardiac troponin-I (cTnI), von Willebrand factor (vWF), and smooth muscle actin (SMA), and a cocktail of cytokines, such as interleukin (IL)-6, insulin-like growth factor (IGF-1), transforming growth factor-β (TGF-β), and vascular endothelial growth factor (VEGF), which are important indicators of efficient cardiomyogenesis and angiogenesis [11].
It was demonstrated that the survival potential of transplanted MSCs in an ischemic heart can be reinforced by overexpressing some genes. Overexpression of IL-7 in BM-MSCs, for example, can potentiate their cardiac regeneration abilities. Co-culturing IL-7++ BM-MSCs with cardiomyocytes was found to increase the level of cardiac-specific proteins, mainly cTnT and α-actinin, with a subsequent enhancement in cardiac function parameters and a reduction of the fibrotic area [12].
Several studies showed that priming MSCs with growth factors promotes the survival of these implanted cells, particularly under hypoxic conditions. Overexpression of IGF-1 in BM-MSCs ameliorated hypoxia-induced apoptosis, and boosted their migration ability and protective effect on cardiomyoblasts by activating the phosphoinositide 3-kinase (PI3K)/AKT/secreted frizzled-related protein 2 (SFRP2)/β-catenin signaling pathway. The cardiac repairing abilities of IGF-1++ BM-MSCs were also observed in reduced infarct volume and modulated levels of anti-apoptotic and pro-apoptotic proteins [13]. Similarly, priming BM-MSCs with TGF-β1 increased the expression of sarcomeric actin, cTnT protein, transcription factors, α-major histocompatibility complex (α-MHC), GATA binding protein 4 (GATA4), and homeobox protein Nkx2-5 during their cardiomyogenic differentiation [14]. TGF-β1-induced BM-MSC cells effectively reduced scar area and myocyte apoptosis and improved myocardial function [14]. Moreover, preconditioning BM-MSCs with a combination of salvianolic acid B (Sal B) and TGF-β1 promoted cardiomyogenic differentiation more than each agent separately [15]. The cardiac regeneration capabilities of BM-MSCs were also promoted after the induction of hypoxia-inducible factor-1α (HIF-1α) overexpression using an adenovirus delivery system. Increasing the expression of HIF-1α in BM-MSCs modulated TGF-β1/mothers against decapentaplegic homolog 4(SMAD4) signaling and enhanced the differentiation of BM-MSCs into mature cardiomyocytes [16]. The combined use of BM-MSCs and heme oxygenase-1 (HO-1) significantly increased the expression of basic fibroblast growth factor (bFGF) and VEGF, which are important to increase angiogenesis. At the same time, there was a significant drop in the level of inflammatory cytokines; tumor necrosis factor (TNF)-α, IL-1β, and IL-6, and a rise in the level of the anti-inflammatory cytokine IL-10. Additionally, the pro-apoptotic protein Bcl2 associated X protein (Bax) was downregulated after incorporating HO-1 with BM-MSCs. These molecular changes were beneficial in reducing the infarct size, decreasing apoptosis, and increasing the ejection fraction [17]. Glycogen synthase kinase (GSK)-3β was also found to push up the therapeutic advantages of BM-MSCs. The overexpression of GSK-3β in BM-MSCs improved their cardiomyocyte differentiation potential, most likely by downregulating β-catenin, increasing capillary density, and stimulating the release of paracrine factors, specifically VEGF-A. VEGF-A elevation in BM-MSCs is partially responsible for improving left ventricular function. Moreover, the transduction of BM-MSCs with GSK-3-containing adenovirus (Ad-GSK-3) increased mRNA expression of cardiomyocyte-related markers, such as Nkx2.5, cardiac troponin C (cTnC), α-MHC, and atrial natriuretic factor (ANF) [18,19].
The most noticeable obstacles to using BM-MSCs for cardiac regeneration are their low differentiation efficiency in vivo and their poor survival after transplantation in an ischemic heart tissue [20]. Therefore, priming these cells with trophic factors and chemical reagents is required to enhance their cardiac differentiation capacity. A recent study found that oxidized low-density lipoprotein (ox-LDL)-receptor-1 is a potent activating stimulus of the extracellular-signal-regulated kinase (ERK) signaling pathway, required to promote BM-MSCs cardiomyogenic differentiation. Additionally, it was shown that major cardiac makers, such as α-MHC, β-MHC, atrial natriuretic peptide (ANP), brain natriuretic peptide (BNP), and α-cardiac actin, were significantly expressed in the cardiomyocytes derived from BM-MSCs treated with ox-LDL [21]. It has been hypothesized that the overexpression of CD51, also called integrin alpha V, in BM-MSCs may promote their migratory abilities to the infarcted heart. Additionally, transplanting CD51++ BM-MSC markedly improved the LVEF and restored cardiac functionality [22]. Transplanting BM-MSCs that overexpress platelet-derived growth factor receptor (PDGFR)-α in the peri-infarcted areas of the ischemic heart reversed the deterioration in left ventricular functions and the stimulation of remodeling machinery, induced angiogenesis, and reduced fibrosis in the infarcted heart. The recruitment and engraftment of PDGFRα++ BM-MSCs to damaged areas within the heart were enhanced by systemic administration of the high-mobility group box-1 (HMGB1) fragment [7]. Upregulating follistatin-like 1 (Fstl1) in BM-MSCs was found to increase their tolerance to hypoxia and to optimize their cardiac repair abilities during the ischemic phase of myocardial infarction. This was coupled with potentiating the phosphorylation of two major survival proteins; protein kinase B (Akt) at Ser473 and GSK3 at Ser9, thus activating the Akt/GSK-3 signaling required for cell death resistance. Further, injection of Fstl1++ BM-MSCs promoted cardiac neovascularization and reduced post-MI fibroblast recruitment, extracellular matrix (ECM) deposition, inflammatory cell infiltration, and scar size [20]. Interestingly, transplantation of a BM-MSC sub-population called stem cell antigen 1 (Sca-1) BM-MSCs rejuvenated the aged heart and reduced the symptoms of heart failure through the activation of the PDGFRβ-Akt/p27Kip1 signaling pathway [23].
BM-MSCs can also mediate their cardiac regeneration by secreting different microRNAs, which have critical regulatory functions in cardiac development and cardiomyocyte differentiation. A recent study found that miR-29c levels were significantly reduced after myocardial ischemic/reperfusion (I/R) injury, and BM-MSCs derived miR-29c can protect the heart from I/R injury and its associated oxidative stress by mitigating excessive autophagy through PTEN inhibition and AKT/mTOR signaling activation [24]. On the other hand, inhibiting certain microRNAs can improve the cardiomyogenic differentiation of BM-MSCs. For instance, it has been found that inhibition of miR-23 enhanced the ability of BM-MSCs to form functional cardiomyocytes via the activation of the Wnt signaling pathway, with the evidence of noticeable elevation of cardiomyocyte molecular markers including GATA4, Nkx2-5, MHC, and cTnI. Moreover, hyaluronan synthase 2 (Has2), which regulates stem cell differentiation and maintains metabolic homeostasis, was markedly upregulated in BM-MSCs treated with a miR-23 inhibitor [25].
These promising results highlighted the efforts of preclinical studies in treating BM-MSCs to make them capable of differentiation into cardiomyocytes by enhancing their migration ability, increasing angiogenesis and LVEF, and reducing infarct size should be confirmed by conducting large scale animal studies and clinical studies. Although BM-MSCs have proven their effectiveness in vivo and in vitro, some challenges and obstacles still exist. Therefore, great efforts should be made to enhance their differentiation potential and improve their survival rate after transplantation. The cardiac regeneration abilities of BM-MSCs have been summarized in Fig. 1.
Figure 1: Certain modifications applied to Bone marrow-derived mesenchymal stem cells enhanced their differentiation into cardiomyocytes. This was mediated by genetic modifications that can increase or decrease the expression of some proteins and markers. These genetic modifications targeted specific proteins that can increase the differentiation of BM-MSCs to cardiomyocytes, including ox-LDL receptor-1, Fstl1, CD-51, HMGB1, growth factors such as IL-7, TGF-β1, and IGF-1, C-kit, HIF-1α, Sca-1 antigen, GSK-3β, and HO-1. The chemical modifications achieved by using drugs, such as 5-azacytidine, were reported to increase the expression of cardiac-specific markers. Targeting specific miRNAs in BM-MSCs was also found to play a role in modulating their cardiac regeneration potential. These modulatory methods reprogrammed BM-MSCs to increase angiogenesis and LVEF and reduce the infarct size. c-kit+, protein kinase Kit+; Fstl1, Follistatin-like 1; GSK-3B, Glycogen Synthase Kinase-3B; HIF-1α, Hypoxia-Inducible Factor-1α; HO-1, Heme oxygenase-1; HMGB1, High-Mobility Group Box-1; IGF-1, Insulin-like Growth Factor-1; IL-7++, Interleukin-7++; LVEF, Left ventricular ejection fraction; miRNA, microRNA; MSCs, mesenchymal stem cells; ox-LDL receptor-1, Oxidized Low-Density Lipoprotein receptor-1; Sca-1, stem cell antigen 1; TGF-β: Transforming Growth Factor-β.
Adipose tissue-derived mesenchymal stem cells and cardiac regeneration
Adipose tissue-derived mesenchymal stem cells (AD-MSCs) are obtained by collagenase treatment of the lipoaspirates. These cells have similar multipotent differentiation capacity to BM-MSCs and umbilical cord-derived mesenchymal stem cells (UC-MSCs) [26]. In comparison to BM-MSCs and UC-MSCs, AD-MSCS express a wide range of adhesive proteins, including N-cadherin, VE-cadherin, cadherin-11, and fibronectin giving them better homing and adhesion properties at the host site [27].
AD-MSCs can be harvested from various sites; the subcutaneous region, which is the most accessible and common site for isolating AD-MSCs. AD-MSCs can be also collected from the visceral, omental, epicardial and perivascular regions. The different subtypes of AD-MSCs have comparable multipotency and immunomodulation characteristics. Some Studies have successfully applied these cells in various animal models of chronic MI. Subcutaneous AD-MSCs have been infused in acute MI and chronic heart failure models. These AD-MSCs were able to enhance angiogenesis by secreting paracrine factors that target neovascularization [28]. The use of visceral AD-MSCs in the animal models of MI showed significant improvements in the left ventricular end-diastolic volume, LVEF, and cardiac output. It also enhances the capillary density and Dopplar tissue perfusion scores. Visceral AD-MSCs have less adiponectin secretion rendering them highly susceptible to apoptosis and cell lysis under ischemic conditions [29]. Despite the comparable osteogenic and adipogenic differentiation abilities for the epicardial and omental AD-MSCs, epicardial AD-MSCs possessed superior cardiomyogenesis potential than any other AD-MSCs subtype. The higher cardiac regenerating ability of the epicardial AD-MSCs must be validated and optimized using large animal models of MI [30].
AD-MSCs can improve cardiac functions by differentiating into new cardiac cells or eliciting paracrine effects that can restore the internal microenvironment of the injured heart [31]. Many preclinical and clinical studies corroborated the cardiac regeneration potential of AD-MSCs. In the APOLLO trial, which used the AD-MSCs for MI treatment in humans and mainly focused on patients with ST elevation, it was found that the intracoronary infusion of AD-MSCs was able to improve the ejection fraction by reducing the infarct size after six months of transplantation [32]. Pre-treatment of AD-MSCs with 5-azacytidine, angiotensin II, and TGF-β was found to boost their ability to differentiate into cardiomyocytes. Moreover, pre-treating AD-MSCs with IGF-1, VEGF, and bFGF directed their differentiating commitment towards endothelial cells. Bioengineered AD-MSCs that overexpressed the T-Box Transcription Factor 18 (TBX18) gene promoted differentiation into pacemaker cells [33]. In addition to their cardiac differentiation ability, AD-MSCs can activate many paracrine effects which are essential in promoting angiogenesis, recruiting intrinsic cardiac stem cells, reducing fibrosis, and curbing apoptosis [34]. These paracrine effects are mediated by various growth factors, particularly endothelial growth factor (EGF), hepatocyte growth factor (HGF), IGF-1, and microRNAs [2].
Preconditioning AD-MSCs with moderate levels of hypoxia for 24 h enhanced their cardiomyogenesis potential. Priming AD-MSCs with moderate hypoxia can significantly upregulate the levels of cardiomyogenic proteins, GATA-4, and cTnT. The effect of hypoxia in promoting cardiac differentiation in AD-MSCs can be mediated by the high level of HIF-1α, which can induce the transcription of angiogenin, fibroblast growth factor-19 (FGF-19), and macrophage inhibitory factor (MIF). These proteins were identified as inducible factors for increasing the expression of cardiomyogenic markers [35].
The P2Y2 receptor is a uridine triphosphate/adenosine triphosphate (UTP/ATP) G-protein-linked nucleotide receptor connected to phosphoinositide signaling [36]. P2Y2 receptor and UTP ligand have been identified recently as potential regulators of the cardiac regeneration ability of AD-MSCs in rat models of myocardial ischemia. It was reported that UTP treatment in rats with myocardial infarction can result in smaller infarct size, higher capillary density, a better ejection fraction, and less cardiac fibrosis. The angiogenic effect of UTP-treated AD-MSCs may be related to an increased release of pro-angiogenic factors, specifically epiregulin and hyaluronan synthase-1 (Has1) [37]. More recently, neuregulin-1 (NRG1), an endothelial-derived growth factor that acts through erythroblastic leukemia viral oncogene homologue (ErbB) receptor tyrosine kinase, has been demonstrated to enhance the AD-MSCs cardiac repair ability [38,39]. Modulating the Wnt and TGF-β signaling pathways was found to affect the cardiomyogenic differentiation capability of AD-MSCs. Using selective inhibitors for canonical Wnt/β-catenin signaling induced proper cardiomyocyte differentiation while using TGF-β inhibitors promoted cTnT expression in AD-MSCs. Furthermore, inhibiting p38 mitogen-activated protein kinases (p38MAPK) using quercetin can shut down Wnt/β-catenin and non-Smad TGF-β pathways, resulting in enhanced cardiomyocyte differentiation in AD-MSCs [40]. Bone morphogenetic protein 4 (BMP4), which belongs to the TGF-β superfamily, can play an important role in increasing the cardiac differentiation of AD-MSCs [41]. It was demonstrated that combined treatment of AD-MSCs with a low concentration of BMP4 and 5‑azacytidine restored their viability and potentiated their cardiac differentiation capacity compared to 5‑azacytidine treatment alone. That was associated with high protein levels of cTnI, α-sarcomeric actinin (α-SA), Cx43, and sarcoendoplasmic reticulum ATPsase-2A (Serca2α) in the formed cardiomyocyte-like cells. The reported underlying mechanism for these findings involved the activation of the ERK signaling pathway [42]. The enrichment of primitive AD-MSCs with a growth factor cocktail, mainly EGF and PDGF-BB, strengthened their cardiac repair ability. Transplanting these enriched AD-MSCs in Sprague-Dawley (SD) rats with a left anterior descending artery (LAD) model of MI improved left ventricular function parameters and increased the angiogenesis rate at the twelfth-week post-transplantation. Additionally, immunofluorescence revealed that these enriched AD-MSCs expressed high levels of cardiomyogenic proteins, mainly GATA4, β-MHC, and cTnT [43].
ISX1, a member of the 3,5-disubstituted isoxazole family, can activate Nkx2-5. Nkx2-5 is an early marker of the differentiation of AD-MSCs into cardiac myocytes. Transplanting ISX1-treated AD-MSCs in the MI model potentiated the recovery of left ventricular function parameters and initiated neovascularization in the third week following transplantation. The enhanced cardiomyogenesis reported in ISX1-treated AD-MSCs intensified their histone acetyltransferase (HAT) activity, which is crucial to direct their differentiation into cardiac cells [44]. Similarly, pretreatment of AD-MSCs with icariin (ICA) was found to activate the ERK signaling pathway and facilitate their differentiation into cardiomyocytes, as indicated by the upregulation of specific cardiac gene expression, mainly Nkx-2.5, GATA 4, myosin light chain-2 (MLC-2v), α-actinin, and cTnT [45]. The increase in α-actinin level, which is an essential constituent of the cardiomyocyte contractile apparatus, was dependent on the ICA dosage and duration of exposure [45].
Increasing the expression of cardiac-specific miRNAs in AD-MSCs can enhance the chances of getting more cardiomyocytes. For example, it was found that overexpression of miR-1 in AD-MSCs can stimulate Notch signaling and inhibit the expression of hairy and enhancer of split-1 (Hes1) gene, which improves their differentiation into cardiomyocyte-like cells. Co-culturing miR-1 overexpressed AD-MSCs with neonatal mouse cardiomyocytes considerably increased the level of mature cardiomyocyte-specific markers; cTnI and GATA 4 [46].
Despite these positive outcomes, more research should be conducted to determine the best dose, route of administration, and safety of these chemical and genetic manipulations of AD-MSCs before incorporating them into the treatment guidelines for MI. A schematic summary of the cardiac regeneration potential of AD-MSCs can be found in Fig. 2.
Figure 2: The effect of some chemical and genetic modification in enhancing the ability of AD-MSCs differentiation into cardiomyocytes and improving cardiac function. The cardiac regeneration abilities of AD-MSCs can be enhanced by different methods, such as priming these cells with growth factors that are required to direct them toward cardiac regeneration or pre-treating these cells with certain drugs. These engineered AD-MSCs showed greater differentiation tendency to cardiomyocytes and better cardiac repair capabilities. Factors that were found to increase the cardiac repair abilities of AD-MSCs are PDGF-BB, EGF, and NRG1, while factors responsible of improving their differentiation tendency to cardiomyocytes are ISX1, UTP treatment, Quercetin, miRNAs, 5-azacytidine, angiotensin II, TGF-β and hypoxia exposure. These modifications applied to AD-MSCs were effective in improving neoangiogenesis and LVEF and decreasing the infarct size. EGF, Endothelial growth factor; LVEF, Left ventricular ejection fraction; miRNA, micro RNA; MSCs, mesenchymal stem cells; NRG1, Neureugulin-1; PDGF-BB, Platelet-derived growth factor BB; TGF-β, Transforming growth factor beta; UTP, Uridine triphosphate.
Dental pulp-derived mesenchymal stem cells and cardiac regeneration
MSCs have been reported to reside within the perivascular niche of the dental pulp. Two subtypes of dental pulp–derived mesenchymal stem cells (DP-MSCs) have been identified; dental pulp stem cells (DPSCs) and stem cells from human exfoliated deciduous teeth (SHEDs) [47]. DPSCs and SHEDs can renew numerous tissues [48] and restore cardiac function by initiating effective regeneration. Recent research showed enhanced cardiac function in DPSC-treated rat models of MI, due to DPSC’s capability to secrete antiapoptotic and proangiogenic factors. DPSCs also can repair infarcted myocardium, followed by an increase in the number of vessels and a decrease in infarct size [49]. Another study conducted on an I/R mouse model confirmed that DPSCs conditioned medium can ameliorate cardiac damage following I/R injury by reducing the level of inflammatory cytokines and increasing myocardial cell viability [50]. A specific sub-population of DPSCs identified as c-kit+ DPSCs has the potential to produce human cardiovascular progenitor cells [51]. Hypoxia pre-conditioning of DPSCs enhanced their angiogenesis ability by increasing the production of proangiogenic growth factors, including placental growth factor (PGF), VEGF, Ang1, Ang2, PDGFB, and bFGF. The upregulation of these cytokines promoted angiogenesis and improved cardiac function parameters by increasing the survival of DPSCs and inhibiting their apoptosis via the activation of the PI3K-Akt pathway [52]. Moreover, intramyocardial administration of DPSCs in rat models of acute myocardial infarction improved the left ventricular function and increased angiogenesis, thus reducing the infarct size [53]. DPSCs expressed high levels of the following transcription factors; heart and neural crest derivative (HAND2), GATA binding protein 6 (GATA 6) and kinase insert domain receptor (KDR), which are important to promote angiogenesis and potentiate cardiomyogenic differentiation [54,55]. Interestingly, rat DPSCs-MUR1 was found to be ontogenically related to the heart and able to migrate and home within the damaged cardiomyocytes and differentiate into new and functional cardiomyocytes in heart ischemia models. The powerful migration and regeneration abilities of rat DPSCs were correlated with the expression of high levels of chemotactic and paracrine factors, as well as typical markers of cardiac/vascular-like progenitors, mainly stromal cell-derived factor 1 (SDF-1), FGF-2, HGF, Cx43, N-cadherin, c-Kit, endoglin, fetal liver kinase 1 (Flk1), Nkx-2.5, myocyte-specific enhancer factor 2c (Mef2c), GATA4, and adrenergic receptor β2 (ADR-β2) [56]. These promising findings highlighted the cardiac regeneration potential of dental pulp MSCs and their potent abilities to migrate and engraft in the ischemic heart and mitigate the deterioration in heart functions. However, further confirmation using large animal models and clinical trials is needed [56]. The therapeutic role of DPSCs in cardiac disorders have been summarized in Fig. 3.
Figure 3: The perivascular niche of the dental pulp is reported to have the ability to restore cardiac function by secreting anti-apoptotic and proangiogenic factors. Hypoxia pre-conditioning of DPSCs enhances the production of proangiogenic growth factors, including PGF, VEGF, Ang-1, Ang-2, PDGFB, and bFGF. The potent migration and cardiac regeneration abilities of DPSCs were linked with the expression of high levels of chemotactic, paracrine factors and typical markers of cardiac/vascular-like progenitors, mainly SDF-1, HGF, Cx43, N-cadherin, endoglin, Flk1, Nkx-2.5, Mef2c, and ADRB2. ADR-β2, Adrenergic Receptor beta 2; Ang-1, Angiopoietin-1; Ang-2, Angiopoietin-2; bFGF, basic Fibroblast Growth Factor; Cx43, Connexin 43; FGF, Fibroblast Growth Factor; Flk1, fetal liver kinase-1; HGF, Hepatocyte Growth Factor; Mef2c, myocyte-specific enhancer factor-2c; MSCs, mesenchymal stem cells; Nkx-2.5, homeobox protein; PGF, Placental Growth Factor; PDGFB, Platelet Derived Growth Factor B; SDF-1, Stromal cell-derived factor-1; VEGF, Vascular Endothelial Growth Factor.
Umbilical cord-derived mesenchymal stem cells and cardiac regeneration
The umbilical cord is a fetal tissue that originates from the extra-embryonic mesoderm and harbors a niche for MSCs. The umbilical cord is considered a medical waste product, and MSCs can be isolated from it without ethical concerns. UC-MSCs have biological and molecular similarities with BM-MSCs and AD-MSCs, but they tend to be more immune privileged by expressing low levels of MHC-II. In addition, UC-MSCs express low levels of octamer-binding transcription factor 4 (OCT4), NANOG, sex-determining region Y box 2 (SOX2), and Krüppel-like factor 4 (KLF4), and these factors are the main regulators of the transcription process [57]. UC-MSCs were used in animal models of cardiovascular diseases and were effective in amending the worsening of heart function [58]. UC-MSCs, with their strong self-renewal capacity and pluripotency, can be an ideal candidate to replace damaged cardiomyocytes.
UC-MSCs can be functionally modified using chemical compounds, most importantly 5‑azacytidine, hydrogen peroxide (H2O2), and 2′-deoxycytidine (2-DC), which could increase the chances of obtaining cardiac cells from these MSCs. Incubating UC-MSCs with 5‑azacytidine can enhance the ability of UC-MSCs to differentiate into cardiomyocytes, as proved by increased expression of early and mature cardiomyocyte markers, such as Nkx2.5, GATA4, MEF2C, troponin T2 (TNNT2), myosin heavy chain (MYH)-7B, and Cx43 [59]. Although H2O2 can trigger the accumulation of ROS, it has been found that pre-treating UC-MSCs with a low dose of H2O2 can enhance their cardiomyogenesis and increase the expression of cardiomyocyte-related genes, such as GATA4, M1c2a, β-MHC, and cTnI. Further, H2O2 pre-treatment was found to activate the transcription of ion channel-related genes, most importantly the K+ channel and Na+ channel, in UC-MSCs, which potentiate cardiac differentiation potential [60,61]. It was also suggested that adding small molecules, such as 2-DC can stimulate UC-MSCs to differentiate into myocardial cells. Administration of UC-MSCs pre-treated with 2-DC in a rat model of MI was able to improve cardiac systolic and diastolic functions, enhance the ventricular pumping capacity, limit the expansion of cardiac fibrosis, and restore cardiac homeostasis. Cells treated with 2-DC showed gene expression of differentiation and maturity markers of cardiomyocytes including GATA-4, Wnt-2, cTnC, cTnT, MHC, cardiac α-actinin, and Ca+2 and Na+ channels, as well as protein expression of Nkx2.5, GATA-4, Cx43, cardiac α-actinin, and MHC, which confirms cardiomyocyte differentiation [62]. Moreover, co-culturing UC-MSCs with rat cardiomyocytes was found to upregulate the Cx43 gene, Mef2c, cTnT, and myosin heavy chain (MYH)-6. Interestingly, a spontaneous contraction of the cardiomyocytes was observed within a week [63]. Research targeting the cardiac differentiation ability of UC-MSCs is still limited and more studies are needed to optimize the dose, route, and transplanting duration to obtain functional cardiac cells. Despite the difficulties surrounding MSC-based cell therapy, it is important to investigate effective approaches to improve cardiomyogenesis, which can improve the quality of life of patients suffering from heart problems. Although UC-MSCs have enchanted characteristics and exhibit promising regenerative capabilities, their use in large animal studies and clinical studies is still limited. A graphical summary of the cardiomyogenic abilities of UC-MSCs can be found in Fig. 4.
Figure 4: The differentiation potential of umbilical cord mesenchymal stem cells for cardiac regeneration. Incubating UC-MSCs with 5‑ azacytidine increased the expression of cardiac specific markers, such as Nkx2.5, GATA4, Mef2c, TNNT2, (MYH)-7B, Cx43. Pre-treating UC-MSCs with a low dose of H2O2 found to increase the expression of cardiomyocyte-related genes such as GATA4, M1c2a, β-MHC, cTnI and activate the transcription of K+ and Na+ channel-related genes. Combining UC-MSCs with 2-DC was reported to be significantly effective in upregulating the gene expression of cardiac differentiation factors, as well as the maturation markers of cardiomyocytes, including GATA-4, Wnt-2, cTnC, cTnT, MHC, cardiac α-actinin, Ca+2 and Na+ channels, Nkx2.5, Cx43, cardiac α-actinin, and MHC. 2-DC: 2’-deoxycytidine; Cx43: Connexin 43; cTnC: cardiac Troponin C; cTnI: cardiac Troponin I; cTnT: cardiac Troponin; GATA4: GATA binding protein 4; H2O2: hydrogen peroxide; LVEF: Left Ventricular Ejection Fraction; Mef2c: myocyte-specific enhancer factor 2c; MHC: Major Histocompatibility Complex; MSCs: mesenchymal stem cells; MYH7B: myosin heavy chain-7B; Nkx2.5: homeobox protein; TNNT2: Troponin T2.
MSCs are considered a promising cell-based therapy. They can be obtained from multiple sources, such as bone marrow, adipose tissue, umbilical cord, and dental pulps. MSCs are suitable for treating cardiac diseases and restoring the functionality of the damaged heart.
AD-MSCs are a major source of stem cell therapy for cardiac diseases. They are superior to other MSC types in abundance, accessibility, proliferative capacity, anti-inflammatory and anti-fibrotic properties, safety profile, and suitability for allogenic transplantation [64,65]. BM-MSCs exhibit a high proliferation and differentiation tendency, powerful immunomodulatory properties, and migration ability. However, their isolation procedure is invasive, only small tissue volumes can be obtained, and they are not stable in the injured cardiac microenvironment, which hinders their clinical benefits [43]. Despite the encouraging properties of UC-MSCs and DP-MSCs (good immunomodulatory effect and stability in cardiac stress microenvironment, respectively) [66,67], the amount of research on these MSCs is limited compared to BM-MSCs and AD-MSCs. Therefore, the optimal MSC source for the regenerative treatment of cardiac diseases cannot be determined yet.
The regenerative effects of MSCs on the diseased heart are achieved by differentiating into cardiomyocytes or cardiomyocyte-like cells and exerting immunomodulatory effects. Considerable efforts are conducted to investigate the impact of modifying strategies on enhancing the survival of MSCs in the cardiac microenvironment and reprograming them to differentiate into functional cardiac cells. Most of these efforts have yielded promising results in improving heart regeneration and restoring heart function, as demonstrated by increased angiogenesis, improved LVEF, and reduced infarct size. In fact, each type of MSCs has its unique cardiac regenerating capabilities and many cardiovascular diseases are characterized by having complicated and harsh microenvironments. Based on that, it is suggested that combining two types or more of MSCs can generate superior regenerative and repairing outcomes in many cardiac disorders. Future studies should be directed toward investigating the cardiac regenerative abilities of each type of MSCs in large-animal models and in clinical trials to shift these cells from bench to bedside.
Acknowledgement: None.
Funding Statement: No specific funding has been received.
Author Contributions: E.A.E.R Conceptualized the review subtopics. E.A.E.R, Y.A.Z, H.B.I, A.K.S, T.A.A, F.R, M.A, R.R.K, A.Z, and H.M collected the literature used to wrote the review and drafted the manuscript. E.A.E.R, R.R.K and F.A.M revised and formatted the content of the manuscript and verified spelling, punctuation and grammatical errors. All authors have read and approve the final manuscript.
Availability of Data and Materials: Data sharing not applicable to this article as no datasets were generated or analyzed during the current study.
Ethics Approval: Not applicable.
Conflicts of Interest: The authors declare no conflict of interest.
References
1. Zhou LN, Wang JC, Zilundu PLM, Wang YQ, Guo WP, Zhang SX, et al. A comparison of the use of adipose-derived and bone marrow-derived stem cells for peripheral nerve regeneration in vitro and in vivo. Stem Cell Res Ther. 2020;11(1):153. doi:10.1186/s13287-020-01661-3. [Google Scholar] [PubMed] [CrossRef]
2. Li X, Ma T, Sun J, Shen M, Xue X, Chen Y, et al. Harnessing the secretome of adipose-derived stem cells in the treatment of ischemic heart diseases. Stem Cell Res Ther. 2019;10(1):196. doi:10.1186/s13287-019-1289-7. [Google Scholar] [PubMed] [CrossRef]
3. Cook DJ, Webb S, Proudfoot A. Assessment and management of cardiovascular disease in the intensive care unit. Heart. 2022;108(5):397. doi:10.1136/heartjnl-2019-315568. [Google Scholar] [PubMed] [CrossRef]
4. He Y, Han Y, Ye Y. Therapeutic potential of menstrual blood-derived stem cell transplantation for intrauterine adhesions. Front Surg. 2022;9:847213. doi:10.3389/fsurg.2022.847213. [Google Scholar] [PubMed] [CrossRef]
5. Poomani MS, Mariappan I, Perumal R, Regurajan R, Muthan K, Subramanian V. Mesenchymal stem cell (MSCs) therapy for ischemic heart disease: a promising frontier. Glob Heart. 2022;17(1):19. doi:10.5334/gh.1098. [Google Scholar] [PubMed] [CrossRef]
6. Chang D, Fan T, Gao S, Jin Y, Zhang M, Ono M. Application of mesenchymal stem cell sheet to treatment of ischemic heart disease. Stem Cell Res Ther. 2021;12(1):384. doi:10.1186/s13287-021-02451-1. [Google Scholar] [PubMed] [CrossRef]
7. Goto T, Miyagawa S, Tamai K, Matsuura R, Kido T, Kuratani T, et al. High-mobility group box 1 fragment suppresses adverse post-infarction remodeling by recruiting PDGFRα-positive bone marrow cells. PLoS One. 2020;15(4):e0230392. doi:10.1371/journal.pone.0230392. [Google Scholar] [PubMed] [CrossRef]
8. Kahrizi MS, Mousavi E, Khosravi A, Rahnama S, Salehi A, Nasrabadi N, et al. Recent advances in pre-conditioned mesenchymal stem/stromal cell (MSCs) therapy in organ failure; a comprehensive review of preclinical studies. Stem Cell Res Ther. 2023;14(1):155. doi:10.1186/s13287-023-03374-9. [Google Scholar] [PubMed] [CrossRef]
9. Sharaf Eldin HEM, Ibrahim MAA, Mousa AMI, Metwaly HG, Abo-Hassan NFE. Cardiogenic differentiation of murine bone marrow-derived mesenchymal stem cells by 5-azacytidine: a follow-up in vitro study. J Microsc Ultrastruct. 2019;7(4):185–93. doi:10.4103/JMAU.JMAU_17_19. [Google Scholar] [PubMed] [CrossRef]
10. Marino F, Scalise M, Cianflone E, Mancuso T, Aquila I, Agosti V, et al. Role of c-Kit in myocardial regeneration and aging. Front Endocrinol. 2019;10:371. doi:10.3389/fendo.2019.00371. [Google Scholar] [PubMed] [CrossRef]
11. Fathi E, Farahzadi R, Vietor I, Javanmardi S. Cardiac differentiation of bone-marrow-resident c-kit+ stem cells by L-carnitine increases through secretion of VEGF, IL6, IGF-1, and TGF-β as clinical agents in cardiac regeneration. J Biosci. 2020;45(1):92. doi:10.1007/s12038-020-00063-0. [Google Scholar] [CrossRef]
12. Haneef K, Ali A, Khan I, Naeem N, Jamall S, Salim A. Role of interleukin-7 in fusion of rat bone marrow mesenchymal stem cells with cardiomyocytes in vitro and improvement of cardiac function in vivo. Cardiovasc Ther. 2018;36(6):e12479. doi:10.1111/1755-5922.12479. [Google Scholar] [PubMed] [CrossRef]
13. Lin M, Liu X, Zheng H, Huang X, Wu Y, Huang A, et al. IGF-1 enhances BMSC viability, migration, and anti-apoptosis in myocardial infarction via secreted frizzled-related protein 2 pathway. Stem Cell Res Ther. 2020;11(1):22. doi:10.1186/s13287-019-1544-y. [Google Scholar] [PubMed] [CrossRef]
14. Gu J, Li H, Wei W, Sun XL, Li B, Chen Y, et al. Bone marrow mesenchymal stem cell transplantation alleviates radiation-induced myocardial fibrosis through inhibition of the TGF-β1/Smad2/3 signaling pathway in rabbit model. Regen Ther. 2023;24:1–10. doi:10.1016/j.reth.2023.04.003. [Google Scholar] [PubMed] [CrossRef]
15. Lv Y, Liu B, Liu Y, Wang HY, Wang HP. TGF-β1 combined with Sal-B promotes cardiomyocyte differentiation of rat mesenchymal stem cells. Exp Ther Med. 2018;15(6):5359–64. doi:10.3892/etm.2018.6105. [Google Scholar] [PubMed] [CrossRef]
16. Raman N, Imran SAM, Ahmad Amin Noordin KB, Wan Kamarul Zaman WS, Nordin F. Mechanotransduction of mesenchymal stem cells (MSCs) during cardiomyocytes differentiation. Heliyon. 2022;8(11):e11624. doi:10.1016/j.heliyon.2022.e11624. [Google Scholar] [PubMed] [CrossRef]
17. Chen X, Zhang Y, Wang W, Liu Z, Meng J, Han Z. Mesenchymal stem cells modified with heme oxygenase-1 have enhanced paracrine function and attenuate lipopolysaccharide-induced inflammatory and oxidative damage in pulmonary microvascular endothelial cells. Cell Physiol Biochem. 2018;49(1):101–22. doi:10.1159/000492847. [Google Scholar] [PubMed] [CrossRef]
18. Cho J, Rameshwar P, Sadoshima J. Distinct roles of glycogen synthase kinase (GSK)-3α and GSK-3β in mediating cardiomyocyte differentiation in murine bone marrow-derived mesenchymal stem cells*. J Biol Chem. 2009;284:36647–58. doi:10.1074/jbc.M109.019109. [Google Scholar] [PubMed] [CrossRef]
19. Cho J, Zhai P, Maejima Y, Sadoshima J. Myocardial injection with GSK-3β-overexpressing bone marrow-derived mesenchymal stem cells attenuates cardiac dysfunction after myocardial infarction. Circ Res. 2011;108(4):478–89. doi:10.1161/CIRCRESAHA.110.229658. [Google Scholar] [PubMed] [CrossRef]
20. Shen H, Cui G, Li Y, Ye W, Sun Y, Zhang Z, et al. Follistatin-like 1 protects mesenchymal stem cells from hypoxic damage and enhances their therapeutic efficacy in a mouse myocardial infarction model. Stem Cell Res Ther. 2019;10(1):689. doi:10.1186/s13287-018-1111-y. [Google Scholar] [PubMed] [CrossRef]
21. Zhang F, Wang C, Lin J, Wang X. Oxidized low-density lipoprotein (ox-LDL) promotes cardiac differentiation of bone marrow mesenchymal stem cells via activating ERK1/2 signaling. Cardiovasc Ther. 2017;35(6):e12305. doi:10.1111/1755-5922.12305. [Google Scholar] [PubMed] [CrossRef]
22. Xie DM, Li Y, Li J, Li Q, Lu G, Zhai YS, et al. CD51 distinguishes a subpopulation of bone marrow mesenchymal stem cells with distinct migratory potential: a novel cell-based strategy to treat acute myocardial infarction in mice. Stem Cell Res Ther. 2019;10:331. doi:10.1186/s13287-019-1439-y. [Google Scholar] [PubMed] [CrossRef]
23. Li SH, Sun L, Yang L, Li J, Shao Z, Du GQ, et al. Young bone-marrow sca-1+ stem cells rejuvenate the aged heart and improve function after injury through PDGFRβ-Akt pathway. Sci Rep. 2017;7:41756. doi:10.1038/srep41756. [Google Scholar] [PubMed] [CrossRef]
24. Li TV, Gu J, Yang O, Wang J, Wang Y, Kong J. Bone marrow mesenchymal stem cell-derived exosomal miRNA-29c decreases cardiac ischemia/reperfusion injury through inhibition of excessive autophagy via the PTEN/Akt/mTOR signaling pathway. Circ J. 2020;84(8):1304–11. doi:10.1253/circj.CJ-19-1060. [Google Scholar] [PubMed] [CrossRef]
25. Lu M, Xu Y, Wang M, Guo T, Luo F, Su N, et al. MicroRNA-23 inhibition protects the ischemia/reperfusion injury via inducing the differentiation of bone marrow mesenchymal stem cells into cardiomyocytes. Int J Clin Exp. 2019;12(3):1060–9. [Google Scholar]
26. Song YQ, Lim JY, Lim T, Im KI, Kim N, Nam YS, et al. Human mesenchymal stem cells derived from umbilical cord and bone marrow exert immunomodulatory effects in different mechanisms. World J Stem Cells. 2020;12:1032–49. doi:10.4252/wjsc.v12.i9.1032. [Google Scholar] [PubMed] [CrossRef]
27. Wu M, Zhang R, Zou Q, Chen Y, Zhou M, Li X, et al. Comparison of the biological characteristics of mesenchymal stem cells derived from the human placenta and umbilical cord. Sci Rep. 2018;8(1):5014. doi:10.1038/s41598-018-23396-1. [Google Scholar] [PubMed] [CrossRef]
28. Zhao L, Johnson TK, Liu D. Therapeutic angiogenesis of adipose-derived stem cells for ischemic diseases. Stem Cell Res Ther. 2017;8:125. doi:10.1186/s13287-017-0578-2. [Google Scholar] [PubMed] [CrossRef]
29. Mathur N, Severinsen MCK, Jensen ME, Naver L, Schrölkamp M, Laye MJ, et al. Human visceral and subcutaneous adipose stem and progenitor cells retain depot-specific adipogenic properties during obesity. Front Cell Dev Biol. 2022;10:983899. doi:10.3389/fcell.2022.983899. [Google Scholar] [PubMed] [CrossRef]
30. Mueller P, Wolfien M, Ekat K, Lang CI, Koczan D, Wolkenhauer O, et al. RNA-based strategies for cardiac reprogramming of human mesenchymal stromal cells. Cells. 2020;9(2):504. doi:10.3390/cells9020504. [Google Scholar] [PubMed] [CrossRef]
31. Bruun K, Schermer E, Sivendra A, Valaik E, Wise RB, Said R, et al. Therapeutic applications of adipose-derived stem cells in cardiovascular disease. Am J Stem Cells. 2018;7(4):94–103. [Google Scholar] [PubMed]
32. Al-Ghadban S, Artiles M, Bunnell BA. Adipose stem cells in regenerative medicine: looking forward. Front Bioeng Biotechnol. 2022;9:837464. doi:10.3389/fbioe.2021.837464. [Google Scholar] [PubMed] [CrossRef]
33. Gorabi AM, Hajighasemi S, Tafti HA, Atashi A, Soleimani M, Aghdami N, et al. TBX18 transcription factor overexpression in human-induced pluripotent stem cells increases their differentiation into pacemaker-like cells. J Cell Physiol. 2018;234:1534–46. doi:10.1002/jcp.27018. [Google Scholar] [PubMed] [CrossRef]
34. Rautiainen S, Laaksonen T, Koivuniemi R. Angiogenic effects and crosstalk of adipose-derived mesenchymal stem/stromal cells and their extracellular vesicles with endothelial cells. Int J Mol Sci. 2021;22(19):10890. doi:10.3390/ijms221910890. [Google Scholar] [PubMed] [CrossRef]
35. Choi JW, Moon H, Jung SE, Lim S, Lee S, Kim IK, et al. Hypoxia rapidly induces the expression of cardiomyogenic factors in human adipose-derived adherent stromal cells. J Clin Med. 2019;8(8):1231. doi:10.3390/jcm8081231. [Google Scholar] [PubMed] [CrossRef]
36. Li W, Wei S, Liu C, Song MY, Wu H, Yang Y. Regulation of the osteogenic and adipogenic differentiation of bone marrow-derived stromal cells by extracellular uridine triphosphate: the role of P2Y2 receptor and ERK1/2 signaling. Int J Mol Med. 2015;37:63–73. doi:10.3892/ijmm.2015.2400. [Google Scholar] [PubMed] [CrossRef]
37. Vanorlé M, Lemaire A, di Pietrantonio L, Horckmans M, Communi D. UTP is a regulator of in vitro and in vivo angiogenic properties of cardiac adipose-derived stem cells. Purinergic Signal. 2021;17:681–91. doi:10.1007/s11302-021-09812-8. [Google Scholar] [PubMed] [CrossRef]
38. Wang Y, Wei J, Zhang P, Zhang X, Wang Y, Chen W, et al. Neuregulin-1, a potential therapeutic target for cardiac repair. Front Pharmacol. 2022;13:945206. doi:10.3389/fphar.2022.945206. [Google Scholar] [PubMed] [CrossRef]
39. Wadugu BA, Kühn B. The role of neuregulin/ErbB2/ErbB4 signaling in the heart with special focus on effects on cardiomyocyte proliferation. Am J Physiol Heart Circ Physiol. 2012;302(11):H2139–47. doi:10.1152/ajpheart.00063.2012. [Google Scholar] [PubMed] [CrossRef]
40. Azadian Z, Hosseini S, Dizjikan ZP, Kazemi J, Marzouni ET, Wang P, et al. Computational and in vitro validation of cardiogenic induction of quercetin on adipose-derived mesenchymal stromal cells through the inhibition of Wnt and non-Smad-dependent TGF-β pathways. J Cell Biochem. 2021;123:450–68. doi:10.1002/jcb.30189. [Google Scholar] [PubMed] [CrossRef]
41. Setiawan AM, Kamarudin TA, Ghafar NA. The role of BMP4 in adipose-derived stem cell differentiation: a minireview. Front Cell Dev Biol. 2022;10:1045103. doi:10.3389/fcell.2022.1045103. [Google Scholar] [PubMed] [CrossRef]
42. Jiang A, Chen YW, Shi L, Li F. Differentiation of brown adipose-derived stem cells into cardiomyocyte-like cells is regulated by a combination of low 5-azacytidine concentration and bone morphogenetic protein 4. Int J Clin Exp Pathol. 2018;11(11):5514–24, PMCID: PMC6963047. [Google Scholar] [PubMed]
43. Guo X, Bai Y, Zhang L, Zhang B, Zagidullin NS, Carvalho KAT, et al. Cardiomyocyte differentiation of mesenchymal stem cells from bone marrow: new regulators and its implications. Stem Cell Res Ther. 2018;9:44. doi:10.1186/s13287-018-0773-9. [Google Scholar] [PubMed] [CrossRef]
44. Burchfield JS, Paul AL, Lanka V, Tan W, Kong Y, McCallister C, et al. Pharmacological priming of adipose-derived stem cells promotes myocardial repair. J Investig Med. 2016;64:50–62. doi:10.1136/jim-2015-000018. [Google Scholar] [PubMed] [CrossRef]
45. Jin M, Shi S, Zhang Y, Yan Y, Sun XD, Liu W, et al. Icariin-mediated differentiation of mouse adipose-derived stem cells into cardiomyocytes. Mol Cell Biochem. 2010;344:1–9. doi:10.1007/s11010-010-0523-5. [Google Scholar] [PubMed] [CrossRef]
46. Chen C, Yan Q, Yan Y, Ma M, He Y, Shui X, et al. MicroRNA-1 regulates the differentiation of adipose-derived stem cells into cardiomyocyte-like cells. Stem Cells Int. 2018;2018:7494530. doi:10.1155/2018/7494530. [Google Scholar] [PubMed] [CrossRef]
47. Zhu SY, Yuan C, Lin YF, Liu H, Yang YQ, Wong HM, et al. Stem cells from human exfoliated deciduous teeth (SHEDs) and dental pulp stem cells (DPSCs) display a similar profile with pericytes. Stem Cells Int. 2021;2021:8859902. doi:10.1155/2021/8859902. [Google Scholar] [PubMed] [CrossRef]
48. Mattei V, Monache SD. Dental pulp stem cells (DPSCs) and tissue regeneration: mmechanisms mediated by direct, paracrine, or autocrine effects. Biomedicines. 2023;11(2):386. doi:10.3390/biomedicines11020386. [Google Scholar] [PubMed] [CrossRef]
49. Mattei V, Martellucci S, Pulcini F, Santilli F, Sorice M, Monache SD. Regenerative potential of DPSCs and revascularization: ddirect, paracrine or autocrine effect? Stem Cell Rev Rep. 2021;17:1635–46. doi:10.1007/s12015-021-10162-6. [Google Scholar] [PubMed] [CrossRef]
50. Yamaguchi S, Shibata R, Yamamoto N, Nishikawa M, Hibi H, Tanigawa T, et al. Dental pulp-derived stem cell conditioned medium reduces cardiac injury following ischemia-reperfusion. Sci Rep. 2015;5:16295. doi:10.1038/srep16295. [Google Scholar] [PubMed] [CrossRef]
51. Enkhmandakh B, Bayarsaihan D. Single-cell transcriptome profiling reveals distinct expression patterns among genes in the mouse incisor dental pulp. Int J Dev Biol. 2023;67:19–25. doi:10.1387/ijdb.220173db. [Google Scholar] [PubMed] [CrossRef]
52. Pankajakshan D, Agrawal DK. Mesenchymal stem cell paracrine factors in vascular repair and regeneration. J Biomed Technol Res. 2014;1(1):10. doi:10.19104/jbtr.2014.107. [Google Scholar] [PubMed] [CrossRef]
53. Hajishoreh NK, Baheiraei N, Naderi N, Salehnia M, Razavi M. Left ventricular geometry and angiogenesis improvement in rat chronic ischemic cardiomyopathy following injection of encapsulated mesenchymal stem cells. Cell J (Yakhteh). 2022;24:741–7. doi:10.22074/CELLJ.2022.557257.1040. [Google Scholar] [PubMed] [CrossRef]
54. Peng T, Deng X, Tian F, Li Z, Jiang P, Zhao X, et al. The interaction of LOXL2 with GATA6 induces VEGFA expression and angiogenesis in cholangiocarcinoma. Int J Oncol. 2019;55:657–70. doi:10.3892/ijo.2019.4837. [Google Scholar] [PubMed] [CrossRef]
55. Masuda S, Matsuura K, Shimizu T. GATA6 regulates anti-angiogenic properties in human cardiac fibroblasts via modulating LYPD1 expression. Regen Ther. 2023;23:8–16. doi:10.1016/j.reth.2023.02.005. [Google Scholar] [PubMed] [CrossRef]
56. Scipio FD, Sprio AE, Folino A, Carere ME, Salamone P, Yang Z, et al. Injured cardiomyocytes promote dental pulp mesenchymal stem cell homing. Biochim Biophys Acta. 2014;1840(7):2152–61. doi:10.1016/j.bbagen.2014.03.005. [Google Scholar] [PubMed] [CrossRef]
57. Lu Y, Qu H, Qi D, Xu W, Liu S, Jin X, et al. OCT4 maintains self-renewal and reverses senescence in human hair follicle mesenchymal stem cells through the downregulation of p21 by DNA methyltransferases. Stem Cell Res Ther. 2019;10(1):28. doi:10.1186/s13287-018-1120-x. [Google Scholar] [PubMed] [CrossRef]
58. Colicchia M, Jones DA, Beirne AM, Hussain M, Weeraman D, Rathod K, et al. Umbilical cord-derived mesenchymal stromal cells in cardiovascular disease: review of preclinical and clinical data. Cytother. 2019;21(10):1007–18. doi:10.1016/j.jcyt.2019.04.056. [Google Scholar] [PubMed] [CrossRef]
59. Bartolucci J, Verdugo FJ, González PL, Larrea RE, Abarzua E, Goset C, et al. Safety and efficacy of the intravenous infusion of umbilical cord mesenchymal stem cells in patients with heart failure. Circ Res. 2017;121(10):1192–204. doi:10.1161/CIRCRESAHA.117.310712. [Google Scholar] [PubMed] [CrossRef]
60. Subramani B, Subbannagounder S, Ramanathanpullai C, Palanivel S, Ramasamy R. Impaired redox environment modulates cardiogenic and ion-channel gene expression in cardiac-resident and non-resident mesenchymal stem cells. Exp Biol Med. 2017;242:645–56. doi:10.1177/1535370216688568. [Google Scholar] [PubMed] [CrossRef]
61. Subramani B, Subbannagounder S, Palanivel S, Ramanathanpullai C, Sivalingam S, Yakub A, et al. Generation and characterization of human cardiac resident and non-resident mesenchymal stem cell. Cytotechnol. 2016;68:2061–73. doi:10.1007/s10616-016-9946-5. [Google Scholar] [PubMed] [CrossRef]
62. Ali SR, Ahmad W, Naeem N, Salim A, Khan I. Small molecule 2′-deoxycytidine differentiates human umbilical cord-derived MSCs into cardiac progenitors in vitro and their in vivo xeno-transplantation improves cardiac function. Mol Cell Biochem. 2020;470:99–113. doi:10.1007/s11010-020-03750-6. [Google Scholar] [PubMed] [CrossRef]
63. Szaraz P, Gratch YS, Iqbal F, Librach CL. In vitro differentiation of human mesenchymal stem cells into functional cardiomyocyte-like cells. J Vis Exp. 2017;126:e55757. doi:10.3791/55757. [Google Scholar] [PubMed] [CrossRef]
64. Sheykhhasan M, Wong JKL, Seifalian AM. Human adipose-derived stem cells with great therapeutic potential. Curr Stem Cell Res Ther. 2019;14(7):532–48. doi:10.2174/1574888X14666190411121528. [Google Scholar] [PubMed] [CrossRef]
65. West CC, Hardy WR, Murray IR, James AW, Corselli M, Pang S, et al. Prospective purification of perivascular presumptive mesenchymal stem cells from human adipose tissue: process optimization and cell population metrics across a large cohort of diverse demographics. Stem Cell Res Ther. 2016;7:47. doi:10.1186/s13287-016-0302-7. [Google Scholar] [PubMed] [CrossRef]
66. Braid LR, Wood CA, Ford BN. Human umbilical cord perivascular cells: a novel source of the organophosphate antidote butyrylcholinesterase. Chem Biol Interact. 2019;305:66–78. doi:10.1016/j.cbi.2019.03.022. [Google Scholar] [PubMed] [CrossRef]
67. Yoshida S, Tomokiyo A, Hasegawa D, Hamano S, Sugii H, Maeda H. Insight into the role of dental pulp stem cells in regenerative therapy. Biology. 2020;9(7):160. doi:10.3390/biology9070160. [Google Scholar] [PubMed] [CrossRef]
Cite This Article
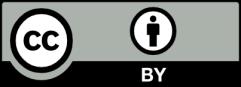
This work is licensed under a Creative Commons Attribution 4.0 International License , which permits unrestricted use, distribution, and reproduction in any medium, provided the original work is properly cited.