Open Access
REVIEW
Estrogen-related receptor alpha: A novel perspective on skeletal, muscular, and vascular systems
1 Department of Orthopedics, Shengjing Hospital of China Medical University, Shenyang, China
2 Department of Orthopedics, Fuxin Central Hospital, Fuxin, China
* Corresponding Author: YE TIAN. Email:
(This article belongs to the Special Issue: Subcellular Organelles and Cellular Molecules: Localization, Detection, Prediction, and Diseases)
BIOCELL 2024, 48(2), 191-203. https://doi.org/10.32604/biocell.2023.045349
Received 24 August 2023; Accepted 06 December 2023; Issue published 23 February 2024
Abstract
Estrogen-related receptor alpha can significantly affect cell metabolism and play key regulatory roles in healthy and diseased organisms. ERRα is also related to the onset and progression of various cancer types. ERRα is primarily expressed in metabolically active tissues and regulates the transcription of metabolic genes in such tissues. It coordinates metabolism and energy demand, affects osteoblasts, osteoclasts, and chondrocytes, promotes muscle regeneration, participates in angiogenesis, and regulates cell aging. In this study, the literature related to the identification of ERRα in skeletal, muscular, and vascular systems was reviewed to further elucidate this receptor.Keywords
Nuclear receptors (NRs) are transcription factors that contain ligand-dependent molecules and orphan NRs. Natural ligands of NRs have not been identified. The earliest orphan NR identified was the estrogen-related receptor (ERR), which comprises three members (ERRα, ERRβ, and ERRγ, also known as Estrogen-related receptor alpha, ESRRA, NR3B1; ESRRB, NR3B2; and ESRRG, NR3B3, respectively) [1]. Although ERR is highly similar to ER, ERR does not bind to estrogen. Nevertheless, ERRα is involved in the estrogen regulatory pathway [2].
ERRα has numerous physiological functions with several different downstream effects (Fig. 1). Gene transcription analysis in mice revealed that ERRα was expressed in various organs [3]. Currently, ERRα is reported to critically affect cellular metabolism, general growth and development, and skeletal homeostasis [4]. ERRα is involved in tumor cell-associated metabolic processes. The function of ERRα in tumorigenesis has been elucidated through prior reports; furthermore, ERRα expression in tumorigenesis has a close relationship with tumor prognosis [5,6]. Reportedly, ERRα exerts certain regulatory effects on normal physiology and bone development [7,8]. It is involved in the regulation of osteocytes, osteoclasts (OCs), and chondrocytes, and consequently promotes the onset and progression of various diseases, including osteoporosis and osteoarthritis (OA). The involvement of ERRα in muscle differentiation has been reported previously [9–11]. The adult skeletal muscle is a relatively plastic tissue that may undergo changes under physical conditions. NR is an important regulator of skeletal muscle function, which is achieved through transcription factors. Previously, studies have investigated the physiological and metabolic functions of ERRα. However, these functions require further elucidation. Because ERRα has not been specifically reported in muscle, blood vessels, and bone, recent insights were summarized in this study to provide valuable guidance for further research.
Figure 1: ERRα regulates the abnormal proliferation of VSMC and causes vascular stenosis. ERRα regulates muscle strength and the aging and regeneration of skeletal muscles. ERRα regulates osteoporosis and chondrocytes. Some agonists and antagonists of ERRα.
Structure and function of ERRα
Structurally, ERRα appears to be similar to human ERR DNA-binding region sequence. based on this characteristic, novel steroid receptors have been identified via a cDNA library method [2]. An unknown protein, ERRα, with conserved steroid receptors, was identified in cDNA libraries of the kidney and heart. Notably, certain synthetic compounds can regulate the transcriptional activity of ERR [12–14], with a large proportion of these compounds inhibiting ERRα transcriptional activity.
Although DNA-binding regions of ERR and ER were reportedly similar (68%), other proteins—including the ligand and E region—only appeared to have a moderate similarity degree (36%). Thus, it is evident that ERRα cannot bind to estrogen [15]. However, ERRα is reported to have a certain effect on the estrogen pathway [16,17].
ERR presents the typical structural features of NR (Fig. 2) [18]. These include the AF-1 domain, DNA-binding domain (DBD), ligand binding domain (LBD), and AF-2 domain. The N-terminal contains the AF-1 domain, which enables weak transcriptional activation in a ligand-independent manner. NR proteins have several conserved regions, including a centrally located DBD and a C-terminal ligand-binding region. The latter is known to interact with transcriptional coregulators. ERR is localized primarily in the nucleus, which can facilitate the identification of the ERR response factors on or beyond the target gene promoter and the regulation of downstream target genes.
Figure 2: ERRα and ERα have the same structural features and domain homology, including the AF-1 domain, DNA-binding domain (DBD), ligand binding domain (LBD), and AF-2 domain.
A recent study has reported extensive crosstalk between peroxisome proliferator-activated receptor γ coactivator 1α (PGC-1α) and ERRα, and the two proteins can influence the transcriptional activities of one another [18]. ERRα regulates the inflammatory response of macrophages. As part of its action mechanism, ERRα directly acts on the promoter region of the deoxygenase Tnfaip3 on the Toll-like receptor (TLR) signaling pathway. Furthermore, ERRα regulates TLR-induced inflammatory response [19]. The transcriptional coactivator family of PGC-1 integrates multiple pathways involving numerous NRs and non-NRs to activate fatty acid oxidation, mitochondria, glucose uptake, and gluconeogenesis in various tissues [20]. Reportedly, PGC-1α is considered a highly efficient ERRα coactivator, which regulates a variety of metabolic genes through synergy [21,22]. Similarly, thyroid hormone (TH) increases the expression of PGC-1α in the liver, which consequently upregulated ERRα expression, thereby promoting the TH-induced mitochondrial activity. ERRα expression can be regulated by estrogen receptor (ER) agonists or antagonists. ERRα can bind to multiple ER response units and recruit ERs similar to those recruited by coregulators, thereby mimicking ER-mediated gene expression [23].
ERRα plays regulatory roles at transcriptional levels, and the effect of microRNA (miR) on ERRα mRNA has been reported. ERRα expression decreased as a result of the action of miR-125a in the 3′ UTR sequence [24]. Additionally, ERRα has an inhibitory effect [25]. MiR-125a is reported to affect ERRα expression. Other microRNAs (miR-137, miR-497, and miR-135a) can target conserved sequences in the 3′ UTR [26–28]. In addition, miRs can inhibit tumor cell proliferation, cell migration, and extracellular matrix invasion.
Additionally, post-translational modifications can regulate ERRα activity. Protein stability is maintained through proteasome-dependent processes and ubiquitination. Parkin enables the expression of the ERR receptor in dopaminergic neurons and ERRα degradation in vascular endothelial cells [29,30]. Physically, ERRα interacts with the histone demethylase lysine-specific demethylase1 (LSD1), which consequently transforms the receptor’s function from transcriptional repression to transcriptional activation [31]. LSD1 protects the receptor from ubiquitination and proteasome-dependent degradation, regardless of its enzymatic activity [32]. Although the mechanism of this protective effect requires further clarification, this mechanism may facilitate the efficient activation of the target of ERR-LSD1. ERRα can be phosphorylated via the following two pathways: the cAMP/PKA-dependent pathway in lung cells [33] and the EGF/MEK pathway in colorectal cancer cells [34]. Moreover, this synergistic effect upregulates ERRα expression, thereby increasing the activity of the surfactant A promoter and accelerating tumor growth. The involvement of these signaling pathways in ERRα mRNA is currently unclear. Thus, ERRα phosphorylation is assumed to be responsible for the stabilization of these pathways.
ERRα and skeletal muscle diseases
ERRα regulates bone and muscle growth. The expression of ERRα in humans is critical for the body’s metabolism and physiology [35]. Additionally, ERRα expression in bones and cartilage of limbs and the trunk may be critical for the regulation of the bone metabolism balance [36,37].
Skeletal muscle, the largest tissue in the human body, plays a pivotal role in the body’s metabolism and exercise. Many mitochondrial function genes related to skeletal muscle aging were expressed based on the results of a public gene database analysis [38]. Polymerase chain reaction (PCR) results showed a significant decrease in the expression level of ERRα in the skeletal muscle of aged rats. In addition, the gastrocnemius muscle of rats showed significant differences between age groups in terms of ERRα expression, and the expression showed a tendency to decrease with increasing age [39]. ERR is an important transcription factor related to mitochondrial energy metabolism in the skeletal muscle aging process, and it is expected to become a new drug for muscle atrophy in older adults in the future. ERRα plays a key role in the regulation of oxidation and oxidative stress. The basic metabolic oxidative capacity of the ERRα deletion group was reported to be significantly decreased. Moreover, a significant decrease in its activity was observed [40]. Ahn et al. [41] reported that the body mass of the rats in the administration group did not change significantly. However, their endurance and grasping abilities were increased, which was attributed to the expression of myocyte enhancer-binding factor 2 (MEF2) transcription factors, such as PGC-1α and ERRα, thereby improving the effect of oxidative muscle fibers. The results showed that ERRα played a role in enhancing muscle strength. In adult muscle tissues, ERRα expression increases with exercise, consequently promoting mitochondria-driven oxidative remodeling [42].
Skeletal muscle aging in older adults considerably affects their quality of life. A previous study has reported that long-term accumulation of reactive oxygen species may cause damage to the structure and function of skeletal muscle mitochondria, thereby leading to mitochondrial damage [43]. In recent years, however, some scholars have opposed this concept [44]. Currently, the imbalance between mitochondrial energy metabolism and calcium balance has garnered increasing attention [45–47]. ERRα regulates the expression of more than 100 genes in mitochondrial electron transport [48]. ERRα is one of the key factors that affect the aging of skeletal muscle cells and plays a key role in ATP synthesis [49–51].
Mature skeletal muscles can independently recover after exposure to injury from daily activities and external stress. Regeneration of skeletal muscle is a necessary process for the human body to maintain the normal quality and function of the muscle, thereby meeting various diastolic and operational needs. ERRα regulates mitochondrial synthesis, glucose, and fatty acid oxidation in skeletal muscle cells. Controlling the oxidative capacity of mitochondria is an important step in maintaining normal muscle fiber regeneration during muscle regeneration [52]. LaBarge et al. [53] observed that muscle cell regeneration was impaired by constructing a muscle-specific ERRα−/−(M-ERRα−/−) mouse model. This mouse model showed that muscle fibers are shrunk and that cells clump together. Additionally, the decrease in mitochondrial biogenesis factors was associated with mitochondrial oxidative repair damage in M-ERR. The AMPK pathway is involved in the regeneration of normal muscle and the expression of ERRα. However, increased expression of AMPK during early regeneration may affect muscle fiber growth. Therefore, the delayed recovery of myofibers in the M-ERRα−/− muscle may be caused by increased AMPK activation. In summary, AMPK can regulate mitochondria by activating the ERRα pathway and promoting muscle regeneration. In contrast, in patients with facioscapulohumeral muscular dystrophy (FSHD), PGC-1α is an important cofactor of ERRα. Banerji et al. [54] reported the morphological and associated transcriptional changes of FSHD myogenesis. They reported that the inhibition of PGC-1α can inhibit the expression of ERRα in FSHD, which affects muscle differentiation and thus causes muscle atrophy. Furthermore, Sjögren et al. [55] reported that the regulatory effect of PGC-1α on the Branched-chain amino acid (BCAA) gene in human muscle tubes is related to ERRα. The PGC-1α-ERRα pathway may rescue myogenically differentiated cell lines from FSHD by adjusting nutrients—such as streptomycin A, flavonols, and genistein—to increase muscle diameter. The novel research results discussed above indicated that regulating the PGC1-α-ERR pathway can improve muscle strength in patients with FSHD and accelerate its repair and regeneration. PGC-1 is an important coactivator of ERRα, which cooperates with numerous metabolic genes. ERRα, as an important transcriptional activator, plays an important role in the oxidative process of the heart and skeletal muscles, which is consistent with the role of PGC-1α. Additionally, this observation was consistent with the functional interaction of ERRα with PGC-1α, which is co-expressed with ERRα in these tissues.
Large amounts of lipid deposition can lead to cardiovascular diseases, and exposure of the liver and pancreas to large amounts of lipid causes lipid accumulation. In muscles, fatty acid oxidation (FAO) and oxidative phosphorylation (OXPHOS) are key steps in fatty acid depletion. ERRα regulates the genes for FAO and OXPHOS in muscle. Kitamura et al. [56] treated the C2C12 mouse muscle cell line with 50 µM daidzein. FAO and OXPHOS genes were reported to be significantly upregulated, whereas ERRα may have been partially blocked. This indicated that soybean sapogenins regulate FAO and OXPHOS genes at least partially through the ERRα pathway, thereby reducing lipid deposition in muscle cells. Daidzein is reported to be involved in regulating ERRα, which consequently prevents various muscle lipotoxicity-caused diseases. Recently, resveratrol, a natural polyphenol contained in red wine, was shown to ameliorate high-fat diet-induced cardiomyopathy in an ERRα-dependent manner [57].
In summary, ERRα showed positive effects on skeletal muscle regeneration, angiogenesis, muscle strength, and improvement in aging status (Fig. 3).
Figure 3: Functions of ERRα in skeletal muscle diseases. ERRα regulates FAO and OXPHOS. ERRα regulates the regeneration of skeletal muscle. ERRα enhances muscle strength and regulates skeletal muscle aging. ERRα regulates lipid deposition. ERRα ameliorates cardiomyopathy. ERRα activates skeletal muscle angiogenesis.
ERRα and vascular-related diseases
To the best of our knowledge, effective treatments for peripheral arterial disease (PAD) and critical limb ischemia (CLI) are currently unavailable. Furthermore, mechanisms underlying intramuscular angiogenesis remain poorly understood. Sopariwala et al. [58] used a skeletal muscle-specific ERRα transgenic mouse model and reported that ERRα is a hypoxia-stimulating factor. Additionally, ERRα overexpression activates the angiogenic gene program in skeletal muscle, increases the distribution of blood vessels in skeletal muscles, promotes ischemic angiogenesis and vascular regeneration in skeletal muscles, and restores the blood supply of ischemic muscle. Therefore, in skeletal muscles, ERRα may be involved in muscle angiogenesis and can alleviate vascular complications caused by ischemic diseases including diabetes.
Metabolically active tissues such as skeletal muscles can regulate vascular density, thereby satisfying metabolic demands. However, the molecular mechanisms that coordinate these processes remain poorly understood. Oxygen and carbon sources reach the tissues via the vascular system as part of aerobic metabolism. According to the findings reported by a new study, ERRα overexpression in skeletal muscle promoted revascularization, characterized by increased capillary staining and muscle perfusion in diet-induced obese (DIO) mice after hindlimb ischemic injury. ERRα activation can promote ischemic revascularization and muscle recovery in obesity [59]. Transcriptional coactivator 1β (PGC-1β) reportedly induces vascular endothelial growth factor (VEGF) production, a process that requires the involvement of ERRα [60]. Baicalein is involved in angiogenesis through the baicalin-induced VEGF expression via activation of the ERRα pathway [61]. In addition, ERRα is involved in the regulation of vascular development during sinus follicle formation and affects angiogenesis [62].
Physical activity has a variety of beneficial effects on the human body through the metabolic, musculoskeletal, and nervous systems [63]. Furthermore, exercise increases serum levels of beta-aminoisobutyric acid (BAIBA), a compound that reduces insulin resistance and inflammation in skeletal muscle, induces the browning of white adipose tissues, and promotes fatty acid oxidation in the liver. In vitro experiments revealed that BAIBA has a significant promoting effect on vascular endothelium and vascular endothelial cells, increases the expression of transcription factors including ERRα, and plays a role via PGC-1β-ERRα/PPAR-δ and PPAR-γ pathways [64].
Oxidative stress is reported to be a key contributor to vascular endothelial dysfunction and may result from an imbalance between antioxidant capacity and active substances [65]. Expression of ERRα was detectable in vascular endothelial cells (EC). However, the other two ERR isoforms—i.e., ERRβ and ERRγ—were nearly undetectable [66]. Notably, 17β-estradiol (E2)-induced ERRα expression enhanced fatty acid uptake/oxidation, increased mitochondrial replication and ATP production, and reduced formation of reactive oxygen species (ROS). Furthermore, E2-induced ERRα expression regulated fatty acid metabolism and reduced circulating lipids via endothelial cells. In mice on a high-fat diet, overexpression of ERRα in the vessel wall normalized the increase in plasma lipids induced by E2 deficiency, thereby ameliorating vascular injury [67].
The abnormal proliferation of vascular smooth muscle cells (VSMCs) was closely associated with the onset and progression of cardiovascular diseases. Abnormal migration and proliferation of vascular smooth muscle cells may lead to vascular restenosis [68]. Therefore, inhibiting the proliferation and migration of vascular smooth muscle cells is an effective strategy to help prevent cardiovascular diseases. The results revealed that XCT790 could reduce the expression of the ERRα gene, consequently inhibiting the ERK signaling pathway, thereby inhibiting VSMC proliferation [69]. The signal transduction of RhoA/p27Kip1 and β-Catenin/Wnt4 is related to the proliferation and migration of VSMCs. XCT790 significantly inhibited the proliferation, phenotypic transformation, and migration of rat RASMCs, whereas the reduction in ERRα inhibited the formation of RASMCs, cyclin transcription, and Rb hyperphosphorylation. Finally, ERRα exerts a significant inhibitory effect on the neovascularization of rat angiogenesis [70]. Therefore, ERRα has the potential to act as a new therapy.
In conclusion, ERRα can promote angiogenesis and improve vascular damage through various pathways, making it a new therapy.
Owing to the progressively aging population worldwide, osteoporosis has become a major public health concern, particularly in postmenopausal women. Osteoblasts (OBs) and OCs are the two main types of bone cells that coordinate with each other to maintain bone homeostasis. Bone formation in OB is impaired with aging due to a decrease in the number and activity of OB. Conversely, in OC, bone resorption increases and osteoporosis develops when bone resorption exceeds bone formation. Osteoporosis is a metabolic disease, and inhibition of OC differentiation may be a viable treatment direction for osteoporosis.
Notably, the expression levels of ERRα are particularly high in bone cells at all developmental stages, i.e., from the earliest precursor cells to the most mature OB in mineralized nodules. Additionally, the 23-bp nucleotide repeat polymorphism of the ERRα gene may be associated with bone mineral density [71]. Additionally, ERRα is reported to inhibit OB development and bone formation [72,73]. Inhibition of ERRα in primary rat cranial (RC) cells prevents differentiation of RC cells into mature OB and significantly reduces the number of mineralized bone nodules, whereas ERRα overexpression enhances differentiation, thereby affecting multiple stages of OB development [73].
ERRα can activate or inhibit Wnt signaling depending on the presence or absence of PGC-1α; furthermore, coactivators, including ERRα and PGC-1α, are novel regulators of the Wnt signaling pathway during OB differentiation [74]. Wnt pathway regulation is cell-intrinsic and does not affect β-linked protein nuclear translocation. However, the regulation of this pathway is dependent on the DNA binding of ERRα. Both knockdown and activation of ERRα in C3H10T1/2 cells regulate osteogenic differentiation via a DNA binding-dependent mechanism, and expression of active ERRα correlates with the effects of OB differentiation in the Wnt pathway. Female ERRα knockout mice are reportedly resistant to bone loss, suggesting that ERRα is an inhibitor of OB differentiation in vivo [75]. ERRα has also been reported to exert a certain induction effect on mesenchymal stem cells derived from periodontal tissue in vitro [73]. OBs are differentiated from mesenchymal stem cells (MSCs) [76,77]. In these cells, ERRα and Glutaminase (Gls) levels are elevated during the osteogenic induction of human MSCs [78]. The signal transduction pathway of ERRα/Gls plays a key role in this process. Therefore, the increase in ERRα in rat bone marrow mesenchymal stem cells can remarkably promote the expression of Gls, increase the consumption of glutamine (Gln), and rescue the osteogenic ability. ERRα knockout can counteract oophorectomy-caused bone loss. However, it cannot regulate natural aging-caused bone loss [79].
ERRα is dynamically regulated during OC differentiation, and MYC (a broadly acting transcription factor) regulates OC production [80] and consequently bone remodeling through ERRα modulation. In addition, ERRα is associated with OC adhesion, migration, and invasion [81,82]. ERRα deficiency reportedly disrupts OC differentiation and inhibits bone resorption [83]. In vivo studies have reported that bone mineral density in ERRα knockout female mice does not experience an age-related decrease [73,84], which is associated with increased bone formation. However, bone resorption is unaltered, and the amount and activity of OC remain unchanged [85], highlighting that the effect is mediated by OB rather than OC [86].
ERRα is involved in regulating OC differentiation and the response to rosiglitazone, a synthetic PPAR γ agonist, thereby causing bone loss [87]. PPAR γ can activate OC formation and inhibit OB formation, indicating that rosiglitazone can reduce bone formation while maintaining or increasing bone resorption. Notably, PPARγ induces the expression of ERRα. After the ERRα-mediated release of PGC1β, the dependent mechanism induces mitochondrial gene expression to promote OC formation. Furthermore, a polymorphic autoregulatory hormone response element on the human ERRα promoter was associated with bone mineral density. These findings not only identify ERRα as a critical regulator of skeletal and mineral homeostasis but also highlight a functional association between the PPARγ and ERRα pathways, converging at the transcriptional coactivator PGC1β. Therefore, rosiglitazone stimulates the formation of OCs and bone resorption via a transcriptional network comprised of ERRα, PPARγ, and PGC1β.
ERRα-knockout cells and mice cannot respond to statins, bisphosphonates, and cholesterol [88]. Thus, ERRα is involved in the regulation of the cholesterol pathway. Statins are reported to reduce osteoporosis by lowering cholesterol. Cholesterol oxidation products (COPs) are important compounds that maintain bone metabolism homeostasis. COPs participate in many vital biological processes, such as the differentiation of MSCs, bone formation in OB, and bone absorption in OC. Recent research confirmed that the effect of specific COPs on MSCs the promotion of OB production and inhibition of adipocyte production [89]. Cholesterol is known to affect ERRα, and the evidence of this activity was derived from the investigation of the bone calcium production process. Additionally, the luciferase report proved that cholesterol can regulate the transcriptional activity of ERRα [90]. ERRα-knockout mice exhibited a decrease in bone resorption and an increase in bone mass, highlighting that ERRα can promote OC differentiation. OC function inhibition is achieved through the administration of statins and nitrogen-containing bisphosphonates. These drugs can inhibit cholesterol biosynthesis and reduce cholesterol accumulation by blocking HMG-CoA reductase and farnesyl diphosphate synthesis (FPPS), respectively [91,92]. However, in the absence of ERRα, OC differentiation is not inhibited by statins or enhanced by cholesterol. These observations highlighted the role of cholesterol as an ERRα agonist involved in OC formation. Cholesterol can also enhance ERR α in OCs and PGC1β. Their interaction subsequently promotes OC formation and bone absorption. Bisphosphonates can negatively regulate OC-mediated bone absorption in patients with cancer. Thus the application of bisphosphonates to protect the bones of patients with cancer is particularly widespread [93].
Sterol regulatory factor-2 (SREBP2) is a vital class of transcription factors that can regulate cholesterol synthesis and OC differentiation [94,95]. Yao et al. [96] applied carnosic acid (CA) to a murine monocyte line and revealed that CA inhibited SREBP2 activity and OC formation, directly bound to ERRα, and promoted its degradation. These findings demonstrated the excellent anti-osteoporotic effects of CA. In addition, Huang et al. found that andrographolide interfered with OC differentiation as an ERRα inverse agonist and could be used to prevent physiological and pathological bone loss [97].
The abovementioned point highlights the critical involvement of ERRα in regulating the function of OB and OC to maintain bone homeostasis. Researchers have speculated that ERRα may be regulated to treat bone metabolic diseases such as osteoporosis and osteosclerosis. Furthermore, CA and andrographolide exhibited favorable anti-osteoporosis effects through ERRα.
OA is a well-known arthritic disease that primarily involves chronic inflammation of the articular cartilage [98] and causes pathological alterations in the synovium, meniscus, and subpatellar fat pad with low-grade inflammation [99,100]. Research on OA has shifted from being considered a “wear and tear” disease to a “metabolic” disease [101,102]. Cellular senescence, inflammatory cytokines, metalloproteinases, estrogens, and biomechanical imbalances play a critical role in OA progression and may lead to a series of key pathological alterations [103,104], ERRα is expressed in human and murine articular chondrocytes and is dysregulated in inflammatory arthritis, which is more commonly observed in older populations [10]. Therefore, ERRα can be considered an important regulator of cellular senescence [105] and may be involved in chondrocyte senescence, thereby promoting OA progression [106].
The effect of ERRα on cartilage is primarily related to the regulation of Sox9. This gene is involved in the proliferation, differentiation, and maturation of chondrocytes; additionally, Sox9 is a key regulator of cartilage differentiation and cartilage formation [107–109]. Regulation of ERRα contributes to the maturation of proliferating chondrocytes to hypertrophy, which may be attributed to direct or indirect regulation of Sox9 by ERRα [110].
ERRα overexpression in C518 cells increased the expression of cytoskeletal transcription factor Sox9 in the SRY-type high mobility group, which is an important factor leading to chondrogenesis [111]. In addition, ERRα plays a vital role in the early proliferation and differentiation of mandibular condyle chondrocytes and exerts a certain regulatory effect on the Sox9 gene; additionally, ERRα exerts a certain promotion effect on the proliferation of mandibular condyle chondrocytes [112]. The ERRα inverse activator XCT790 can inhibit the Sox9 expression in human OA chondrocytes, thereby inhibiting its interaction with PGC-1α. This then leads to inhibition of the expression of Sox9 in cartilage tissue, which regulates Sox9 expression together with ERRα and PGC-1α, thereby confirming the presence of a synergistic effect of the two on cartilage formation and maintenance [112,113].
Interleukin-1β (IL-1β) is an inflammatory factor closely associated with OA pathogenesis [114,115], which may be related to the pathological process of OA. IL-1β can significantly increase the expression of ERRα in OA chondrocytes in vitro, whereas the ERRα-mediated degradation of cartilage is associated with IL-1β and matrix metalloproteinase-13 (MMP-13) [116].
XCT790 reduced the upregulation of ERRα by Sox9 and IL-1β and decreased the level of MMP-13 mRNA in a dose-dependent manner, highlighting that ERRα regulates itself through the IL-1β pathway and promotes cartilage formation [113]. Furthermore, the abovementioned report found that statins can inhibit IL-1β-induced articular chondrocyte senescence as well as MMP production [117]. They also increase mRNA levels of bone morphogenetic protein, aggregated proteoglycan, and type II collagen, maintain chondrocyte longevity, inhibit catabolic factor-induced matrix-degrading enzyme production, and prevent OA cartilage degeneration.
Agonists and antagonists of ERRα
Despite previously unavailable natural ligands for ERRα, recent studies have shown that endogenous cholesterol may be a potential ligand for ERRα [118,119]. However, it is inconclusive whether cholesterol and other related substances are natural endogenous ligands of ERRα [120]. In addition, statins enhanced hepatic gluconeogenesis by modulating PCK1, which was negatively regulated by ERRα [121,122]. Although statins can have excitatory effects in vitro, their actual effects in the body are much more complicated than ERRα. This complex mechanism warrants a considerable degree of research for verification.
In addition to several plant-derived compounds (apigenin, resveratrol, rutaecarpine, and flavone), genistein, forskolin, and phorbol 12-myristate13-acetate(3MA) are agonists of ERRα and ERRα-PPARGC1A dimers [123–126]. Certain signaling modulators, such as PMAs and herbal compounds, including apigenin, resveratrol, and rutin, are the most potent ERRα activators.
The expression of ERRα in breast cancer is associated with its poor prognosis. The potential applications of ERRα in breast cancer are evident. LingH2-10 is a novel selective ERRα inversion activator, and its action mechanism involves the reduction of pyruvate dehydrogenase kinase 4 (PDK4), osteopontin, and presenilin-2 (pS2), thereby inhibiting TNBC proliferation [127]. Compound 11 has a significant inhibitory effect on the transcriptional regulation of ERRα, and its action mechanism is to regulate the signaling pathway downstream of the receptor, thereby infiltrating and transferring the ER-negative MDA-MB-231 cell line. The results showed that compound 11 was a highly effective ERRα inverse activator [128].
XCT790 has been considered a specific inverse agonist of ERRα [129]. shRNA or XCT790 induces a remarkable degree of apoptosis in myeloma cell line MM. 1S cells by interfering with ERRα expression [130], inhibits pancreatic cancer (PC) progression by inhibiting ERRα and MEK/ERK signaling pathway [131], suppresses ERRα activity, and causes considerable accumulation of triglyceride present in rat hepatocytes, thereby reducing triglyceride secretion in the culture medium and regulating triglyceride metabolism [132]. Rotenone and aminopterin appear to be more effective than XCT790 in inhibiting ERR and PGC/ERR signaling pathways [124].
Owing to the synergistic relationship between ERRα and PGC-1α, Lynch et al. [133] performed an ERR/PGC antagonist assay to compare the ERR antagonist activity of each compound in the presence and absence of the cofactor PGC-1α. Artemisinin, carfilzomib, bortezomib, and showed antagonistic effects in the ERR/PGC assay, implying that these compounds inhibit ERR via PGC-1α. In addition, SAHA and etoposide showed antagonistic activity in the ERR assay but agonist activity in the ERR/PGC assay. Lynch et al. [133] also identified five antitumor drugs (artemisinin, methichlorophen, bortezomib, carfilzomib, and gimerticam) and nine pesticides (acriflavine, berberine, chlormidazole, fluoxastrobin, picoxystrobin, proflavin, pyridaben, rotenone, and trifloxystrobin) as ERRα antagonists. Additionally, these nine insecticides inhibit and/or control pests by adversely affecting the metabolism and mitochondrial function in multiple ways [134–136]. The activity of ERRα and PGC-1α decreased after exposure to these pesticides [133].
Recently, food products like genistein, apigenin, resveratrol, rutin, piceatannol, daidzein, flavonoids, and cholesterol are potential cholesterol-promoting substances [119,137,138]. In addition, certain pesticides (acriflavine, imidazole, pyridaben, and fluridone), antineoplastic drugs (artemisinin, bortezomib, etoposide, and vorinostat), and other compounds (rotenone, camptothecin, toxic carotene, papaverine, staurosporine, and progesterone) appear to be ERRα antagonists or ERRα/peroxisome proliferator-activated receptor gamma coactivator 1alpha (PPARGC1A) antagonists or an antagonist of both [123,139] Although the aforementioned compounds have been developed in clinical trials, information regarding their roles is lacking in normal physiological conditions and diseases [140–151]. Among the above compounds, the classification of ERRα will no longer be orphan NR. According to the impact of ERRα on pathogenesis, the use of ERR molecules to modulate the disease research provides a new biological basis for preventing and treating the disease (Tables 1 and 2).
As a typical member of the orphan receptor, ERRα has the typical structural features of NR and is an important transcription factor. ERRα has numerous physiological functions,critically affect cellular metabolism, general growth and development, and skeletal homeostasis. The data reported in the present study suggest that ERRα plays a regulatory role in osteoporosis, OA, skeletal muscle diseases, vascular diseases, and other diseases. Previously, it was believed that ERRα has no natural ligand, but happily, Several ERRα receptor agonists and antagonists are already used in clinical trials, The ultimate goal could be to utilize the transcriptional advantages of ERRα to prevent and treat human diseases, such as vascular and skeletal, metabolic, and other disorders.
Acknowledgement: None.
Funding Statement: This work was supported by the National Natural Science Foundation of China (Grant No. 81970760), the Natural Science Foundation of Liaoning Province (Grant No. 2021-MS-201), and the 345 Talent Project of Shengjing Hospital.
Author Contributions: The authors confirm contribution to the paper as follows: study conception and design: Lei Wang; data collection: Lei Wang, Zhi-hang Wang, Nian-ping Cao, Bobo Chen, Chong-jun Huang; draft manuscript preparation: Lei Wang, Zhi-hang Wang, Nian-ping Cao, Bobo Chen, Chong-jun Huang, Lei Yang, Ye Tian. All authors reviewed the results and approved the final version of the manuscript.
Availability of Data and Materials: Data sharing not applicable to this article as no datasets were generated or analyzed during the current study.
Ethics Approval: Not applicable.
Conflicts of Interest: The authors declare that they have no conflicts of interest to report regarding the present study.
References
1. Benoit G, Cooney A, Giguere V, Ingraham H, Lazar M, Muscat G, et al. International union of pharmacology. LXVI. Orphan nuclear receptors. Pharmacol Rev. 2006;58:798–836. doi:10.1124/pr.58.4.10. [Google Scholar] [PubMed] [CrossRef]
2. Tanida T. Molecular dynamics of estrogen-related receptors and their regulatory proteins: roles in transcriptional control for endocrine and metabolic signaling. Anat Sci Int. 2022;97:15–29. doi:10.1007/s12565-021-00634-7. [Google Scholar] [PubMed] [CrossRef]
3. Huss JM, Garbacz WG, Xie W. Constitutive activities of estrogen-related receptors: transcriptional regulation of metabolism by the ERR pathways in health and disease. BBA Biomembr. 2015;1852(9):1912–27. doi:10.1016/j.bbadis.2015.06.016. [Google Scholar] [PubMed] [CrossRef]
4. Thouennon E, Delfosse V, Bailly R, Blanc P, Boulahtouf A, Grimaldi M, et al. Insights into the activation mechanism of human estrogen-related receptor gamma by environmental endocrine disruptors. J Cell Mol Life. 2019;76:4769–81. [Google Scholar]
5. Sakellakis M. Orphan receptors in prostate cancer. Prostate. 2022;82(10):1016–24. doi:10.1002/pros.24370. [Google Scholar] [PubMed] [CrossRef]
6. Frodyma DE, Troia TC, Rao C, Svoboda RA, Berg JA, Shinde DD, et al. PGC-1β and ERRα promote glutamine metabolism and colorectal cancer survival via transcriptional upregulation of PCK2. Cancers. 2022;14(19):4879. doi:10.3390/cancers14194879. [Google Scholar] [PubMed] [CrossRef]
7. Feng C, Xu ZW, Tang XJ, Cao HF, Zhang GL, Tan JW. Estrogen-related receptor α: a significant regulator and promising target in bone homeostasis and bone metastasis. Mol. 2022;27(13):3976. doi:10.3390/molecules27133976. [Google Scholar] [PubMed] [CrossRef]
8. Cerutti C, Shi JR, Vanacker JM. Multifaceted transcriptional network of estrogen-related receptor alpha in health and disease. Int J Mol Sci. 2023;24(5):4265. doi:10.3390/ijms24054265. [Google Scholar] [PubMed] [CrossRef]
9. Nelson ER, Wardell SE, McDonnell DP. The molecular mechanisms underlying the pharmacological actions of estrogens, SERMs and oxysterols: implications for the treatment and prevention of osteoporosis. Bone. 2013;53(51):42–50. doi:10.1016/j.bone.2012.11.011. [Google Scholar] [PubMed] [CrossRef]
10. Bonnelye E, Aubin JE. An energetic orphan in an endocrine tissue: a revised perspective of the function of estrogen receptor-related receptor alpha in bone and cartilage. J Bone Miner Res. 2013;28(2):225–33. doi:10.1002/jbmr.1836. [Google Scholar] [PubMed] [CrossRef]
11. Carnesecchi J, Vanacker JM. Estrogen-related receptors and the control of bone cell fate. Mol Cell Endocrinol. 2016;432:37–43. doi:10.1016/j.mce.2015.07.019. [Google Scholar] [PubMed] [CrossRef]
12. Karnati KR, Wang YX, Du YL. Exploring the binding mode thermodynamics of inverse agonists against estrogen-related receptor alpha. RSC Adv. 2020;10(28):16659–68. doi:10.1039/C9RA10697A. [Google Scholar] [PubMed] [CrossRef]
13. He S, Xiao H, Luo S, Li X, Zhang JD, Ren XM, et al. Benzotriazole ultraviolet stabilizers promote breast cancer cell proliferation via activating estrogen-related receptors α and γ at human-relevant levels. Environ Sci Technol. 2022;56(4):2466–75. doi:10.1021/acs.est.1c03446. [Google Scholar] [PubMed] [CrossRef]
14. Zhang K, Zhu S, Li JM, Jiang TT, Feng L, Pei JP, et al. Targeting autophagy using small-molecule compounds to improve potential therapy of Parkinson’s disease. Acta Pharm Sin B. 2021;11(10):3015–34. doi:10.1016/j.apsb.2021.02.016. [Google Scholar] [PubMed] [CrossRef]
15. Hong H, Yang L, Stallcup MR. Hormone-independent transcriptional activation and coactivator binding by novel orphan nuclear receptor ERR3. Biol Chem. 1999;74(32):22618–26. doi:10.1074/jbc.274.32.22618. [Google Scholar] [PubMed] [CrossRef]
16. Bonnelye E, Aubin JE. Estrogen receptor-related receptor α: a mediator of estrogen response in bone. JCEM. 2005;90(5):3115–21. doi:10.1210/jc.2004-2168. [Google Scholar] [PubMed] [CrossRef]
17. Zhang ZP, Chen K, Shih JC, Teng CT. Estrogen-related receptors-stimulated monoamine oxidase B promoter activity is down-regulated by estrogen receptors. J Mol Endocrinol. 2006;20(7):1547–61. doi:10.1210/me.2005-0252. [Google Scholar] [PubMed] [CrossRef]
18. Laganière J, Tremblay GB, Dufour CR, Giroux S, Rousseau F, Giguère V. A polymorphic autoregulatory hormone response element in the human estrogen-related receptor α (ERRα) promoter dictates peroxisome proliferator-activated receptor γ coactivator-1α control of ERRα expression. Bioelectrochem. 2004;279(18):18504–10. doi:10.1074/jbc.M313543200. [Google Scholar] [PubMed] [CrossRef]
19. Yuk JM, Kim TS, Kim SY, Lee HM, Han J, Dufour CR, et al. Orphan nuclear receptor ERRα controls macrophage metabolic signaling and A20 expression to negatively regulate TLR-induced inflammation. Immunity. 2015;43(1):80–91. doi:10.1016/j.immuni.2015.07.003. [Google Scholar] [PubMed] [CrossRef]
20. Scarpulla RC. Metabolic control of mitochondrial biogenesis through the PGC-1 family regulatory network. Biochim Biophys Acta. 2011;1813:1269–78. doi:10.1016/j.bbamcr.2010.09.019. [Google Scholar] [PubMed] [CrossRef]
21. Ramjiawan A, Bagchi RA, Albak L, Czubryt MP. Mechanism of cardiomyocyte PGC-1α gene regulation by ERRα. Biochem Mol Biol. 2013;91(3):148–54. doi:10.1139/bcb-2012-0080. [Google Scholar] [PubMed] [CrossRef]
22. Brown EL, Foletta VC, Wright CR, Sepulveda PV, Konstantopoulos N, Sanigorski A, et al. PGC-1α and PGC-1β increase protein synthesis via ERRα in C2C12 myotubes. Front Physiol. 2018;25:1336. doi:10.3389/fphys.2018.01336. [Google Scholar] [PubMed] [CrossRef]
23. Li Y, Birnbaumer L, Teng CT. Regulation of ERRα gene expression by estrogen receptor agonists and antagonists in SKBR3 breast cancer cells: diferential molecular mechanisms mediated by g protein-coupled receptor GPR30/GPER-1. Mol Endocrinol. 2010;24(5):969–80. doi:10.1210/me.2009-0148. [Google Scholar] [PubMed] [CrossRef]
24. Ji HL, Song CC, Li YF, He JJ, Li YL, Zheng XL, et al. miR-125a inhibits porcine preadipocytes diferentiation by targeting ERRα. Mol Cell Biochem. 2014;395(1–2):155–65. doi:10.1007/s11010-014-2121-4. [Google Scholar] [PubMed] [CrossRef]
25. Carnesecchi J, Vanacker JM. The estrogen-related receptors and the adipocyte. Horm Mol Biol Clin I. 2013;14(3):107–12. doi:10.1515/hmbci-2013-0020. [Google Scholar] [PubMed] [CrossRef]
26. Zhao YY, Li YP, Lou GY, Zhao L, Xu ZZ, Zhang Y, et al. MiR137 targets estrogen-related receptor alpha and impairs the proliferative and migratory capacity of breast cancer cells. PLoS One. 2012;7(6):e39102. doi:10.1371/journal.pone.0039102. [Google Scholar] [PubMed] [CrossRef]
27. Han L, Liu B, Jiang LX, Liu JY, Han SM. MicroRNA-497 downregulation contributes to cell proliferation, migration, and invasion of estrogen receptor alpha negative breast cancer by targeting estrogen-related receptor alpha. Biol. 2016;37(10):13205–14. doi:10.1007/s13277-016-5200-1. [Google Scholar] [PubMed] [CrossRef]
28. Tribollet V, Barenton B, Kroiss A, Vincent S, Zhang L, Forcet C, et al. miR-135a inhibits the invasion of cancer cells via suppression of ERRα. PLoS One. 2016;11(5):e0156445. doi:10.1371/journal.pone.0156445. [Google Scholar] [PubMed] [CrossRef]
29. Ren Y, Jiang H, Ma D, Nakaso K, Feng J. Parkin degrades estrogen-related receptors to limit the expression of monoamine oxidases. Hum Mol Genet. 2011;20(6):1074–83. doi:10.1093/hmg/ddq550. [Google Scholar] [PubMed] [CrossRef]
30. Xia WW, Yin J, Zhang SP, Guo CC, Li YY, Zhang Y, et al. Parkin modulates ERRα/eNOS signaling pathway in endothelial cells. Cell Physiol Biochem. 2018;49(6):2022–34. doi:10.1159/000493713. [Google Scholar] [PubMed] [CrossRef]
31. Carnesecchi J, Forcet C, Zhang L, Tribollet V, Barenton B, Boudra R, et al. ERRα induces H3K9 demethylation by LSD1 to promote cell invasion. Proc Natl Acad Sci USA. 2017;114(15):3909–14. doi:10.1073/pnas.1614664114. [Google Scholar] [PubMed] [CrossRef]
32. Carnesecchi J, Cerutti C, Vanacker JM, Forcet C. ERRα protein is stabilized by LSD1 in a demethylation-independent manner. PLoS One. 2017;12(11):e0188871. doi:10.1371/journal.pone.0188871. [Google Scholar] [PubMed] [CrossRef]
33. Liu D, Benlhabib H, Mendelson CR. cAMP enhances estrogen-related receptor α (ERRα) transcriptional activity at the SP-A promoter by increasing its interaction with protein kinase A and steroid receptor coactivator 2 (SRC-2). Mol Endocrinol. 2009;23(6):772–83. doi:10.1210/me.2008-0282. [Google Scholar] [PubMed] [CrossRef]
34. Zhou S, Xia HW, Xu HJ, Tang QL, Nie OZ, Gong QY, et al. ERRα suppression enhances the cytotoxicity of the MEK inhibitor trametinib against colon cancer cells. J Exp Clin Cancer Res. 2018;37(1):218. doi:10.1186/s13046-018-0862-8. [Google Scholar] [PubMed] [CrossRef]
35. Fischer A, Bardakci F, Sellner M, Lill MA, Smieško M. Ligand pathways in estrogen-related receptors. J Biomol Struct. 2023;41(5):1639–48. doi:10.1080/07391102.2022.2027818. [Google Scholar] [PubMed] [CrossRef]
36. Bouchet M, Lainé A, Boyault C, Proponnet-Guerault M, Meugnier E, Bouazza L, et al. ERRα expression in bone metastases leads to an exacerbated antitumor immune response. Cancer Res. 2020;80(13):2914–26. doi:10.1158/0008-5472.CAN-19-3584. [Google Scholar] [PubMed] [CrossRef]
37. Marie JC, Bonnelye E. Effects of estrogens on osteoimmunology: a role in bone metastasis. Front Immunol. 2022;23(13):899104. doi:10.3389/fimmu.2022.899104. [Google Scholar] [PubMed] [CrossRef]
38. Kan JB, Hu YF, Ge YQ, Zhang WS, Lu S, Zhao CP, et al. Declined expressions of vast mitochondria-related genes represented by CYCS and transcription factor ESRRA in skeletal muscle aging. Bioeng. 2021;12(1):3485–3502. doi:10.1080/21655979.2021.1948951. [Google Scholar] [PubMed] [CrossRef]
39. So WK, Kim HK, Chen YX, Jeong SH, Yeung PKK, Chow BCK, et al. Exchange protein directly activated by cAMP (Epac) 1 plays an essential role in stress-induced exercise capacity by regulating PGC-1α and fatty acid metabolism in skeletal muscle. Pflug Arch Eur J Phy. 2020;472(2):195–216. doi:10.1007/s00424-019-02344-6. [Google Scholar] [PubMed] [CrossRef]
40. Perry MC, Dufour CR, Tam IS, B’chir W, Giguère V. Estrogen-related receptor alpha coordinates transcriptional programs essential for exercise tolerance and muscle fitness. Mol Endocrinol. 2014;28:2060–71. doi:10.1210/me.2014-1281. [Google Scholar] [PubMed] [CrossRef]
41. Ahn JS, Son HJ, Seo HD, Ha TY, Ahn JY, Lee HJ, et al. γ-oryzanol improves exercise endurance and muscle strength by upregulating PPARδ and ERRγ activity in aged mice. Mol Nutr Food Res. 2021;65(14):e2000652. doi:10.1002/mnfr.202000652. [Google Scholar] [PubMed] [CrossRef]
42. Fan WW, He NH, Lin CS, Wei Z, Hah N, Waizenegger W, et al. ERRγ promotes angiogenesis, mitochondrial biogenesis, and oxidative remodeling in PGC1α/β-deficient muscle. Cell Rep. 2018;22(10):2521–9. doi:10.1016/j.celrep.2018.02.047. [Google Scholar] [PubMed] [CrossRef]
43. Umanskaya A, Santulli G, Je Xi W, Andersson DC, Reiken SR, Marks AR. Genetically enhancing mitochondrial antioxidant activity improves muscle function in aging. Proc Natl Acad Sci USA. 2014;111(42):15250–5. doi:10.1073/pnas.1412754111. [Google Scholar] [PubMed] [CrossRef]
44. Hood DA, Memme JM, Oliveira AN, Triolo M. Maintenance of skeletal muscle mitochondria in health, exercise, and aging. Annu Rev Physiol. 2019;81(1):19–41. doi:10.1146/annurev-physiol-020518-114310. [Google Scholar] [PubMed] [CrossRef]
45. Pénélope AA, Marcus PJ, Maxime RH, Johan A, Chris R, Geert JG, et al. Mitochondrial function is impaired in the skeletal muscle of pre-frail elderly. Sci Rep. 2018;8(1):8548. doi:10.1038/s41598-018-26944-x. [Google Scholar] [PubMed] [CrossRef]
46. Dzik KP, Kaczor JJ. Mechanisms of vitamin D on skeletal muscle function: oxidative stress, energy metabolism and anabolic state. Eur J Appl Physiol. 2019;119(4):825–39. doi:10.1007/s00421-019-04104-x. [Google Scholar] [PubMed] [CrossRef]
47. Bravo-Sagua R, Parra V, Muñoz-Cordova F, Sanchez-Aguilera P, Garrido V, Contreras-Ferrat A, et al. Sarcoplasmic reticulum and calcium signaling in muscle cells: homeostasis and disease. Int Rev Cel Mol Biol. 2020;350:197–264. doi:10.1016/bs.ircmb.2019.12.007. [Google Scholar] [PubMed] [CrossRef]
48. Xia H, Dufour CR, Giguère V. ERRα as a bridge between transcription and function: role in liver metabolism and disease. Front Endocrinol. 2019;10:206. doi:10.3389/fendo.2019.00206. [Google Scholar] [PubMed] [CrossRef]
49. Tsushida K, Tanabe K, Masuda K, Tanimura S, Miyake H, Arata Y, et al. Estrogen-related receptor α is essential for maintaining mitochondrial integrity in cisplatin-induced acute kidney injury. Biochem Biophys Res Commun. 2018;498(4):918–24. doi:10.1016/j.bbrc.2018.03.080. [Google Scholar] [PubMed] [CrossRef]
50. Singh BK, Sinha RA, Tripathi M, Mendoza A, Ohba KJ, Jann ACS, et al. Thyroid hormone receptor and ERRα coordinately regulate mitochondrial fission, mitophagy, biogenesis, and function. Sci Signal. 2018;11(536):eaam5855. doi:10.1126/scisignal.aam5855. [Google Scholar] [PubMed] [CrossRef]
51. Billon C, Sitaula S, Banerjee S, Welch R, Elgendy B, Hegazy H, et al. Synthetic ERRα/β/γ agonist induces an ERRα-dependent acute aerobic exercise response and enhances exercise capacity. ACS Chem Biol. 2022;18(4):756–71. doi:10.1021/acschembio.2c00720. [Google Scholar] [PubMed] [CrossRef]
52. Ovchinnikov AN, Paoli A, Seleznev VV, Deryugina AV. Royal jelly plus coenzyme Q10 supplementation improves high-intensity interval exercise performance via changes in plasmatic and salivary biomarkers of oxidative stress and muscle damage in swimmers: a randomized, double-blind, placebo-controlled pilot trial. J Int Soc Sports Nutr. 2022;19(1):239–57. doi:10.1080/15502783.2022.2086015. [Google Scholar] [PubMed] [CrossRef]
53. LaBarge S, McDonald M, Smith-Powell L, Auwerx J, Huss JM. Estrogen-related receptor-α (ERRα) deficiency in skeletal muscle impairs regeneration in response to injury. FASEB J. 2013;28(3):1082–97. doi:10.1096/fj.13-229211. [Google Scholar] [PubMed] [CrossRef]
54. Banerji CRS, Panamarova M, Pruller J, Figeac N, Hebaishi H, Fidanis E, et al. Dynamic transcriptomic analysis reveals suppression of PGC1α/ERRα drives perturbed myogenesis in facioscapulohumeral muscular dystrophy. Hum Mol Genet. 2019;28(8):1244–59. doi:10.1093/hmg/ddy405. [Google Scholar] [PubMed] [CrossRef]
55. Sjögren RJO, Rizo-Roca D, Chibalin AV, Chorell E, Furrer R, Katayama S, et al. Branched-chain amino acid metabolism is regulated by ERRα in primary human myotubes and is further impaired by glucose loading in type 2 diabetes. Diabetol. 2021;64(9):2077–91. doi:10.1007/s00125-021-05481-9. [Google Scholar] [PubMed] [CrossRef]
56. Kitamura K, Erlangga JS, Tsukamoto S, Sakamoto Y, Mabashi-Asazuma H, Iida K. Daidzein promotes the expression of oxidative phosphorylation-and fatty acid oxidation-related genes via an estrogen-related receptor α pathway to decrease lipid accumulation in muscle cells. J Nutr Biochem. 2020;77:108315. doi:10.1016/j.jnutbio.2019.108315. [Google Scholar] [PubMed] [CrossRef]
57. Song YJ, Zhong CB, Wu W. Resveratrol and diabetic cardiomyopathy: focusing on the protective signaling mechanisms. Oxid Med Cell Longev. 2020;2020:7051845. doi:10.1155/2020/7051845. [Google Scholar] [PubMed] [CrossRef]
58. Sopariwala DH, Likhite N, Pei G, Haroon F, Lin L, Yadav V, et al. Estrogen-related receptor α is involved in angiogenesis and skeletal muscle revascularization in hindlimb ischemia. FASEB J. 2021;35(5):e21480. doi:10.1096/fj.202001794RR. [Google Scholar] [PubMed] [CrossRef]
59. Sopariwala DH, Rios AS, Park MK, Song MS, Kumar A, Narkar VA. Estrogen-related receptor alpha is an AMPK-regulated factor that promotes ischemic muscle revascularization and recovery in diet-induced obese mice. FASEB Bioadv. 2022;4(9):602–18. doi:10.1096/fba.2022-00015. [Google Scholar] [PubMed] [CrossRef]
60. Rowe GC, Jang C, Patten IS, Arany Z. PGC-1β regulates angiogenesis in skeletal muscle. Am J Physiol Endocrinol Metab. 2011;301(1):E155–63. doi:10.1152/ajpendo.00681.2010. [Google Scholar] [PubMed] [CrossRef]
61. Zhang KQ, Lu JM, Mori T, Smith-Powell L, Synold TW, Chen S, et al. Baicalin increases VEGF expression and angiogenesis by activating the ERRα/PGC-1α pathway. Cardiovasc Res. 2011;89(2):426–35. doi:10.1093/cvr/cvq296. [Google Scholar] [PubMed] [CrossRef]
62. Blair SN, Kohl HW, Barlow CE, Paffenbarger Jr, Gibbons LW, Macera CA. Changes in physical fitness and all-cause mortality. A prospective study of healthy and unhealthy men. JAMA. 1995;273(14):1093–8. doi:10.1001/jama.1995.03520380029031. [Google Scholar] [CrossRef]
63. Sawada M, Yamamoto H, Ogasahara A, Tanaka Y, Kihara S. β-aminoisobutyric acid protects against vascular inflammation through PGC-1β-induced antioxidative properties. Biochem Biophys Res Commun. 2019;516(3):963–8. doi:10.1016/j.bbrc.2019.06.141. [Google Scholar] [PubMed] [CrossRef]
64. Guzmán A, Hughes CHK, Murphy BD. Orphan nuclear receptors in angiogenesis and follicular development. Reprod. 2021;162(3):R35–54. doi:10.1530/REP-21-0118. [Google Scholar] [PubMed] [CrossRef]
65. Steven S, Frenis K, Oelze M, Kalinovic S, Kuntic M, Jimenez MEB, et al. Vascular inflammation and oxidative stress: major triggers for cardiovascular disease. Oxid Med Cell Longev. 2019;23:7092151. doi:10.1155/2019/7092151. [Google Scholar] [PubMed] [CrossRef]
66. Likhite N, Yadav V, Milliman EJ, Sopariwala DH, Lorca S, Narayana NP, et al. Loss of estrogen-related receptor alpha facilitates angiogenesis in endothelial cells. Mol Cell Biol. 2019;39:e00411–8. doi:10.1128/MCB.00411-18. [Google Scholar] [PubMed] [CrossRef]
67. Li HW, Liu ZY, Gou YL, Yu HB, Siminelakis S, Wang S, et al. Estradiol mediates vasculoprotection via ERRα-dependent regulation of lipid and ROS metabolism in the endothelium. J Mol Cell Cardiol. 2015;87:92–101. doi:10.1016/j.yjmcc.2015.08.008. [Google Scholar] [PubMed] [CrossRef]
68. Ghasempour G, Mohammadi A, Zamani-Garmsiri F, Najafi M. miRNAs through β-ARR2/p-ERK1/2 pathway regulate the VSMC proliferation and migration. Life Sci. 2021;15:119703. doi:10.1016/j.lfs.2021.119703. [Google Scholar] [PubMed] [CrossRef]
69. Lu YH, Li QY, Chen L, Shi XJ. XCT790 inhibits rat vascular smooth muscle cells proliferation through down-regulating the expression of estrogen-related receptor alpha. Yao Xue Xue Bao. 2014;49(2):190–7 (In Chinese). [Google Scholar] [PubMed]
70. Li QY, Zhu L, Zhang LD, Chen HF, Zhu YF, Du YL, et al. Inhibition of estrogen related receptor α attenuates vascular smooth muscle cell proliferation and migration by regulating RhoA/p27Kip1 and β-Catenin/Wnt4 signaling pathway. Eur J Pharmacol. 2017;799(15):188–95. doi:10.1016/j.ejphar.2017.02.020. [Google Scholar] [PubMed] [CrossRef]
71. Shoukry A, Shalaby SM, Etewa RL, Ahmed HS, Abdelrahmanl HM. Association of estrogen receptor β and estrogen-related receptor α gene polymorphisms with bone mineral density in postmenopausal women. Mol Cell Biochem. 2005;405(1–2):23–31. doi:10.1007/s11010-015-2391-5. [Google Scholar] [PubMed] [CrossRef]
72. Gallet M, Vanacker JM. ERR receptors as potential targets in osteoporosis. Trends Endocrinol Metab. 2010;21(10):637–41. doi:10.1016/j.tem.2010.06.008. [Google Scholar] [PubMed] [CrossRef]
73. Teyssier C, Gallet M, Rabier B, Monfoulet L, Dine J, Macari C, et al. Absence of ERRα in female mice confers resistance to bone loss induced by age or estrogen-deficiency. PLoS One. 2009;4(11):e7942. doi:10.1371/journal.pone.0007942. [Google Scholar] [PubMed] [CrossRef]
74. Bonnelye E, Merdad L, Kung V, Aubin JE. The orphan nuclear estrogen receptor-related receptor α (ERRα) is expressed throughout osteoblast differentiation and regulates bone formation in vitro. JCB. 2001;28(5):971–84. doi:10.1083/jcb.153.5.971. [Google Scholar] [PubMed] [CrossRef]
75. Cai C, Yuan GJ, Huang Y, Yang N, Chen X, Wen L, et al. Estrogen-related receptor α is involved in the osteogenic differentiation of mesenchymal stem cells isolated from human periodontal ligaments. Int J Mol Med. 2013;31(5):1195–201. doi:10.3892/ijmm.2013.1305. [Google Scholar] [PubMed] [CrossRef]
76. Marie PJ, Cohen-Solal M. The expanding life and functions of osteogenic cells: from simple bone-making cells to multifunctional cells and beyond. J Bone Miner Res. 2018;33(2):199–210. doi:10.1002/jbmr.3356. [Google Scholar] [PubMed] [CrossRef]
77. Xu L, Qian Z, Wang S, Wang R, Pu XJ, Yang B, et al. Galectin-3 enhances osteogenic differentiation of precursor cells from patients with diffuse idiopathic skeletal hyperostosis via Wnt/β-catenin signaling. J Bone Miner Metab. 2022;37(4):724–39. doi:10.1002/jbmr.4508. [Google Scholar] [PubMed] [CrossRef]
78. Huang T, Liu RZ, Fu XK, Yao DS, Yang M, Liu QL, et al. Aging reduces an erralpha-directed mitochondrial glutaminase expression suppressing glutamine anaplerosis and osteogenic differentiation of mesenchymal stem cells. Stem Cells. 2017;35(2):411–24. doi:10.1002/stem.2470. [Google Scholar] [PubMed] [CrossRef]
79. Gallet M, Saïdi S, Haÿ E, Photsavang J, Marty C, Sailland J, et al. Repression of osteoblast maturation by ERRα accounts for bone loss induced by estrogen deficiency. PLoS One. 2013;8(1):e54837. doi:10.1371/journal.pone.0054837. [Google Scholar] [PubMed] [CrossRef]
80. Vancea A, Serban O, Fodor D. Relationship between osteopontin and bone mineral density. Acta Endocrinol. 2021;7(4):509–16. doi:10.4183/aeb.2021.509. [Google Scholar] [PubMed] [CrossRef]
81. Li KX, Sun X, Li BY, Yokota H. Conversion of osteoclasts into bone-protective, tumor-suppressing cells. Cancers. 2021;13(22):5593. doi:10.3390/cancers13225593. [Google Scholar] [PubMed] [CrossRef]
82. Zhu HF, Tamura A, Zhang SY, Terauchi M, Yoda T, Yui N. Mitigating RANKL-induced cholesterol overload in macrophages with β-cyclodextrin-threaded polyrotaxanes suppresses osteoclastogenesis. Biomater Sci UK. 2022;10(18):5230–42. doi:10.1039/D2BM00833. [Google Scholar] [CrossRef]
83. Wan Y. PPARγ in bone homeostasis. Trends Endocrinol Metab. 2012;23(5):722–8. doi:10.1016/j.tem.2010.08.006. [Google Scholar] [PubMed] [CrossRef]
84. Delhon I, Gutzwiller S, Morvan F, Rangwala S, Wyder L, Evans G, et al. Absence of estrogen receptor-related-α increases osteoblastic differentiation and cancellous bone mineral density. Endocrinol. 2009;150(10):4463–72. doi:10.1210/en.2009-0121. [Google Scholar] [PubMed] [CrossRef]
85. Zhang L, Wong J, Vanacker JM. The estrogen-related receptors (ERRspotential targets against bone loss. Cell Mol Life Sci. 2016;73(20):3781–7. doi:10.1007/s00018-016-2328-5. [Google Scholar] [PubMed] [CrossRef]
86. Yang D, Wan Y. Molecular determinants for the polarization of macrophage and osteoclast. Semin Immunopathol. 2019;41:551–63. doi:10.1007/s00281-019-00754-3. [Google Scholar] [PubMed] [CrossRef]
87. Wei W, Wang XQ, Yang M, Smith LC, Dechow PC, Sonoda JC, et al. PGC1β mediates PPARγ activation of osteoclastogenesis and rosiglitazone-induced bone loss. Cell Metab. 2010;11(6):503–16. doi:10.1016/j.cmet.2010.04.015. [Google Scholar] [PubMed] [CrossRef]
88. Wei W, Schwaid AG, Wang XQ, Wang XD, Chen SL, Chu Q, et al. Ligand activation of ERRα by cholesterol mediates statin and bisphosphonate effects. Cell Metab. 2016;23(3):479–91. doi:10.1016/j.cmet.2015.12.010. [Google Scholar] [PubMed] [CrossRef]
89. Che YT, Yang JZ, Tang F, Wei ZH, Chao YF, Li N, et al. New function of cholesterol oxidation products involved in osteoporosis pathogenesis. Int J Mol Sci. 2022;23(4):2020. doi:10.3390/ijms23042020. [Google Scholar] [PubMed] [CrossRef]
90. Casaburi I, Chimento A, Luca AD, Nocito M, Sculco S, Avena P, et al. Cholesterol as an endogenous ERRα agonist: a new perspective to cancer treatment. Front Endocrinol. 2018;9:525. doi:10.3389/fendo.2018.00525. [Google Scholar] [PubMed] [CrossRef]
91. Maurizi A, Rucci N. The osteoclast in bone metastasis: player and target. Cancers. 2018;10:218. doi:10.3390/cancers10070218. [Google Scholar] [PubMed] [CrossRef]
92. Zheng ZG, Cheng HM, Zhou YP, Zhu ST, Thu PM, Li HJ, et al. Dual targeting of SREBP2 and ERRα by carnosic acid suppresses RANKL-mediated osteoclastogenesis and prevents ovariectomy-induced bone loss. Cell Death Differ. 2020;27(7):2048–65. doi:10.1038/s41418-019-0484-5. [Google Scholar] [PubMed] [CrossRef]
93. Teixeira S, Branco L, Fernandes MH, Costa-Rodrigues J. Bisphosphonates and cancer: a relationship beyond the antiresorptive effects. Mini-Rev Med Chem. 2019;19(12):988–98. doi:10.2174/1389557519666190424163044. [Google Scholar] [PubMed] [CrossRef]
94. Okuyan HM, Begen MA. LncRNAs in osteoarthritis. Clin Chin Acta. 2022;532:145–63. doi:10.1016/j.cca.2022.05.030. [Google Scholar] [PubMed] [CrossRef]
95. Motta F, Barone E, Sica A, Selmi C. Inflammaging and osteoarthritis. Clin Rev Allerg Immu. 2023;64(2):222–38. doi:10.1007/s12016-022-08941-1. [Google Scholar] [PubMed] [CrossRef]
96. Yao Q, Wu XH, Tao C, Gong WY, Chen MJ, Qu MH, et al. Osteoarthritis: pathogenic signaling pathways and therapeutic targets. Signal Transduct Tar. 2023;8(1):56. doi:10.1038/s41392-023-01330-w. [Google Scholar] [PubMed] [CrossRef]
97. Huang T, Fu XK, Wang N, Yang M, Zhang MY, Wang BX, et al. Andrographolide prevents bone loss via targeting estrogen-related receptor-α-regulated metabolic adaption of osteoclastogenesis. Brit J Pharmacol. 2021;178(21):4352–67. doi:10.1111/bph.15614. [Google Scholar] [PubMed] [CrossRef]
98. Astrike-Davis EM, Coryell P, Loeser RF. Targeting cellular senescence as a novel treatment for osteoarthritis. Curr Opin Pharmacol. 2022;64:102213. doi:10.1016/j.coph.2022.102213. [Google Scholar] [PubMed] [CrossRef]
99. Wang YY, Xu JJ, Zhang XD, Wang CD, Huang Y, Dai K, et al. TNF-α-induced LRG1 promotes angiogenesis and mesenchymal stem cell migration in the subchondral bone during osteoarthritis. Cell Death Dis. 2017;8:e2715. doi:10.1038/cddis.2017.129. [Google Scholar] [PubMed] [CrossRef]
100. Kang YH, Lee HJ, Lee CJ, Park JS. Natural products as sources of novel drug candidates for the pharmacological management of osteoarthritis: a narrative review. Biomol Ther. 2019;27(6):503–13. doi:10.4062/biomolther.2019.139. [Google Scholar] [PubMed] [CrossRef]
101. Hawker GA. Osteoarthritis is a serious disease. Clin Exp Rheumatol. 2019;120(5):3–6. [Google Scholar]
102. Son YO, Park S, Kwak JS, Won Y, Choi WS, Rhee J, et al. Estrogen-related receptor γ causes osteoarthritis by upregulating extracellular matrix-degrading enzymes. Nat Commun. 2017;8:2133. doi:10.1038/s41467-017-01868-8. [Google Scholar] [PubMed] [CrossRef]
103. Yu L, Zhang XG, Liu XC, Li G, Chen ML, Liu ZX, et al. CircTMOD3 promotes lipopolysaccharide-induced chondrocyte apoptosis in osteoarthritis by sponging miR-27a. J Bone Miner Metab. 2022;40(3):415–21. doi:10.1007/s00774-022-01310-0. [Google Scholar] [PubMed] [CrossRef]
104. Mehana EE, Khafaga AF, El-Blehi SS. The role of matrix metalloproteinases in osteoarthritis pathogenesis: an updated review. Life Sci. 2019;234:116786. doi:10.1016/j.lfs.2019.116786. [Google Scholar] [PubMed] [CrossRef]
105. Huang M, Chen LL, Mao XD, Liu GF, Gao YQ, You XQ, et al. ERRα inhibitor acts as a potential agonist of PPARγ to induce cell apoptosis and inhibit cell proliferation in endometrial cancer. Aging. 2020;12(24):23029–46. doi:10.18632/aging.104049. [Google Scholar] [PubMed] [CrossRef]
106. Li MY, Yu YJ, Xue K, Li JY, Son GH, Wang JJ, et al. Genistein mitigates senescence of bone marrow mesenchymal stem cells via ERRα-mediated mitochondrial biogenesis and mitophagy in ovariectomized rats. Redox Biol. 2023;61:102649. doi:10.1016/j.redox.2023.102649. [Google Scholar] [PubMed] [CrossRef]
107. Kovács B, Vajda E, Nagy EE. Regulatory effects and interactions of the Wnt and OPG-RANKL-RANK signaling at the bone-cartilage interface in osteoarthritis. Int J Mol Sci. 2019;20(18):4653. doi:10.3390/ijms20184653. [Google Scholar] [PubMed] [CrossRef]
108. Liu CF, Angelozzi M, Haseeb A, Lefebvre V. SOX9 is dispensable for the initiation of epigenetic remodeling and the activation of marker genes at the onset of chondrogenesis. Dev. 2018;145(14):dev164459. doi:10.1242/dev.164459. [Google Scholar] [PubMed] [CrossRef]
109. Wu SC, Chen CH, Wang JY, Lin YS, Chang JK, Ho ML. Hyaluronan size alters chondrogenesis of adipose-derived stem cells via the CD44/ERK/SOX-9 pathway. Acta Biomater. 2018;66:224–37. doi:10.1016/j.actbio.2017.11.025. [Google Scholar] [PubMed] [CrossRef]
110. Auld KL, Berasi SP, Liu Y, Cain M, Zhang Y, Huard C, et al. Estrogen-related receptor α regulates osteoblast differentiation via Wnt/β-catenin signaling. J Mol Endocrinol. 2012;48(2):177–91. doi:10.1530/JME-11-0140. [Google Scholar] [PubMed] [CrossRef]
111. Bonnelye E, Zirngibl RA, Jurdic P. The orphan nuclear estrogen receptor-related receptor-α regulates cartilage formation in vitro: implication of Sox9. Endocrinology. 2007;148(3):1195–205. doi:10.1210/en.2006-0962. [Google Scholar] [PubMed] [CrossRef]
112. Chen X, Cai C, Liu J, Wen L, Wang X, Ding Y. Impact of estrogen-related receptor α on the biological characteristics of rat mandibular condylar chondrocytes. Mol Med Rep. 2014;10(1):195–202. doi:10.3892/mmr.2014.2210. [Google Scholar] [PubMed] [CrossRef]
113. Bonnelye ERP, Duval N, Cardelli M, Aubin JE. Estrogen receptor-related receptor-α regulation by interleukin-1β in prostaglandin E2- and cAMP-dependent pathways in osteoarthritic chondrocytes. Arthritis Rheum. 2011;63(8):2374–84. doi:10.1002/art.30398. [Google Scholar] [PubMed] [CrossRef]
114. Liao CR, Wang SN, Zhu SY, Wang YQ, Li ZZ, Liu ZY, et al. Advanced oxidation protein products increase TNF-α and IL-1β expression in chondrocytes via NADPH oxidase 4 and accelerate cartilage degeneration in osteoarthritis progression. Redox Biol. 2020;28:10130. doi:10.1016/j.redox.2019.101306. [Google Scholar] [PubMed] [CrossRef]
115. Shen LJ, Ji C, Lin J, Yang HP. Regulation of circADAMTS6-miR-324-5p-PIK3R3 ceRNA pathway may be a novel mechanism of IL-1β-induced osteoarthritic chondrocytes. J Bone Miner Metab. 2022;40(3):389–401. doi:10.1007/s00774-021-01308-0. [Google Scholar] [PubMed] [CrossRef]
116. Tang JS, Liu T, Wen XJ, Zhou ZS, Yan JT, Gao JP, et al. Estrogen-related receptors: novel potential regulators of osteoarthritis pathogenesis. Mol Med. 2021;27(1):5. doi:10.1186/s10020-021-00270-x. [Google Scholar] [PubMed] [CrossRef]
117. Yudoh K, Karasawa R. Statin prevents chondrocyte aging and degeneration of articularcartilage in osteoarthritis (OA). Aging. 2010;2(12):990–8. doi:10.18632/aging.100213. [Google Scholar] [PubMed] [CrossRef]
118. Brindisi M, Frattaruolo L, Fiorillo M, Dolce V, Sotgia F, Lisanti MP, et al. New insights into cholesterol-mediated ERRα activation in breast cancer progression and pro-tumoral microenvironment orchestration. FEBS J. 2023;290(6):1481–501. doi:10.1111/febs.16651. [Google Scholar] [PubMed] [CrossRef]
119. Ghanbari F, Mader S, Philip A. Cholesterol as an endogenous ligand of ERRα promotes ERRα-mediated cellular proliferation and metabolic target gene expression in breast cancer cells. Cells. 2020;9(8):1765. doi:10.3390/cells9081765. [Google Scholar] [PubMed] [CrossRef]
120. Shi MY, Bang IH, Han CY, Lee DH, Park BH, Bae EJ. Statin suppresses sirtuin 6 through miR-495, increasing FoxO1-dependent hepatic gluconeogenesis. Theranostics. 2020;10(25):11416–27. doi:10.7150/thno.49770. [Google Scholar] [PubMed] [CrossRef]
121. Stee MFV, Graaf AAD, Groen AK. Actions of metformin and statins on lipid and glucose metabolism and possible benefit of combination therapy. Cardiovasc Diabetol. 2018;17(1):94. doi:10.1186/s12933-018-0738-4. [Google Scholar] [PubMed] [CrossRef]
122. Yandrapalli S, Malik A, Guber K. Statins and the potential for higher diabetes mellitus risk. Expert Rev Clin Phar. 2019;12(9):825–30. doi:10.1080/17512433.2019.1659133. [Google Scholar] [PubMed] [CrossRef]
123. He S, Li X, Ma JZ, Yang Y, Luo S, Xie XD, et al. The potential endocrine disruption mechanism of anthelmintic drug niclosamide by activating estrogen receptors and estrogen-related receptors. Toxicol. 2021;457:152805. doi:10.1016/j.tox.2021.152805. [Google Scholar] [PubMed] [CrossRef]
124. Teng CT, Hsieh JH, Zhao JH, Huang RL, Xia MH, Martin NG, et al. Development of novel cell lines for high-throughput screening to detect estrogen-related receptor alpha modulators. Slas Discov. 2017;22(6):720–31. doi:10.1177/2472555216689772. [Google Scholar] [PubMed] [CrossRef]
125. Scheepstra M, Hekking KFW, van Hijfte L, Folmer RHA. Bivalent ligands for protein degradation in drug discovery. Comput Struct Biotechnol J. 2019;17:160–76. doi:10.1016/j.csbj.2019.01.006. [Google Scholar] [PubMed] [CrossRef]
126. Lu YJ, Lu X, Wang LF, Yang W. Resveratrol attenuates high fat diet-induced mouse cardiomyopathy through upregulation of estrogen related receptor-α. Eur J Pharmacol. 2019;843:88–95. doi:10.1016/j.ejphar.2018.10.018. [Google Scholar] [PubMed] [CrossRef]
127. Ning Y, Chen HF, Du YL, Ling H, Zhang LD, Chen L, et al. A novel compound LingH2-10 inhibits the growth of triple negative breast cancer cells in vitro and in vivo as a selective inverse agonist of estrogen-related receptor α. Biomed Pharmacother. 2017;93:913–22. doi:10.1016/j.biopha.2017.07.016. [Google Scholar] [PubMed] [CrossRef]
128. Gao ZP, Wang TX, Li R, Du YL, Lv H, Zhang LD, et al. The discovery of a novel series of potential ERRα inverse agonists based on p-nitrobenzenesulfonamide template for triple-negative breast cancer in vivo. J Enzym Inhib Med Ch. 2023;37(1):125–34. doi:10.1080/14756366.2021.199572. [Google Scholar] [CrossRef]
129. Kokabu T, Mori T, Matsushima HS, Yoriki K, Kataoka HS, Tarumi Y, et al. Antitumor efect of XCT790, an ERRα inverse agonist, on ERα-negative endometrial cancer cells. Cell Oncol. 2019;42(2):223–35 (In Chinese). doi:10.1007/s13402-019-00423-5. [Google Scholar] [PubMed] [CrossRef]
130. Zhang RX, Gao YQ, Lei L, Wu DP, Zhu TT, Chu JH. Intervention of ERRα expression on apoptosis induction of multiple myeloma MM. 1S cells cultured in vitro. Zhongguo Shi Yan Xue Ye Xue Za Zhi. 2022;30(2):476–80 (In Chinese). doi:10.19746/j.cnki.issn.1009-2137.2022.02.025. [Google Scholar] [PubMed] [CrossRef]
131. Liu SL, Liang HB, Yang ZY, Cai C, Wu ZY, Wu XS, et al. Gemcitabine and XCT790, an ERRα inverse agonist, display a synergistic anticancer effect in pancreatic cancer. Int J Med Sci. 2022;19(2):286–98. doi:10.7150/ijms.68404. [Google Scholar] [PubMed] [CrossRef]
132. Chen CY, Li Y, Zeng N, He L, Zhang XW, Tu T, et al. Inhibition of estrogen-related receptor α blocks liver steatosis and steatohepatitis and attenuates triglyceride biosynthesis. Am J Speech-lang Pat. 2021;191(7):1240–54. doi:10.1016/j.ajpath.2021.04.007. [Google Scholar] [PubMed] [CrossRef]
133. Lynch C, Zhao JH, Sakamuru S, Zhang L, Huang R, Witt KL, et al. Identification of compounds that inhibit estrogen-related receptor alpha signaling using high-throughput screening assays. Mol. 2019;24(5):841. doi:10.3390/molecules24050841. [Google Scholar] [PubMed] [CrossRef]
134. Heinz S, Freyberger A, Lawrenz B. Investigations of the mitochondrial complex I inhibitor rotenone in the context of pharmacological and safety evaluation. Sci Rep. 2017;7:45465. doi:10.1038/srep45465. [Google Scholar] [PubMed] [CrossRef]
135. Xia MH, Huang RL, Shi Q, Boyd WA, Zhao JH, Sun N, et al. Comprehensive analyses and prioritization of Tox21 10K chemicals affecting mitochondrial function by in-depth mechanistic studies. Environ Health Persp. 2018;126(7):077010. doi:10.1289/EHP2589. [Google Scholar] [PubMed] [CrossRef]
136. Jang Y, Kim J, Jeong S, Paik M, Kim JS, Cho MH. Trifloxystrobin-induced mitophagy through mitochondrial damage in human skin keratinocytes. J Toxicol Sci. 2016;41(6):731–7. doi:10.2131/jts.41.731. [Google Scholar] [PubMed] [CrossRef]
137. Teng CT, Beames B, Alex Merrick B, Martin N, Romeo C, Jetten AM. Development of a stable cell line with an intact PGC-1α/ERRα axis for screening environmental chemicals. Biochem Biophys Res Commun. 2014;444(2):177–81. doi:10.1016/j.bbrc.2014.01.033. [Google Scholar] [PubMed] [CrossRef]
138. Lu RR, Zheng ZC, Yin YM, Jiang ZQ. Effect of genistein on cholesterol metabolism-related genes in HepG2 cell. J Food Sci. 2019;84(8):2330–6. doi:10.1111/1750-3841.14725. [Google Scholar] [PubMed] [CrossRef]
139. Shinozuka T, Ito S, Kimura T, Izumi M, Wakabayashi K. Discovery of a novel class of ERRα agonists. ACS Med Chem Lett. 2021;12(5):817–8. doi:10.1021/acsmedchemlett.1c00100. [Google Scholar] [PubMed] [CrossRef]
140. Schoepke E, Billon C, Haynes KM, Avdagic A, Sitaula S, Sanders R, et al. A selective ERRα/γ Inverse agonist, SLU-PP-1072, inhibits the warburg effect and induces apoptosis in prostate cancer cells. ACS Chem Biol. 2020;15(9):2338–45. doi:10.1021/acschembio.0c00670. [Google Scholar] [PubMed] [CrossRef]
141. Patch RJ, Searle LL, Kim AJ, De D, Zhu XZ, Askari HB, et al. Identifcation of diaryl ether-based ligands for estrogenrelated receptor α as potential antidiabetic agents. J Med Chem. 2011;54(3):788–808. doi:10.1021/jm101063h. [Google Scholar] [PubMed] [CrossRef]
142. Patch RJ, Huang H, Patel S, Cheung W, Xu GZ, Zhao BP, et al. Indazole-based ligands for estrogen-related receptor α as potential anti-diabetic agents. Eur J Med Chem. 2017;138:830–53. doi:10.1016/j.ejmech.2017.07.015. [Google Scholar] [PubMed] [CrossRef]
143. Krzysik-Walker SM, González-Mariscal I, Scheibye-Knudsen M, Indig FE, Bernier M. The biarylpyrazole compound AM251 alters mitochondrial physiology via proteolytic degradation of ERRα. Mol Pharmacol. 2013;83(1):157–66. doi:10.1124/mol.112.082651. [Google Scholar] [PubMed] [CrossRef]
144. Lu X, Peng L, Lv M, Ding K. Recent advance in the design of small molecular modulators of estrogen-related receptors. Curr Pharm Des. 2012;18(23):3421–31. [Google Scholar] [PubMed]
145. Li DP, Jiang KX, Teng D, Wu ZR, Li WH, Tang Y, et al. Discovery of new estrogen-related receptor α agonists via a combination strategy based on shape screening and ensemble docking. J Chem Inf Model. 2022;62(3):486–97. doi:10.1021/acs.jcim.1c00662. [Google Scholar] [PubMed] [CrossRef]
146. Choi WS, Lee G, Song WH, Koh JT, Yang JY, Kwak JS, et al. The CH25H-CYP7B1-RORα axis of cholesterol metabolism regulates osteoarthritis. Nature. 2019;566:254–8. doi:10.1038/s41586-019-0920-1. [Google Scholar] [PubMed] [CrossRef]
147. Tripathi M, Yen PM, Singh BK. Estrogen-related receptor alpha: an underappreciated potential target for the treatment of metabolic diseases. Int J Mol Sci. 2020;21:1645. doi:10.3390/ijms21051645. [Google Scholar] [PubMed] [CrossRef]
148. Kumari K, Keshari S, Sengupta D, Sabat SC, Mishra SK. Transcriptome analysis of genes associated with breast cancer cell motility in response to Artemisinin treatment. BMC Cancer. 2017;17:858. doi:10.1186/s12885-017-3863-7. [Google Scholar] [PubMed] [CrossRef]
149. Pan ZZ, Wang K, Wang X, Jia ZR, Yang YQ, Duan YL, et al. Cholesterol promotes EGFR-TKIs resistance in NSCLC by inducing EGFR/Src/Erk/SP1 signaling-mediated ERRα re-expression. Mol Cancer. 2022;21:77. doi:10.1186/s12943-022-01547-3. [Google Scholar] [PubMed] [CrossRef]
150. Li DP, Cai YC, Teng D, Li WH, Tang Y, Liu GX. Computational insights into the interaction mechanisms of estrogen-related receptor alpha with endogenous ligand cholesterol. Chem Biol Drug Des. 2019;94:1316–29. doi:10.1111/cbdd.13506. [Google Scholar] [PubMed] [CrossRef]
151. Suetsugi M, Karlsberg LSK. Flavone and isofavone phytoestrogens are agonists of estrogen-related receptors. Mol Cancer Res. 2003;1:981–91. [Google Scholar] [PubMed]
Cite This Article
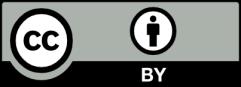
This work is licensed under a Creative Commons Attribution 4.0 International License , which permits unrestricted use, distribution, and reproduction in any medium, provided the original work is properly cited.