Open Access
REVIEW
How aging affects bone health via the intestinal micro-environment
1 School of Stomatology, Zunyi Medical University, Zunyi, China
2 Microbial Resources and Drug Development Key Laboratory of Guizhou Tertiary Institution, Zunyi Medical University, Zunyi, China
3 Department of Stomatology, Luoyang Maternal and Child Health Hospital, Luoyang, China
* Corresponding Authors: HUAN HU. Email: ; YANZI YAO. Email:
(This article belongs to the Special Issue: Gut Microbiota in Human Health: Exploring the Complex Interplay)
BIOCELL 2024, 48(3), 353-362. https://doi.org/10.32604/biocell.2024.048311
Received 04 December 2023; Accepted 11 January 2024; Issue published 15 March 2024
Abstract
Increasing life expectancy and an aging population lead to age-related bone diseases like osteoporosis and low bone mass more prevalent. These conditions represent a common, costly and chronic burden, not only for elderly but also to society at large. Consequently, elucidating the pathophysiology and developing effective therapies for these diseases is of paramount importance. Recent advances in research have identified the gut as a novel and promising target for addressing bone disorders, giving rise to the concept of the “gut-bone axis”. An in-depth review of the latest insights into the effects of age-related physiological changes in the gastrointestinal tract on bone health is presented in this article. It examines how the “gut-bone” axis interacts with bone aging across various domains, including metabolism, nutrition, intestinal permeability, immunity, and oxidative stress.Keywords
The human intestinal tract harbors trillions of microorganisms that constitute a selective permeability barrier, essential for safeguarding against the ingress of deleterious entities such as exogenous antigens, pathogens, and toxins. Simultaneously, this barrier facilitates the absorption of vital nutrients, electrolytes, and immune sensing. The maintenance of intestinal barrier integrity is vital for overall health, with its compromise leading to the opportunistic penetration of pathogenic bacteria and their metabolites, potentially inflicting damage on distant organs and overall well-being. The intestinal microenvironment is linked to a variety of diseases and is shaped by an array of factors, including age, genetics, environmental exposure, lifestyle, stress, and diet [1].
Aging is a complex process characterized by the progressive decline of physiological systems and the deterioration of organ structures and functions. It is often accompanied by disruptions to the integrity of the intestinal barrier, changes in the composition of gut microbiota (GM), and an increased susceptibility to various aging-related diseases, including neurodegenerative disorders, cardiovascular conditions, metabolic disorders, musculoskeletal ailments, immune system dysregulation, and cancers [2]. Among these diseases, there have been reports highlighting skeletal issues associated with aging, such as bone loss, degenerating articular cartilage, and narrowing of intervertebral discs, which are precursors to conditions like osteoporosis, arthritis, and fractures [3,4]. Bone health is intricately regulated by the dynamic balance between bone formation and resorption, which is mediated by osteoblasts and osteoclasts, respectively. However, during aging, the cell lineages of skeletal system underwent rigorous changes, senescence accumulation in osteoprogenitors like bone marrow mesenchymal stem cells (BMSCs) lead to impaired osteogenesis and cause imbalance [5]. Additionally, in senescence-related cancer, BMSCs also contribute to the formation of a cancer-promoting microenvironment [6,7].
However, the precise causal relationships or correlations between these phenomena and age-related changes in the intestinal microenvironment remain poorly understood. This knowledge gap represents a great challenge, particularly in terms of understanding the implications for bone health and the potential underlying regulatory mechanisms.
Accordingly, this review endeavors to enhance the current comprehension of the interplay between the age-related intestinal microenvironment and bone health. It also aims to pinpoint the research lacunae that warrant further investigation.
Aging and Bone Health: The Role of the Intestinal Barrier
Regulation of skeletal system is influenced by a myriad of signals, including inflammatory factors and endocrine hormones. Notably, the intestinal barrier is integral to this regulatory network. These interactions are critical for managing systemic chronic low-grade inflammation and metabolism, which have significant implications for bone health.
The endocrine hormones that regulate bone health in elderly individuals primarily include estrogen, parathyroid hormone, insulin-like grown factor-1, and serotonin (5-HT). The gastrointestinal tract, as the largest endocrine organ, secretes hormones such as glucose-dependent insulinotropic peptide (GIP) and glucagon-like peptide-1 and 2 (GLP-1, GLP-2), which have profound effects on bone metabolism and are considered essential components of the gut-bone axis [8,9]. For instance, GIP has been found to reduce the levels of biochemical marker carboxy-terminal collagen crosslinks (CTX), which is indicative of bone absorption. Supplementing with exogenous GIP has been shown to effectively mitigate bone absorption in postmenopausal women [10,11]. Conversely, GLP-1 receptor agonists promote osteoblastogenesis and suppress bone resorption through the advanced glycation endproducts (AGEs)-receptor of AGE (RAGE)-reactive oxygen species (ROS, AGEs-RAGE-ROS) pathway and GLP-1 receptor interaction [12]. Maintaining high GIP levels, particularly in aging individuals, especially pre-/post-menopausal women, can thus play a protective role against bone strength deterioration.
Approximately 95% of 5-HT is produced in the intestines, where it inhibits osteoblast proliferation and bone growth by binding to receptors on pre-osteoblasts. Targeted knockout of tryptophan hydroxylase 1 gene (Tph1), the rate-limiting enzyme for intestinal 5-HT synthesis, in enterocytes has been found to increase osteoblast numbers and bone formation, resulting in high bone mass [13]. Conversely, brain-derived 5-HT has been shown to promote bone formation by inhibiting peripheral autonomic nerves [14]. Thus, intestinal-derived 5-HT may hold promise as a potential therapeutic approach for increasing bone mass.
Depression in the elderly is associated with osteoporosis [15]. Although selective serotonin reuptake inhibitors (SSRIs) are effective as antidepressants, they have been implicated in reduced bone mineral density (BMD) and increased fracture risk. This possibly due to desensitization of the 5-HT receptor 2C (HTR2C), which mediates brain-derived 5-HT’s effects on bone [16]. A novel drug, (R)-ketamine, has shown efficacy in effectively improving BMD in ovariectomized mice with depression. This improvement is attributed to its anti-inflammatory actions, which involve the regulation of dysregulated intestinal microbiota and its metabolites. Specifically, at the phylum level of intestinal flora, there was a decrease in the abundance of Tenericutes and an increase in Kiloniella. Additionally, there were decreased levels of metabolites such as succinic acid and dihydrouracil [17].
Intestinal barrier homeostasis
The maintenance of intestinal barrier homeostasis is crucial for bone health. Disruptions to the gut flora, such as those induced by prednisolone, can lead to intestinal barrier dysfunction, increased serum endotoxin levels, inhibition of Wnt10b signaling, and apoptosis of osteoblasts and osteocytes, culminating in glucocorticoid-induced osteoporosis (GIO). Conversely, mucus supplements can bolster barrier function and counteract trabecular bone loss caused by glucocorticoids [18].
Although direct evidence is scarce, age-related intestinal changes are strongly correlated with bone loss. The intestinal barrier, composed of the mucus layer, epithelial cell layer, and lamina propria, is critical for preventing the entry of harmful substances and maintaining homeostasis. However, its integrity is compromised by aging [19] (Fig. 1).
Figure 1: The impact of aging on intestinal barrier regulation and its negative effect on bone health. (1) the maladaptive remodeling of the gut microbiota (GM), characterized by declined diversity and stability, decreased beneficial microorganisms, and increased facultative anaerobic and pathogenic bacteria. Additionally, aging leads to a reduction in mucus thickness, dysregulation of antimicrobial peptide (AMP) expression, and bacteria translocation; (2) the downregulation of tight junction proteins, resulting in compromised intercellular interaction and barrier integrity; (3) the activation of intestinal immune cells due to aging-related altered microbiota and epithelial barrier dysfunction, coupled with a decline in gut mucosal immune system, resulting in local and systemic inflammation. These aging-associated changes in gut microecology, including remodeled gut flora, increased gut permeability, and imbalanced gut immune homeostasis, contribute to the development of age-related bone diseases. Abbreviation list: GALT: gut-associated lymphoid tissues, GI: gastrointestinal, M cell: microfold cell, DC: dendritic cell.
The integrity of the intestinal epithelial cell (IEC) layer relies heavily on the health of tight junction proteins and intestinal epithelial stem cells (IESCs). In the process of aging, there is a decrease in the expression of tight junction proteins such as zonula occludens (ZOs), occludins, claudins, and junctional adhesion molecules (JAMs, Fig. 1), resulting in increased colon permeability [19]. Furthermore, aging disrupts the balance between JUN kinase/protein phosphatase I and results in increased intestine-specific actin (ACT-5) phosphorylation, compromising intestinal intercellular interaction and barrier integrity. The number and proliferative capacity of IESCs also decline with age, impeding the timely self-renewal and repair of the intestinal mucosa [20]. This decline may be linked to the downregulation of Wnt and bone morphogenetic protein (BMP) signaling pathways [21,22]. Clinical and experimental evidence suggests that age-related disruption of the intestinal epithelial barrier is linked to immune activation, elevated inflammation, and reduced bone density [23].
The disruption of the intestinal mucus layer is characterized by a decrease in mucus secretion and thickness, as well as increased degradation and permeability. Such changes expose the IECs to bacteria, precipitating infections and inflammatory conditions like ulcerative colitis (UC) and Crohn’s disease (CD) [24,25] (Fig. 1). Mccin2 (MUC2), the major mucus component, imparts gel-like properties through its abundant and variable O-linked oligosaccharides (O-glycans) [26]. In elderly hosts, upregulation of miR-124-3p correlates with diminished mucus thickness and frequent bacterial translocation, attributable to suppressed O-glycan expression. Moreover, the age-related thinning of the mucus layer may stem from downregulated mucus biosynthesis genes, a proliferation of pathogenic microbes, and a reduction in beneficial bacterial populations [27].
The host and intestinal resident bacteria form a complex, symbiotic micro-ecosystem that acts as a biological defense against external pathogens. Aging, however, can disrupt this delicate equilibrium, leading to a decrease in beneficial gut bacteria and a proliferation of pathogenic strains [28]. The gut microbiome undergoes maladaptive changes with aging (Fig. 1), including a reduction in bacteria that produce short-chain fatty acids (SCFAs) and an elevated Firmicutes/Bacteroidetes (F/B) ratio [29,30]. In peri-/post-menopausal women with low BMD, there is often a higher abundance of Bacteroides vulgatus and a lower level of serum valeric acid [31]. Interestingly, studies have shown that colonizing mice with the GM of healthy children can reverse the reduction of Akkermansia muciniphila caused by ovariectomy and prevent estrogen deficiency-induced osteoporosis [32]. Similarly, transplantation of the GM from wild-type mouse or A. muciniphila into prematurely aging mice has been found to restore secondary bile acid metabolism, normalize age-accelerated gut dysbiosis, and improve overall health and longevity [33]. This reshaping of the GM with age heightens the risk of systemic inflammation and age-related bone diseases [34–36].
The gut mucosal immune system is primarily comprised of gut-associated lymphoid tissue (GALT), which includes a variety of lymphoid tissues like Peyer’s patches (PPs) in the small intestine, cecal patches and appendix, as well as isolated lymphoid follicles (ILFs). As individuals age, there is a notable decline in the functional maturation of Microfold (M) cells within the follicle-associated epithelia that overlay PPs, which are specialized for transepithelial transport. This decline hampers antigen presentation capabilities [37]. Concurrently, plasmacytoid dendritic cells (pDCs), pivotal in detecting pathogens or infection signals, show age-related impairments in migration, a reduction in both absolute numbers and proportions, culminating a marked decrease in mucosal immune efficiency [38]. In the mucosal tissue of aged host, isolated DCs display a compromised immune priming function, diminishing their capacity to initiate antigen-specific T-cell responses [39]. Furthermore, aging is associated with a significant downregulation of genes involved in innate and adaptive immunity, including a decreased expression of T cell-specific transcripts and alterations in T cell signaling pathways [40].
CD4 T cells, which represent a majority of T cells in the intestinal lamina propria, demonstrate a diminished expression of inhibitory receptors, increased rated of spontaneous apoptosis, decreased frequencies of specific Th cell subsets, and altered functional responses due to aging [41]. These changes hinder the T cell responses to the GM, leading to both local and systemic inflammation in the elderly. Moreover, heightened inflammation within the bone marrow microenvironment is implicated in bone loss [42,43]. Conversely, studies have shown that dietary intervention with prebiotics in accelerated aging (SAMP6) mice can enhance the GM, mitigate systemic inflammation, and reduce bone resorption, underscoring the importance of the gut microenvironment in the aging process and its influence on bone health [44].
Regulatory Pathways of the Gut-Bone Axis on Age-Related Bone Diseases
The age-related changes in intestinal barrier function are believed to negatively impact bone health in older individuals. It is therefore reasonable to speculate that enhancing intestinal barrier function could improve bone health in the elderly. Various strategies, such as dietary and lifestyle modifications, pharmacological interventions, probiotic supplementation, exercise, and modulation of the intestinal microenvironment have been identified as potential approaches to regulate bone health. These strategies act through pathways involving immune regulation, nutrient absorption, neuronal signals, hormonal pathways, metabolism, microRNA, intestinal barrier function, and oxidative stress (Fig. 2).
Figure 2: Regulatory pathways of the gut-bone axis on bone diseases. The shifts in diet, lifestyle, intakes of drugs and probiotics, exercise, or other interventions can pose various effects on the intestinal micro-environments, and regulate age-related bone health via metabolism, nutrition, immunity, and oxidative stress. Abbreviation list: SCFAs: short-chain fatty acids, H2S: hydrogen sulfide, BAs: bile acids, TGR5: G-protein-coupled bile acid receptor, GLP-1: glucagon-like peptide-1, Treg: upregulating regulatory T cells, sIgA: secretory immunoglobulin A, FMO3: flavin containing dimethylaniline monoxygenase 3, TMAO: trimethylamine-N-oxide, ATCC334: Lactobacillus acidophilus stain.
The GM can produce a diverse array of bioactive compounds in response to dietary nutrients. These metabolites act as signaling molecules, facilitating communication with the endocrine system, immune system, and host metabolism. This network, known as host-microbe metabolic axis, involves various microbial species and host cell pathways and is crucial in regulating metabolic homeostasis.
SCFAs, such as formic acid, acetic acid, propionic acid, and butyric acid, are produced by microbial fermentation of indigestible carbohydrates in the gut [45]. They play a vital role in maintaining intestinal barrier integrity by regulating gut pH, stimulating mucus production, providing fuel for IECs, and modulating mucosal immune response [46,47]. SCFAs also act as critical regulators and mediators of gut-bone homeostasis. Studies have shown that direct supplementation of SCFAs or a high-fiber diet can inhibit osteoclast differentiation by directly suppressing the expression of genes involves in osteoclastogenesis, such as TRAF6 (tumor necrosis factor (TNF) receptor associated factor 6) and NFATc1 (nuclear factor-activated T cell 1), while upregulating regulatory T cells (Tregs) populations, thereby improving bone mass and reducing postmenopausal bone loss [48]. Similarly, oral administration of supplements such as fructooligosaccharides and inulin can inhibit osteoclastogenesis and bone resorption by increasing SCFA levels and maintaining GM homeostasis, intestinal permeability, and intestinal immune function, thereby preventing bone loss induced by estrogen deficiency [49]. Additionally, the probiotic strain Lactobacillus plantarum TWK10 has also been found to mitigate age-related bone loss by modulating gut dysbiosis and increasing total SCFA levels [50].
Polyamines are fatty amines that act as physiological regulators of intestinal development and barrier integrity [51]. However, with age, both the levels of polyamines and their biosynthetic capacity decline [52]. Adequate supplementation of polyamines has been found effective in treating cardiovascular diseases, metabolic bone diseases, and in delaying cellular aging [53,54]. Heat exposure can increase the abundance of polyamine-producing bacteria and enhance bacterial polyamine synthesis capacity, thus alleviating the age-related decline in total polyamine levels and improving bone loss caused by ovariectomy [55]. Direct supplementation of natural polyamine agents can also prevent bone loss by interfering with osteoclast differentiation and maturation [56]. Enhancing polyamine biosynthesis in gut bacteria may inhibit abnormal osteoclast activation and holds promise as a preventive and therapeutic approach for age-related metabolic bone diseases.
Hydrogen sulfide (H2S) is a signaling molecule in the form of gas that is generated by cysteine in the intestine, produced by epithelial cells and GM. It serves as an energy source for gastrointestinal epithelial cells and is crucial in maintaining mucosal integrity. It has been discovered that H2S has the ability to inhibit lymphocyte infiltration and suppress T cell proliferation to prevent inflammation [57]. Additionally, H2S is involved in bone metabolism and can slow down the aging process by inhibiting free radical reactions, activating Sirtuin 1 (SIRT1), and interacting with age-related gene Klotho [58–60]. While Glucocorticoids may impair endogenous H2S synthesis, supplementation of exogenous H2S can activate the Wnt signaling pathway to enhance bone formation and prevent osteoporosis [61]. The decline of H2S levels and its biosynthetic pathway are considered contributing factors to bone loss in estrogen-deficient mice [60].
Bile acids (BAs) are produced in the liver and further modified by GM, regulating lipid and bone metabolism [62]. Serum BA levels were positively correlated with bone density and negatively correlated with bone turnover markers, reflecting bone resorption. It is significantly lower in postmenopausal osteoporosis patients compared with healthy controls [63]. The G protein-coupled BA receptor 5 (TGR5) is involved in bone mass reduction and osteoblast differentiation [64]. BA-induced activation of TGR5 on small intestinal cells promotes the secretion of GLP-1 by enteroendocrine cells, which in turn promotes bone formation and inhibits bone resorption [65,66]. S-propargyl-cysteine (SPRC), an endogenous H2S donor, provides substrates for H2S synthesis, exhibits anti-inflammatory effects, and attenuates bone damage in rheumatoid arthritis. This effect is related to changes in GM composition, particularly the enrichment of bile salt hydrolase-producing bacteria, and BA metabolism [67].
Minerals, such as calcium, are vital for healthy aging. Calcium absorption in the small intestine is predominantly an ATP-dependent active process, accounting for approximately 90% of total calcium uptake. Postmenopausal women often experience a decline in calcium absorption, which possibly due to reduced active calcium transport or diffusion components of the calcium absorption system [68]. Supplements such as Astragalus polysaccharide, which are designed to repair the intestinal barrier, can restore intestinal function and alleviate osteoporosis by promoting osteoclast differentiation and reactivating the calcium signaling pathway [69].
Vitamin K, a fat-soluble nutrient, is present in two natural forms: phylloquinone (vitamin K1, PK), primarily obtained from vegetables, and menaquinones (vitamin K2, MKn), primarily synthesized by intestinal bacteria. This vitamin serves as a modulator of the GM composition and can be converted into various MKn forms by GM remodeling. Clinical evidence suggests that high vitamin K level can mitigate inflammation and inhibit abnormal calcification and mineralization linked to aging-related diseases. In contrast, vitamin K deficiency is linked to an increased risk of skeletal disorders in the elderly, such as osteoarthritis and osteoporosis [70]. In studies conducted on ovariectomized rats, MKn has been shown to enhance bone matrix quality and intestinal calcium absorption, thus preventing age-related bone loss [71]. Additionally, in obese mice, the bone-protective effects of tea polyphenol supplements were accompanied by changes in the composition and function of GM, specifically an increase in A. muciniphila abundance and enhancement of MKn biosynthesis pathways [72].
Vitamin D, despite its name, functions as a steroid hormone with two main forms: vitamin D2 (ergocalciferol), derived from plants, and vitamin D3 (cholecalciferol), synthesized from cholesterol [73]. It regulates calcium and phosphate homeostasis by facilitating calcium absorption in the intestine and influencing osteoblast and osteoclast activity [74]. As a member of the nuclear receptor superfamily, vitamin D receptor (VDR) mediates the effects of 1,25-dihydroxyvitamin D3 (1,25(OH)2D3), which is the active metabolite of vitamin D [75]. Due to the widespread expression of VDR in various cell types, particularly in the small intestine, the vitamin D/VDR signaling pathway not only regulates intestinal barrier and immune function but also modulates nutrient transport [76]. Studies have shown that mice lacking VDR specifically in the small intestine exhibit suppressed expression of Paneth cell-specific α-defensins, the converting enzyme matrix metalloproteinase 7 (MMP7), tight junction proteins, and MUC2. This leads to mucosal collapse, increased intestinal permeability, dysbiosis, and systemic inflammation [77]. Conversely, a high dietary vitamin D has been associated with lower intestinal permeability and stronger trabecular bone structure [78]. This suggests that vitamin D contributes to bone health in the elderly by improving intestinal barrier function.
Osteoimmunology examines the interaction between the immune and skeletal system, which is regulated by a suite of shared molecules including receptors, chemokines, cytokines, and transcription factors [79]. Transforming growth factor-β (TGF-β) and inflammatory stimulate the differentiation of immature T cells into Th17 cells--a subset of T lymphocytes implicated in osteoclastogenesis [80,81]. Th17 cells facilitate the upregulation of Receptor activator of nuclear factor-κB (RANK) ligand (RANKL), which interacts with RANK on osteoclast precursors, fostering their maturation and enhancing bone resorption [82]. Additionally, interleukin 17 (IL-17) released by Th17 cells directly increases osteoclastogenesis [83].
In murine model, Th17 cells are mainly produced in the lamina propria of the intestine, with their development being contingent upon segmented filamentous bacteria (SFB) [84,85]. SFB presence boosts IL-17α expression in the ileum and upregulates LCN2 in the liver and serum, both of which favor osteoclastogenesis while inhibiting osteoblastogenesis [86].
Aging-related factors and estrogen deficiency can downregulate epithelial binding proteins and increase intestinal permeability, facilitating microbial translocation from the lumen to the subepithelial space. This incites the production of pro-inflammatory cytokines by immune cell, precipitating the emergence of age-related pathologies [87,88]. A multiethnic longitudinal cohort study corroborates the association of increased intestinal permeability during menopause with heightened inflammation and reduced bone density [23]. For example, estrogen deficiency exacerbates intestinal permeability, enabling microbial components to activate T cells and boost TNF and IL-17 production in the lamina propria. Subsequently, TNF+T cells and Th17 cells egress from the intestine through an S1P receptor 1 (S1PR1)-dependent pathway, with TNF+T cells migrating to the bone marrow through the C-X-C motif chemokine receptor 3 (CXCR3) and Th17 cells use the C-C chemokine receptor 6 (CCR6)/chemokine (C-C motif) ligand 20 (CCL20, CCR6/CCL20) axis, leading to trabecular bone loss [89].
Reducing the GM dysbiosis and intestinal permeability may protect bone by inhibiting intestinal and bone marrow inflammation [90,91]. For example, polyphenols from betel nut seeds can increase lysozyme expression, maintain Paneth cell numbers, regulate the GM, modulate inflammatory responses, and ameliorate osteoporosis [92]. Probiotics can also restore the GM, promote intestinal barrier function, and equilibrate the balance between Th17 and Treg cells in the bone marrow, thereby guarding against bone loss under estrogen-deficient conditions [93]. Traditional herbal formulas like Xiong Fu powder can modulate the GM, enrich Lactobacillus abundance, and alleviate bone destruction. One potential mechanism involves the interaction between secretory Immunoglobulin A (IgA), regulated by intestinal mucosal Treg and Th17 cells and Lactobacillus adhesion [94].
Oxidative stress arises from an imbalance between antioxidants and reactive oxygen species (ROS), due to either excessive ROS production or insufficient antioxidants. Recognized as a hallmark of aging, oxidative stress is associated with age-related bone disorders. ROS changes, alongside shifts in antioxidant systems, contribute to bone loss and compromised bone quality by simultaneously modulating osteoclasts and osteoblasts. Excessive ROS typically activate signaling pathways such as mitogen-activated protein kinase (MAPK), phosphoinositide 3-kinase (PI3K), nuclear factor-κB (NF-κB), and Ca2+/Nuclear factor erythroid 2-related factor 2 (Ca2+/Nrf2), culminating in the activation of osteoclast-related genes like CTSK (Cathepsin K), MMP9 (matrix metalloproteinase 9), and NFATc1 [95]. Concurrently, oxidative stress disrupts bone formation by upregulating MAPK, releasing cytochrome C, and downregulating pathways such as Wnt/β-catenin, bone morphogenetic protein 2/Smad (BMP2/Smad), and focal adhesion kinase (FAK) phosphorylation, thereby inducing osteoblasts apoptosis [96]. The loss of sex hormones, such as estrogen or androgen, in aged individuals accelerates skeletal aging by diminishing oxidative stress defense and interfering with Wnt signaling [97,98].
Oxidative imbalances in the gut not only impede its own function but also affect overall health. While it can typically handle oxidative stress, its defensive capacity is overwhelmed by aging or heightened ROS levels. Oxidative stress-induced pathophysiological changes may impair calcium absorption by altering the expression and/or function of proteins integral to intracellular and/or intercellular Ca2+ transport [99,100].
Antioxidants have demonstrated potential in improving bone health by modulating the gut microenvironment [101,102]. For instance, Lactobacillus acidophilus (ATCC334) supplementation can enrich L. acidophilus abundance, stabilize redox balance, downregulate pro-inflammatory cytokine expression, and alleviate arthritis symptoms [103].
The current body of research on age-related bone diseases has largely concentrated on mechanisms related to telomere attrition, cellular apoptosis, immunosenescence, low-grade systemic inflammation, and Wnt signaling pathways [104–107]. Advances in research methodologies and omics technologies has deepened our comprehension of the gut microenvironment. The gut’s ability to rapidly respond to external stimuli is crucial for maintaining homeostasis, which is integral to overall health. Dysfunctions in intestinal barrier, which may lead to increased permeability and dysbiosis, could contribute to age-related osteoporosis by disrupting bone immune homeostasis. The gut environment’s influence on age-related bone diseases encompasses pathways related to metabolism, nutrient absorption, intestinal permeability, immunology, and oxidative stress. The potential for manipulating the gut microenvironment to prevent bone loss is a subject of ongoing research.
However, due to the high costs and technical limitations of sequencing and analytical methods, most studies on the intestinal microbiota and human health have relied on 16S rRNA amplicon sequencing. This approach provides only partial microbial species information, lacking gene-level and transcriptional insights, which hinders establishing a definitive causal link between the microbiota and health outcomes. Therefore, it is too early to conclude that assessments of the gut microenvironment can reliably reflect bone health. Furthermore, as the majority of current studies are preclinical and based on animal models, translating these findings to human clinical practice requires further rigorous and comprehensive efforts.
Acknowledgement: The authors thank Prof. Jianguo Liu for his useful suggestions.
Funding Statement: This study was supported by grant from the New Academic Talents Project of Zunyi Medical University (QKHPTRC [2018]5772-010), and Zunyi Oral Disease Immune Prevention and Medical Biomaterials Research and Development Innovation Talent Team, Zunyi Science Talent [2022] No. 1.
Author Contributions: Hu H and Yao YZ substantial contributions to the conception and design of the work, and wrote the manuscript. Huang Y, Liu FL and Wang Q revised it critically for important intellectual content. All authors contributed to manuscript revision, read, and approved the submitted version.
Availability of Data and Materials: Data sharing not applicable to this article as no datasets were generated or analyzed during the current study.
Ethics Approval: Not applicable.
Conflicts of Interest: The authors declare that they have no conflicts of interest to report regarding the present study.
References
1. Gacesa R, Kurilshikov A, Vich Vila A, Sinha T, Klaassen MAY, Bolte LA, et al. Environmental factors shaping the gut microbiome in a Dutch population. Nature. 2022;604(7907):732–9. [Google Scholar] [PubMed]
2. Guo J, Huang X, Dou L, Yan M, Shen T, Tang W, et al. Aging and aging-related diseases: from molecular mechanisms to interventions and treatments. Signal Transduct Target Ther. 2022;7(1):391. [Google Scholar] [PubMed]
3. Chandra A, Rajawat J. Skeletal aging and osteoporosis: mechanisms and therapeutics. Int J Mol Sci. 2021;22(7):3553. [Google Scholar] [PubMed]
4. Zioupos P, Kirchner HOK, Peterlik H. Ageing bone fractures: the case of a ductile to brittle transition that shifts with age. Bone. 2020;131:115176. [Google Scholar] [PubMed]
5. Ding P, Gao C, Gao Y, Liu D, Li H, Xu J, et al. Osteocytes regulate senescence of bone and bone marrow. eLife. 2022;11:e81480. [Google Scholar] [PubMed]
6. Lasorsa F, Rutigliano M, Milella M, Ferro M, Pandolfo SD, Crocetto F, et al. Cancer stem cells in renal cell carcinoma: origins and biomarkers. Int J Mol Sci. 2023;24(17):13179. [Google Scholar] [PubMed]
7. Sai B, Dai Y, Fan S, Wang F, Wang L, Li Z, et al. Cancer-educated mesenchymal stem cells promote the survival of cancer cells at primary and distant metastatic sites via the expansion of bone marrow-derived-PMN-MDSCs. Cell Death Dis. 2019;10(12):941. [Google Scholar] [PubMed]
8. Skov-Jeppesen K, Veedfald S, Madsbad S, Holst JJ, Rosenkilde MM, Hartmann B. Subcutaneous GIP and GLP-2 inhibit nightly bone resorption in postmenopausal women: a preliminary study. Bone. 2021;152:116065. [Google Scholar] [PubMed]
9. Helsted MM, Gasbjerg LS, Lanng AR, Bergmann NC, Stensen S, Hartmann B, et al. The role of endogenous GIP and GLP-1 in postprandial bone homeostasis. Bone. 2020;140:115553. [Google Scholar] [PubMed]
10. Nissen A, Christensen M, Knop FK, Vilsbøll T, Holst JJ, Hartmann B. Glucose-dependent insulinotropic polypeptide inhibits bone resorption in humans. J Clin Endocrinol Metab. 2014;99(11):E2325–9. [Google Scholar] [PubMed]
11. Abildgaard J, Ploug T, Pedersen AT, Eiken P, Pedersen BK, Holst JJ, et al. Preserved postprandial suppression of bone turnover markers, despite increased fasting levels, in postmenopausal women. Bone. 2021;143:115612. [Google Scholar] [PubMed]
12. Cheng Y, Liu P, Xiang Q, Liang J, Chen H, Zhang H, et al. Glucagon-like peptide-1 attenuates diabetes-associated osteoporosis in ZDF rat, possibly through the RAGE pathway. BMC Musculoskelet Disord. 2022;23(1):465. [Google Scholar] [PubMed]
13. Yadav VK, Ryu JH, Suda N, Tanaka KF, Gingrich JA, Schütz G, et al. Lrp5 controls bone formation by inhibiting serotonin synthesis in the duodenum. Cell. 2008;135(5):825–37. [Google Scholar] [PubMed]
14. Lavoie B, Lian JB, Mawe GM. Regulation of bone metabolism by serotonin. Adv Exp Med Biol. 2017;1033:35–46. [Google Scholar] [PubMed]
15. Fujita Y, Hashimoto K. Decreased bone mineral density in ovariectomized mice is ameliorated after subsequent repeated intermittent administration of (R)-ketamine, but not (S)-ketamine. Neuropsychopharmacol Rep. 2020;40(4):401–6. [Google Scholar] [PubMed]
16. Luo N, Mosialou I, Capulli M, Bisikirska B, Lin CS, Huang YY, et al. A neuronal action of sirtuin 1 suppresses bone mass in young and aging mice. J Clin Invest. 2022;132(23):e152868. [Google Scholar] [PubMed]
17. Wan X, Eguchi A, Fujita Y, Ma L, Wang X, Yang Y, et al. Effects of (R)-ketamine on reduced bone mineral density in ovariectomized mice: a role of gut microbiota. Neuropharmacology. 2022;213:109139. [Google Scholar] [PubMed]
18. Schepper JD, Collins F, Rios-Arce ND, Kang HJ, Schaefer L, Gardinier JD, et al. Involvement of the gut microbiota and barrier function in glucocorticoid-induced osteoporosis. J Bone Miner Res. 2020;35(4):801–20. [Google Scholar] [PubMed]
19. Egge N, Arneaud SLB, Wales P, Mihelakis M, McClendon J, Fonseca RS, et al. Age-onset phosphorylation of a minor actin variant promotes intestinal barrier dysfunction. Dev Cell. 2019;51(5):587–601.E7. [Google Scholar] [PubMed]
20. Moorefield EC, Andres SF, Blue RE, van Landeghem L, Mah AT, Santoro MA, et al. Aging effects on intestinal homeostasis associated with expansion and dysfunction of intestinal epithelial stem cells. Aging. 2017;9(8):1898–915. [Google Scholar] [PubMed]
21. He D, Wu H, Xiang J, Ruan X, Peng P, Ruan Y, et al. Gut stem cell aging is driven by mTORC1 via a p38 MAPK-p53 pathway. Nat Commun. 2020;11(1):37. [Google Scholar] [PubMed]
22. Pentinmikko N, Iqbal S, Mana M, Andersson S, Cognetta ABIII, Suciu RM, et al. Notum produced by Paneth cells attenuates regeneration of aged intestinal epithelium. Nature. 2019;571(7765):398–402. [Google Scholar] [PubMed]
23. Shieh A, Epeldegui M, Karlamangla AS, Greendale GA. Gut permeability, inflammation, and bone density across the menopause transition. JCI Insight. 2020;5(2):e134092. [Google Scholar] [PubMed]
24. Paone P, Cani PD. Mucus barrier, mucins and gut microbiota: the expected slimy partners? Gut. 2020;69(12):2232–43. [Google Scholar] [PubMed]
25. van der Post S, Jabbar KS, Birchenough G, Arike L, Akhtar N, Sjovall H, et al. Structural weakening of the colonic mucus barrier is an early event in ulcerative colitis pathogenesis. Gut. 2019;68(12):2142–51. [Google Scholar] [PubMed]
26. Fu J, Wei B, Wen T, Johansson ME, Liu X, Bradford E, et al. Loss of intestinal core 1-derived O-glycans causes spontaneous colitis in mice. J Clin Invest. 2011;121(4):1657–66. [Google Scholar] [PubMed]
27. Elderman M, Sovran B, Hugenholtz F, Graversen K, Huijskes M, Houtsma E, et al. The effect of age on the intestinal mucus thickness, microbiota composition and immunity in relation to sex in mice. PLoS One. 2017;12(9):e0184274. [Google Scholar] [PubMed]
28. Ragonnaud E, Biragyn A. Gut microbiota as the key controllers of “healthy” aging of elderly people. Immun Ageing. 2021;18(1):2. [Google Scholar] [PubMed]
29. Lim MY, Song EJ, Kang KS, Nam YD. Age-related compositional and functional changes in micro-pig gut microbiome. GeroScience. 2019;41(6):935–44. [Google Scholar] [PubMed]
30. Spychala MS, Venna VR, Jandzinski M, Doran SJ, Durgan DJ, Ganesh BP, et al. Age-related changes in the gut microbiota influence systemic inflammation and stroke outcome. Ann Neurol. 2018;84(1):23–36. [Google Scholar] [PubMed]
31. Lin X, Xiao HM, Liu HM, Lv WQ, Greenbaum J, Gong R, et al. Gut microbiota impacts bone via Bacteroides vulgatus-valeric acid-related pathways. Nat Commun. 2023;14(1):6853. [Google Scholar] [PubMed]
32. Liu JH, Chen CY, Liu ZZ, Luo ZW, Rao SS, Jin L, et al. Extracellular vesicles from child gut microbiota enter into bone to preserve bone mass and strength. Adv Sci. 2021;8(9):2004831. [Google Scholar]
33. Bárcena C, Valdés-Mas R, Mayoral P, Garabaya C, Durand S, Fernández-García F, et al. Healthspan and lifespan extension by fecal microbiota transplantation into progeroid mice. Nat Med. 2019;25(8):1234–42. [Google Scholar]
34. Liu A, Lv H, Wang H, Yang H, Li Y, Qian J. Aging increases the severity of colitis and the related changes to the gut barrier and gut microbiota in humans and mice. J Gerontol A Biol Sci Med Sci. 2020;75(7):1284–92. [Google Scholar] [PubMed]
35. Thevaranjan N, Puchta A, Schulz C, Naidoo A, Szamosi JC, Verschoor CP, et al. Age-associated microbial dysbiosis promotes intestinal permeability, systemic inflammation, and macrophage dysfunction. Cell Host Microbe. 2017;21(4):455–66.E4. [Google Scholar] [PubMed]
36. Hu H, Yao Y, Liu F, Luo L, Liu J, Wang X, et al. Integrated microbiome and metabolomics revealed the protective effect of baicalin on alveolar bone inflammatory resorption in aging. Phytomedicine. 2024;124:155233. [Google Scholar] [PubMed]
37. Donaldson DS, Shih BB, Mabbott NA. Aging-related impairments to M cells in Peyer’s patches coincide with disturbances to Paneth cells. Front Immunol. 2021;12:761949. [Google Scholar] [PubMed]
38. Hoffman RA, Huang S, Chalasani G, Vallejo AN. Disparate recruitment and retention of plasmacytoid dendritic cells to the small intestinal mucosa between young and aged mice. Aging Dis. 2021;12(5):1183–96. [Google Scholar] [PubMed]
39. Moretto MM, Lawlor EM, Khan IA. Aging mice exhibit a functional defect in mucosal dendritic cell response against an intracellular pathogen. J Immunol. 2008;181(11):7977–84. [Google Scholar] [PubMed]
40. Sovran B, Hugenholtz F, Elderman M, Van Beek AA, Graversen K, Huijskes M, et al. Age-associated impairment of the mucus barrier function is associated with profound changes in microbiota and immunity. Sci Rep. 2019;9(1):1437. [Google Scholar] [PubMed]
41. Dillon SM, Liu J, Purba CM, Christians AJ, Kibbie JJ, Castleman MJ, et al. Age-related alterations in human gut CD4 T cell phenotype, T helper cell frequencies, and functional responses to enteric bacteria. J Leukoc Biol. 2020;107(1):119–32. [Google Scholar] [PubMed]
42. Dillon SM, Thompson TA, Christians AJ, McCarter MD, Wilson CC. Reduced immune-regulatory molecule expression on human colonic memory CD4 T cells in older adults. Immun Ageing. 2021;18(1):6. [Google Scholar] [PubMed]
43. Ke K, Arra M, Abu-Amer Y. Mechanisms underlying bone loss associated with gut inflammation. Int J Mol Sci. 2019;20(24):6323. [Google Scholar] [PubMed]
44. Tanabe K, Nakamura S, Moriyama-Hashiguchi M, Kitajima M, Ejima H, Imori C, et al. Dietary fructooligosaccharide and glucomannan alter gut microbiota and improve bone metabolism in senescence-accelerated mouse. J Agric Food Chem. 2019;67(3):867–74. [Google Scholar] [PubMed]
45. Flint HJ, Duncan SH, Scott KP, Louis P. Links between diet, gut microbiota composition and gut metabolism. Proc Nutr Soc. 2015;74(1):13–22. [Google Scholar] [PubMed]
46. Blaak EE, Canfora EE, Theis S, Frost G, Groen AK, Mithieux G, et al. Short chain fatty acids in human gut and metabolic health. Benef Microbes. 2020;11(5):411–55. [Google Scholar] [PubMed]
47. Morrison DJ, Preston T. Formation of short chain fatty acids by the gut microbiota and their impact on human metabolism. Gut Microbes. 2016;7(3):189–200. [Google Scholar] [PubMed]
48. Lucas S, Omata Y, Hofmann J, Böttcher M, Iljazovic A, Sarter K, et al. Short-chain fatty acids regulate systemic bone mass and protect from pathological bone loss. Nat Commun. 2018;9(1):55. [Google Scholar] [PubMed]
49. Li B, Liu M, Wang Y, Gong S, Yao W, Li W, et al. Puerarin improves the bone micro-environment to inhibit OVX-induced osteoporosis via modulating SCFAs released by the gut microbiota and repairing intestinal mucosal integrity. Biomed Pharmacother. 2020;132:110923. [Google Scholar] [PubMed]
50. Lee CC, Liao YC, Lee MC, Lin KJ, Hsu HY, Chiou SY, et al. Lactobacillus plantarum TWK10 attenuates aging-associated muscle weakness, bone loss, and cognitive impairment by modulating the gut microbiome in mice. Front Nutr. 2021;8:708096. [Google Scholar] [PubMed]
51. Bekebrede AF, Keijer J, Gerrits WJJ, Boer VCJ. The molecular and physiological effects of protein-derived polyamines in the intestine. Nutrients. 2020;12(1):197. [Google Scholar] [PubMed]
52. Xuan M, Gu X, Li J, Huang D, Xue C, He Y. Polyamines: their significance for maintaining health and contributing to diseases. Cell Commun Signal. 2023;21(1):348. [Google Scholar] [PubMed]
53. Eisenberg T, Abdellatif M, Schroeder S, Primessnig U, Stekovic S, Pendl T, et al. Cardioprotection and lifespan extension by the natural polyamine spermidine. Nat Med. 2016;22(12):1428–38. [Google Scholar] [PubMed]
54. Soda K, Uemura T, Sanayama H, Igarashi K, Fukui T. Polyamine-rich diet elevates blood spermine levels and inhibits pro-inflammatory status: an interventional study. Med Sci. 2021;9(2):22. [Google Scholar]
55. Chevalier C, Kieser S, Çolakoğlu M, Hadadi N, Brun J, Rigo D, et al. Warmth prevents bone loss through the gut microbiota. Cell Metab. 2020;32(4):575–90.E7. [Google Scholar] [PubMed]
56. Yamada T, Park G, Node J, Ozaki K, Hiraiwa M, Kitaguchi Y, et al. Daily intake of polyamine-rich Saccharomyces cerevisiae S631 prevents osteoclastic activation and bone loss in ovariectomized mice. Food Sci Biotechnol. 2019;28(4):1241–5. [Google Scholar] [PubMed]
57. Donertas Ayaz B, Zubcevic J. Gut microbiota and neuroinflammation in pathogenesis of hypertension: a potential role for hydrogen sulfide. Pharmacol Res. 2020;153:104677. [Google Scholar] [PubMed]
58. Sun WH, Liu F, Chen Y, Zhu YC. Hydrogen sulfide decreases the levels of ROS by inhibiting mitochondrial complex IV and increasing SOD activities in cardiomyocytes under ischemia/reperfusion. Biochem Biophys Res Commun. 2012;421(2):164–9. [Google Scholar] [PubMed]
59. Yamamoto M, Clark JD, Pastor JV, Gurnani P, Nandi A, Kurosu H, et al. Regulation of oxidative stress by the anti-aging hormone klotho. J Biol Chem. 2005;280(45):38029–34. [Google Scholar] [PubMed]
60. Grassi F, Tyagi AM, Calvert JW, Gambari L, Walker LD, Yu M, et al. Hydrogen sulfide is a novel regulator of bone formation implicated in the bone loss induced by estrogen deficiency. J Bone Miner Res. 2016;31(5):949–63. [Google Scholar] [PubMed]
61. Ma J, Shi C, Liu Z, Han B, Guo L, Zhu L, et al. Hydrogen sulfide is a novel regulator implicated in glucocorticoids-inhibited bone formation. Aging. 2019;11(18):7537–52. [Google Scholar] [PubMed]
62. Perino A, Demagny H, Velazquez-Villegas L, Schoonjans K. Molecular physiology of bile acid signaling in health, disease, and aging. Physiol Rev. 2021;101(2):683–731. [Google Scholar] [PubMed]
63. Zhao YX, Song YW, Zhang L, Zheng FJ, Wang XM, Zhuang XH, et al. Association between bile acid metabolism and bone mineral density in postmenopausal women. Clinics. 2020;75:e1486. [Google Scholar] [PubMed]
64. Li Z, Huang J, Wang F, Li W, Wu X, Zhao C, et al. Dual targeting of bile acid receptor-1 (TGR5) and farnesoid X receptor (FXR) prevents estrogen-dependent bone loss in mice. J Bone Miner Res. 2019;34(4):765–76. [Google Scholar] [PubMed]
65. Pathak P, Xie C, Nichols RG, Ferrell JM, Boehme S, Krausz KW, et al. Intestine farnesoid X receptor agonist and the gut microbiota activate G-protein bile acid receptor-1 signaling to improve metabolism. Hepatology. 2018;68(4):1574–88. [Google Scholar] [PubMed]
66. Zhao C, Liang J, Yang Y, Yu M, Qu X. The impact of glucagon-like peptide-1 on bone metabolism and its possible mechanisms. Front Endocrinol. 2017;8:98. [Google Scholar]
67. Wang Z, Yu Y, Liao J, Hu W, Bian X, Wu J, et al. S-propargyl-cysteine remodels the gut microbiota to alleviate rheumatoid arthritis by regulating bile acid metabolism. Front Cell Infect Microbiol. 2021;11:670593. [Google Scholar] [PubMed]
68. Nordin BE, Need AG, Morris HA, O’Loughlin PD, Horowitz M. Effect of age on calcium absorption in postmenopausal women. Am J Clin Nutr. 2004;80(4):998–1002. [Google Scholar] [PubMed]
69. Liu J, Liu J, Liu L, Zhang G, Peng X. Reprogrammed intestinal functions in Astragalus polysaccharide-alleviated osteoporosis: combined analysis of transcriptomics and DNA methylomics demonstrates the significance of the gut-bone axis in treating osteoporosis. Food Funct. 2021;12(10):4458–70. [Google Scholar] [PubMed]
70. Shea MK, Kritchevsky SB, Hsu FC, Nevitt M, Booth SL, Kwoh CK, et al. The association between vitamin K status and knee osteoarthritis features in older adults: the health, aging and body composition study. Osteoarthr Cartil. 2015;23(3):370–8. [Google Scholar]
71. Kobayashi M, Hara K, Akiyama Y. Effects of vitamin K2 (menatetrenone) on calcium balance in ovariectomized rats. Jpn J Pharmacol. 2002;88(1):55–61. [Google Scholar] [PubMed]
72. Elmassry MM, Chung E, Cao JJ, Hamood AN, Shen CL. Osteoprotective effect of green tea polyphenols and annatto-extracted tocotrienol in obese mice is associated with enhanced microbiome vitamin K2 biosynthetic pathways. J Nutr Biochem. 2020;86:108492. [Google Scholar] [PubMed]
73. Demer LL, Hsu JJ, Tintut Y. Steroid hormone vitamin D. Circ Res. 2018;122(11):1576–85. [Google Scholar] [PubMed]
74. Gil Plaza-Diaz J, Mesa MD. Vitamin D: classic and novel actions. Ann Nutr Metab. 2018;72(2):87–95. [Google Scholar] [PubMed]
75. Li YC, Chen Y, Du J. Critical roles of intestinal epithelial vitamin D receptor signaling in controlling gut mucosal inflammation. J Steroid Biochem Mol Biol. 2015;148:179–83. [Google Scholar] [PubMed]
76. Zhang YG, Wu S, Sun J. Vitamin D, vitamin D receptor, and tissue barriers. Tissue Barriers. 2013;1(1):e23118. [Google Scholar] [PubMed]
77. Su D, Nie Y, Zhu A, Chen Z, Wu P, Zhang L, et al. Vitamin D signaling through induction of Paneth cell defensins maintains gut microbiota and improves metabolic disorders and hepatic steatosis in animal models. Front Physiol. 2016;7:498. [Google Scholar] [PubMed]
78. Villa CR, Chen J, Wen B, Sacco SM, Taibi A, Ward WE, et al. Maternal vitamin D beneficially programs metabolic, gut and bone health of mouse male offspring in an obesogenic environment. Int J Obes. 2016;40(12):1875–83. [Google Scholar]
79. Okamoto K, Takayanagi H. Osteoimmunology. Cold Spring Harb Perspect Med. 2019;9(1):a031245. [Google Scholar] [PubMed]
80. Sato K, Suematsu A, Okamoto K, Yamaguchi A, Morishita Y, Kadono Y, et al. Th17 functions as an osteoclastogenic helper T cell subset that links T cell activation and bone destruction. J Exp Med. 2006;203(12):2673–82. [Google Scholar] [PubMed]
81. Xia Y, Inoue K, Du Y, Baker SJ, Reddy EP, Greenblatt MB, et al. TGFβ reprograms TNF stimulation of macrophages towards a non-canonical pathway driving inflammatory osteoclastogenesis. Nat Commun. 2022;13(1):3920. [Google Scholar] [PubMed]
82. Chen S, Guo C, Wang R, Feng Z, Liu Z, Wu L, et al. Monocytic MDSCs skew Th17 cells toward a pro-osteoclastogenic phenotype and potentiate bone erosion in rheumatoid arthritis. Rheumatology. 2021;60(5):2409–20. [Google Scholar] [PubMed]
83. Bhadricha H, Patel V, Singh AK, Savardekar L, Patil A, Surve S, et al. Increased frequency of Th17 cells and IL-17 levels are associated with low bone mineral density in postmenopausal women. Sci Rep. 2021;11(1):16155. [Google Scholar] [PubMed]
84. Ivanov II, Atarashi K, Manel N, Brodie EL, Shima T, Karaoz U, et al. Induction of intestinal Th17 cells by segmented filamentous bacteria. Cell. 2009;139(3):485–98. [Google Scholar] [PubMed]
85. Brockmann L, Tran A, Huang Y, Edwards M, Ronda C, Wang HH, et al. Intestinal microbiota-specific Th17 cells possess regulatory properties and suppress effector T cells via c-MAF and IL-10. Immunity. 2023;56(12):2719–35.E7. doi:10.1016/j.immuni.2023.11.003. [Google Scholar] [PubMed] [CrossRef]
86. Hathaway-Schrader JD, Poulides NA, Carson MD, Kirkpatrick JE, Warner AJ, Swanson BA, et al. Specific commensal bacterium critically regulates gut microbiota osteoimmunomodulatory actions during normal postpubertal skeletal growth and maturation. JBMR Plus. 2020;4(3):e10338. [Google Scholar] [PubMed]
87. Riaz Rajoka MS, Zhao H, Li N, Lu Y, Lian Z, Shao D, et al. Origination, change, and modulation of geriatric disease-related gut microbiota during life. Appl Microbiol Biotechnol. 2018;102(19):8275–89. [Google Scholar] [PubMed]
88. Wang N, Ma S, Fu L. Gut microbiota dysbiosis as one cause of osteoporosis by impairing intestinal barrier function. Calcif Tissue Int. 2022;110(2):225–35. [Google Scholar] [PubMed]
89. Yu M, Pal S, Paterson CW, Li JY, Tyagi AM, Adams J, et al. Ovariectomy induces bone loss via microbial-dependent trafficking of intestinal TNF+ T cells and Th17 cells. J Clin Invest. 2021;131(4):e143137. [Google Scholar]
90. Li JY, Chassaing B, Tyagi AM, Vaccaro C, Luo T, Adams J, et al. Sex steroid deficiency-associated bone loss is microbiota dependent and prevented by probiotics. J Clin Invest. 2016;126(6):2049–63. [Google Scholar] [PubMed]
91. Schepper JD, Collins FL, Rios-Arce ND, Raehtz S, Schaefer L, Gardinier JD, et al. Probiotic Lactobacillus reuteri prevents postantibiotic bone loss by reducing intestinal dysbiosis and preventing barrier disruption. J Bone Miner Res. 2019;34(4):681–98. [Google Scholar] [PubMed]
92. Mei F, Meng K, Gu Z, Yun Y, Zhang W, Zhang C, et al. Arecanut (Areca catechu L.) seed polyphenol-ameliorated osteoporosis by altering gut microbiome via LYZ and the immune system in estrogen-deficient rats. J Agric Food Chem. 2021;69(1):246–58. [Google Scholar] [PubMed]
93. Jia L, Tu Y, Jia X, Du Q, Zheng X, Yuan Q, et al. Probiotics ameliorate alveolar bone loss by regulating gut microbiota. Cell Prolif. 2021;54(7):e13075. [Google Scholar] [PubMed]
94. Xi X, Ye Q, Li X, Lu X, Fan D, Xia Y, et al. Xiong Fu powder regulates the intestinal microenvironment to protect bones against destruction in collagen-induced arthritis rat models. Front Cell Infect Microbiol. 2022;12:854940. [Google Scholar] [PubMed]
95. Teitelbaum SL, Ross FP. Genetic regulation of osteoclast development and function. Nat Rev Genet. 2003;4(8):638–49. [Google Scholar] [PubMed]
96. Tao H, Ge G, Liang X, Zhang W, Sun H, Li M, et al. ROS signaling cascades: dual regulations for osteoclast and osteoblast. Acta Biochim Biophys Sin. 2020;52(10):1055–62. [Google Scholar] [PubMed]
97. Almeida M, Han L, Martin-Millan M, Plotkin LI, Stewart SA, Roberson PK, et al. Skeletal involution by age-associated oxidative stress and its acceleration by loss of sex steroids. J Biol Chem. 2007;282(37):27285–97. [Google Scholar] [PubMed]
98. Lean JM, Jagger CJ, Kirstein B, Fuller K, Chambers TJ. Hydrogen peroxide is essential for estrogen-deficiency bone loss and osteoclast formation. Endocrinology. 2005;146(2):728–35. [Google Scholar] [PubMed]
99. Diaz de Barboza G, Guizzardi S, Moine L, Tolosa de Talamoni N. Oxidative stress, antioxidants and intestinal calcium absorption. World J Gastroenterol. 2017;23(16):2841–53. [Google Scholar] [PubMed]
100. Walters JR, Balesaria S, Chavele KM, Taylor V, Berry JL, Khair U, et al. Calcium channel TRPV6 expression in human duodenum: different relationships to the vitamin D system and aging in men and women. J Bone Miner Res. 2006;21(11):1770–7. [Google Scholar] [PubMed]
101. Domazetovic V, Marcucci G, Iantomasi T, Brandi ML, Vincenzini MT. Oxidative stress in bone remodeling: role of antioxidants. Clin Cases Miner Bone Metab. 2017;14(2):209–16. [Google Scholar] [PubMed]
102. Wang Y, Wu Y, Wang Y, Xu H, Mei X, Yu D, et al. Antioxidant properties of probiotic bacteria. Nutrients. 2017;9(5):521. [Google Scholar] [PubMed]
103. Pan H, Guo R, Ju Y, Wang Q, Zhu J, Xie Y, et al. A single bacterium restores the microbiome dysbiosis to protect bones from destruction in a rat model of rheumatoid arthritis. Microbiome. 2019;7(1):107. [Google Scholar] [PubMed]
104. Colmegna I, Weyand CM. Haematopoietic stem and progenitor cells in rheumatoid arthritis. Rheumatology. 2011;50(2):252–60. [Google Scholar] [PubMed]
105. Fragkiadaki P, Nikitovic D, Kalliantasi K, Sarandi E, Thanasoula M, Stivaktakis PD, et al. Telomere length and telomerase activity in osteoporosis and osteoarthritis. Exp Ther Med. 2020;19(3):1626–32. [Google Scholar] [PubMed]
106. Lu H, Jia C, Wu D, Jin H, Lin Z, Pan J, et al. Fibroblast growth factor 21 (FGF21) alleviates senescence, apoptosis, and extracellular matrix degradation in osteoarthritis via the SIRT1-mTOR signaling pathway. Cell Death Dis. 2021;12(10):865. [Google Scholar] [PubMed]
107. Chen D, Xie R, Shu B, Landay AL, Wei C, Reiser J, et al. Wnt signaling in bone, kidney, intestine, and adipose tissue and interorgan interaction in aging. Ann NY Acad Sci. 2019;1442(1):48–60. [Google Scholar] [PubMed]
Cite This Article
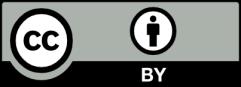
This work is licensed under a Creative Commons Attribution 4.0 International License , which permits unrestricted use, distribution, and reproduction in any medium, provided the original work is properly cited.