Open Access
ARTICLE
The regulatory role of melatonin in pituitary thyroid-stimulating hormone synthesis through casein kinase 1α
1 College of Veterinary Medicine, Yangzhou University, Yangzhou, 225009, China
2 Institute of Reproduction and Metabolism, Yangzhou University, Yangzhou, 225009, China
3 Jiangsu Co-Innovation Center for Prevention and Control of Important Animal Infectious Diseases and Zoonoses, Yangzhou University, Yangzhou, 225009, China
* Corresponding Author: SHENG CUI. Email:
(This article belongs to the Special Issue: Subcellular Organelles and Cellular Molecules: Localization, Detection, Prediction, and Diseases)
BIOCELL 2024, 48(2), 327-338. https://doi.org/10.32604/biocell.2023.044630
Received 04 August 2023; Accepted 26 October 2023; Issue published 23 February 2024
Abstract
Introduction: The regulation of thyroid-stimulating hormone (TSH) synthesis involves neurotransmitters, with melatonin being a subject of ongoing debate. TSH transcription, synthesis, and secretion from the pituitary pars distalis (PD) is primarily regulated in a photoperiodic manner by thyrotropin-releasing hormone (TRH). In contrast, in the pituitary pars tuberalis (PT), mRNA transcription and alpha/beta chain synthesis, but not secretion, of a TSH-like product is regulated by melatonin. Conversely, non-photoperiodic melatonin might also affect the secretion of a TSH-like product from the PT. Nevertheless, the impact of exogenous melatonin on the underlying PD-TSH synthesis remains unclear. Casein kinase 1α (CK1α) plays a negative regulatory role in TSH synthesis in the mouse pituitary. Objective: We investigated whether non-photoperiodic melatonin affects PD-TSH synthesis through its interaction with CK1α. Methods: Immunohistochemistry and immunofluorescence staining detected the colocalization of the melatonin receptor MT1 with CK1α and TSH-β in the PD. RT-qPCR, western blotting, and ELISA revealed the effect of melatonin on Tshb mRNA, MTNR1A mRNA, Csnk1a1 mRNA, CK1α protein, MT1 protein, and TSH levels. Results: Robust colocalization of the melatonin receptor MT1 with CK1α and TSH-β in the PD. Tshb mRNA and CK1α protein expression levels peaked at opposite phases of the 24-h light:dark cycle. Exogenous melatonin administration promoted pituitary TSH synthesis, while concurrently inhibiting CK1α activity. The upregulation of endogenous CK1α activity in primary pituitary cells significantly blunted the melatonin stimulatory impact on Tshb mRNA and TSH levels. Mechanistically, the CK1α agonist pyrvinium abrogated melatonin-induced activation of p-PKC and p-CREB expression in vitro. Conclusion: The CK1α/PKC signaling pathway mediates the regulation of melatonin in pituitary TSH synthesis. We demonstrate an important theoretical and experimental basis for understanding the mechanism of endocrine system diseases caused by abnormal TSH synthesis in the pituitary.Keywords
A study on tortoises identified two thyrotrope populations with distinct properties and morphology in the pars tuberalis (PT) and pars distalis (PD) of the pituitary [1]. The synthesis of PT- thyroid-stimulating hormone (TSH) has been found to mainly be regulated by melatonin (MLT) [2,3]. Meanwhile, a short photoperiod (i.e., long melatonin signal) was correlated with a decrease in PT Tshb mRNA [4] and protein levels [5]. The synthesis of PD-TSH is mainly regulated by hypothalamic input and thyroid feedback, and also by rhythm factors in the adenohypophysis [6]; however, the mechanisms underlying the regulatory effects of non-photoperiodic MLT on PD-TSH synthesis and secretion remains unclear.
Melatonin, N-acetyl-5-hydroxytryptamine, is a hormone that synchronizes the internal environment with the photoperiod. The regulation of MLT in the physiological process includes antioxidation, immunity and apoptosis [7]. MLT can regulate target cells in two ways. One is through a receptor-independent pathway, where MLT can be transported to the target cell only through blood without carrier proteins, because of its water solubility, allowing easily crossing of the cell membrane into the cytoplasm to perform its physiological function [8]. The other way is through a receptor-dependent pathway; most of the research on the mechanism of MLT depends on this pathway. There are mainly two kinds of G protein-coupled receptors that bind MLT in mammals, namely Mel1a (MT1) and Mel1b (MT2) [9]. Immunoreactivity to MT1 accues in the human hypothalamus and pituitary gland [10]. Stimulation of Gi-, Gs-, and Gq-protein-coupled receptors can modulate the MAPK cascade of mitogen-activated protein kinase (MAPK) [11]. It has also been reported that upon exposure to MLT in HEK293 cells, the phosphorylation of ERK1/2 mediated via PI3K/PDK1/PKC pathway which was activated by combination of MT1 receptors and Gi proteins and the dissociation of βγ subunits from Gαi [12].
Casein kinase 1α (CK1α) is important for the circadian system of multiple taxa, including mammals, flies, and fungi [13]. CK1α collaborates with double-time (Dbt) to regulate period (PER) function in the circadian rhythm of drosophila [14]. Other enzymes also influence the circadian system, including calcium/calmodulin-dependent kinase 1, protein phosphatases 1 and 2A, checkpoint kinase 2, and protein kinase C (PKC) [15]. CK1α is a component of the β-catenin degradation complex and plays a negative regulatory role in Wnt/β-catenin signaling pathway [16]. Photoperiod-induced changes in the expressions levels of some Wnt-related genes have been reported in photoperiod-sensitive F344 rats [17]. Studies have found that MLT can participate in the regulation of the classical Wnt/β-catenin pathway. For example, MLT can inhibit the phosphorylation level of Akt and activate GSK3β, and the activated GSK3β can induce the degradation of β-catenin [18].
Previously, we demonstrated that CK1α regulates PD-TSH synthesis by modulating the PKC/ERK/CREB signaling pathway in pituitary tissues [19]. In this study, we sought to clarify whether non-photoperiodic melatonin can regulate TSH output from the pars distalis and whether CK1α mediates the effects of melatonin. Our findings should extend current understanding of melatonin action and central thyrotropin synthesis regulation, and provides a theoretical and experimental basis for the pathogenesis of endocrine system diseases caused by abnormal pituitary TSH hormone synthesis.
Male ICR mice (age: 6–8 weeks, weight: 25–33 g) were bought from Yangzhou University Medical Center (Jiangsu, China) and subjected to a standard LD cycle (12L:12D) with lights on at 07:00 h and off at 19:00 h. Mice were offered a commercial mouse diet (Medicine, Jiangsu, China) and water ad libitum throughout the experiment. The protocol (from design to implementation) was in line with the methods put forward by the National Research Council’s Guide for the Care and Use of Laboratory Animals. Approval of the current work was provided by the Animal Protection and Utilization Committee of Yangzhou University (SYXK[Su] 2017-0044).
For in vitro experiments, 0.1% collagenase II (Sigma, St. Louis, MO, USA) and 0.25% pancreatic enzyme (Sigma, St. Louis, MO, USA) were used for digestion of freshly dissected mouse pituitary PD [20]. The cells of anterior pituitary were harvested and resuspended with Dulbecco’s modified Eagle medium (DMEM/F-12, GIBCO-Invitrogen, Grand Island, NY, USA), and 10% fetal bovine serum and antibiotics (penicillin G 100 IU/mL, streptomycin 100 µg/mL) were added, followed by cultured in 24-well plates at a density of 105 cells/well. The cells were incubated at 37°C for 12 h in a mixture of 95% air and 5% CO2. Each experiment involved at least three to four independent replicates, and each trial contained three to five replicates per treatment condition.
The CK1α agonist pyrvinium (MCE, Shanghai, China) was used to determine whether CK1α mediates the effects of melatonin on TSH synthesis in PD cells.
To explore the role of melatonin in CK1α expression and TSH synthesis, five mice were subjected to subcutaneous injection in the neck with 100 µL of 0.9% NaCl and 6 µg melatonin (Macklin, Shanghai, China) daily 1 h before lights off for 3 weeks. A control group of five mice was simultaneously injected with NaCl only [4].
TSH enzyme-linked immunosorbent assay (TSH-ELISA)
Following in vivo and in vitro experiments, blood and cell culture supernatants were collected. The samples were centrifuged at 4,500 rpm, for 5 min at 4°C, then divided into equal aliquots, and stored at −20°C until use. TSH levels (pg/mL) in the stored samples were determined using an ELISA kit according to the manufacturer’s instructions (Adanti, Wuhan, China). The absorbance of each well was measured at 450 nm using a microplate reader. The detection range of the ELISA kit was 2–90 pg/mL (the final dilution of the sample was five times). The intra- and inter-determination coefficients of variation of the parameters were less than 9% and 11%, respectively.
Quantitation of exogenous melatonin
Reagents (methanol, 0.1% (v/v) formic acid, ultrapure water, and ethyl acetate) were of chromatographic grade. After 3 weeks of melatonin administration, blood samples were collected for quantification. Fresh serum samples (300 µL) were treated with 1 mL of ethyl acetate, shaken for 3 min, then centrifuged at 13,400 × g and 4°C for 10 min. The supernatant was collected, dried under nitrogen for 15 min, then resuspended in 0.15 mL of a solution containing 65 parts of 0.1% (v/v) formic acid in ultrapure water and 35 parts of methanol. The mixture was swirled for 1 min and centrifuged for 10 min at 12,000 rpm and 4°C. 1 mL syringe was used to collect the supernatant. The supernatant was collected using a 1 mL syringe. Samples were separated and detected with high-performance liquid chromatography tandem mass spectrometry.
The mobile phase consisted of 0.1% (v/v) formic acid in ultrapure water (buffer A) and methanol (buffer B). Mass spectrometry procedures followed an established method, with peak injection and extraction times at approximately 3.75 min [21]. The melatonin standard was diluted two-fold, dissolved in methanol, and diluted in the mobile phase (A:B = 65:35) at 10, 5, 2.5, 1.25, 0.625, 0.3125, 0.156, 0.078, 0.04, 0.02, and 0.01 ng/mL.
Real-time quantitative PCR (RT-qPCR)
Total RNA was extracted from the pituitary PD or cultured pituitary PD cells using the TRIzol reagent (Takara, Dalian, China) according to the manufacturer’s instructions. Complementary DNAs were synthesized using M-MLV reverse transcription reagents (Vazyme, Nanjing, China). Gene expression levels were measured using SYBR Green master mix (Vazyme, Nanjing, China) in the ABI-PRISM 7500 Sequence Detection System (Applied Biosystems, Foster City, CA, USA). Endogenous Gapdh was used for standardization. Finally, according to the Ct value, the relative expression was calculated by the 2−ΔΔCt method. Suppl. Table S1 shows all primer sequences.
Immunohistochemistry and immunofluorescence
To conduct immunofluorescence and immunohistochemistry, the pituitary PDs were fixed in 4% paraformaldehyde, embedded in paraffin, and cut into 5 µm pieces. The whole pituitary was cut into 15 pieces, and every three slices were stained. Next, sections were then dewaxed using xylene for 10 min before being incubated with 100%, 95%, 85%, 75%, and 50% ethanol for 5 min each at 25°C. Antigen retrieval was performed in 0.01 mol/L Sodium Citrate Buffer (pH6.0). Then, the sections were treated with 10% normal donkey or goat serum in a phosphate buffer solution (PBS) for 1 h at 25°C to block non-specific binding sites, incubated at 4°C overnight with primary antibody and washed with PBS (for antibodies, see Suppl. Table S2). Sections were then incubated with fluorescent conjugated secondary antibodies for 1 h at 25°C, then incubated with 4’, 6’-diamino-2-phenylindole (DAPI, Beyotime, Shanghai, China) for 10 min, and incubated again at 25°C. Finally, sections were visualized under an Olympus IX71 fluorescence microscope (Olympus, Tokyo, Japan). The number of TSH-β+, CK1α+, MT1+, TSH-β+-MT1+ and CK1α+-MT1+ cells was counted in five slices of each pituitary.
Proteins were extracted from the pituitary of the mice or the cultured primary pituitary cells by the radioimmunoprecipitation (Cell Signaling, Danvers, MA, USA) containing 1 mmol/L phenylmethylsulfonyl fluoride (PMSF; Cell Signaling, Danvers, MA, USA). Protein concentration was determined in accordance with the manufacturer’s instructions following a bicinchoninic acid assay (Vigorous Biotechnology, Beijing, China). The protein lysates were electrophoresed on 13% SDS-PAGE and then transferred to the polyvinylidene fluoride membranes (Bio-Rad Laboratories, Richmond, CA, USA), which were blocked for 1 h with 5% (w/v) non-fat dry milk, then incubated with antibodies for 12 h at 4°C. Then the membranes were washed thrice in Tris-buffered saline with Tween-20 and incubated with horseradish peroxidase (HRP)-conjugated donkey anti-goat IgG, HRP-conjugated goat anti-mouse IgG, or HRP-conjugated goat anti-rabbit IgG for 1–2 h at 25°C. After washing, the protein bands were visualized using a highly sensitive enhanced chemiluminescence detection kit (E412-01; Vazyme, Nanjing) at 2°C–8°C. Gray values were analyzed in ImageJ 1.53a. All antibodies are shown in Suppl. Table S2.
Data are presented as means ± S.E.M. of at least three independent experiments using GraphPad Prism 9.0 software. Student’s t-test was used for single comparison, and one-way analysis of variance was used for multiple comparisons; p < 0.05 was considered statistically significant, and p < 0.01 was considered extremely significant.
Expression and distribution of MT1/MT2 in mouse pituitary tissue
The results of RT-qPCR indicated that MTNR1A mRNA expression (encoding the MT1 receptor) was higher than MTNR1B mRNA expression (encoding the MT2 receptor) in the pituitary gland (Fig. 1A). Their respective proteins exhibited similar differences according to WB results (Fig. 1B). Immunohistochemistry then detected MT1 receptor signals in the mouse PD, whereas MT2 receptors signals were found in the pars intermedia (PI) (Fig. 1C). These findings suggest that melatonin is important in mouse PD hormone synthesis.
Figure 1: Expression and distribution of MT1/MT2 melatonin receptors in mouse pituitary tissue. (A) MTNR1A and MTNR1B mRNA expression in mouse tissues and the difference in pituitaries were analyzed by RT-qPCR; Gapdh was used as the internal parameters, *p < 0.05; n = 3. (B) MT1 and MT2 protein levels in mice tissues and the difference in pituitary were analyzed by western blot (WB), α-tubulin was used as internal parameters, *p < 0.05; n = 3. (C) Immunohistochemical detection of MT1 and MT2 receptor expression in pituitary tissue. NC: negative control; MT1: Mel1a; MT2: Mel1b; PD: pars distalis; PI: pars intermedia. Scale bar = 50, 100 μm.
Melatonin promotes PD-TSH synthesis in mice
Melatonin is the main circadian-rhythm-regulating hormone in animals. We investigated the effects of melatonin administration on melatonin levels and PD-TSH expression in vivo using liquid chromatography, RT-qPCR, and WB. Exogenous melatonin increased melatonin by 10-fold from control levels (Fig. 2A). Furthermore, Tshb mRNA expression and TSH level increased two-fold and 1.6-fold from control levels in melatonin-treated mice respectively, whereas Mtnr1A mRNA expression did not change significantly (Figs. 2B–2D). Melatonin treatment increased MT1 protein expression by 1.3-fold from control levels (Fig. 2E).
Figure 2: Melatonin promotes PD-TSH synthesis in mice. (A) Serum melatonin content in mice measured using liquid chromatography after melatonin injection. **p < 0.01; n = 5. (B) After melatonin administration for 30 min, RT-qPCR was used to measure the mRNA of key pituitary hormones: Gh, Pomc, Prl, Fsh, Lh, and Tsh; n = 4. *p < 0.05, **p < 0.01, and ns = p > 0.05. (C) TSH levels in blood serum measured using ELISA kit after melatonin injection; n = 4. *p < 0.05. (D) Effect of melatonin treatment on Mtnr1A mRNA in mouse PD, determined using RT-qPCR. ns = p > 0.05; n = 5. (E) MT1 levels of WB in mouse PD. *p < 0.05; n = 5. (F, G) Tshb mRNA detected with RT-qPCR in primary pituitary cells treated with different melatonin concentrations (0, 0.1, 1 and 10 μM) for 5 min and 10 μM melatonin for different timepoints (0, 5, 10, and 20 min). Gapdh was used as an internal reference. *p < 0.05, ns = p > 0.05; n = 3. (H) TSH levels in cell culture supernatant measured using ELISA after 10 μM melatonin for different timepoints (0, 5, 10, and 20 min); *p < 0.05, ns = p > 0.05; n = 3.
These in vitro results are similar to the results described in in vivo. We treated primary pituitary cells with melatonin at different concentrations (0, 0.1, 1 and 10 μM) and at different timepoints (0, 5, 10 and 20 min). Treatment with 10 μM melatonin for 5 min increased Tshb mRNA and TSH level (Figs. 2F–2H).
Melatonin downregulates CK1α to promote PD-TSH synthesis
Notably, immunofluorescence staining showed that 45% MT1 and TSH-β signals colocalized. Additionally, 90% of the MT1 and CK1α signals colocalized in the PD (Figs. 3A and 3B). Next, we used RT-qPCR and WB to measure the effects of melatonin administration on CK1α expression in vivo. Although melatonin did not significantly affect Csnk1a1 mRNA expression (Fig. 3C), it decreased the ratio of phosphorylated to total CK1α protein (p-CK1α/CK1α) by 1.6-fold from control levels (Fig. 3D).
Figure 3: Melatonin promotes PD-TSH synthesis by downregulating CK1α activity. (A, B) Immunofluorescent staining for MT1 (green), CK1α (red) and TSH-β (red) in the adult mouse PD. Nuclei are stained blue using DAPI. Yellow staining in the merged channel indicates colocalization. Scale bar: 10 µm. (C) Effect of melatonin treatment on Csnk1a1 mRNA levels in mouse PD according to RT-qPCR. ns = p > 0.05; n = 5. (D) Effect of melatonin on phospho-CK1α and total CK1α levels in the pituitary according to WB. *p < 0.05; n = 5. (E, F) Csnk1a1 mRNA levels in primary pituitary cells treated with different melatonin concentrations (0, 0.1, 1 and 10 μM) for 5 min and 10 μM melatonin for different timepoints (0, 5, 10, and 20 min) were detected by RT-qPCR. Gapdh was the internal reference. *p < 0.05, ns = p > 0.05; n = 3. CK1α protein levels were detected by WB; α-tubulin was the internal loading control. **p < 0.01, ns = p > 0.05; n = 3. (G) Tshb mRNA expression measured with RT-qPCR after primary cultured murine pituitary cells were treated with pyrvinium for 6 h, followed by 10 µM melatonin for 5 min. *p < 0.05, ns = p > 0.05; n = 3. (H) TSH levels in cell culture supernatant measured with ELISA kit after primary cultured murine pituitary cells were treated with pyrvinium for 6 h, followed by 10 µM melatonin for 5 min. *p < 0.05, ns = p > 0.05; n = 3.
These in vitro results are similar to the results described in vivo. We treated primary pituitary cells with melatonin at different concentrations (0, 0.1, 1, and 10 μM) and timepoints (0, 5, 10, and 20 min). In response to treatment with 10 μM melatonin for 5 min, Csnk1a1 mRNA and protein levels significantly decreased (Figs. 3E and 3F). We then determined whether melatonin regulated PD-TSH by acting on CK1α. The primary pituitary cells were pre-treated with CK1α activator pyrvinium for 6 h, followed by 5 min of melatonin treatment. These results suggest that pyrvinium blunted the upregulation of PD-TSH synthesis induced by melatonin (Figs. 3G and 3H).
CK1α/PKC/ERK/CREB and CK1α/β-catenin signaling pathways are associated with mouse PD-TSH rhythms
We previously found that CK1α is highly expressed in pituitary TSH cells [19], and TSH is known to be rhythmic [22]. To explore possible variation in the relationship between CK1α and TSH, we first measured Tshb mRNA and CK1α protein expression under 12:12 light-dark conditions (LD; zeitgeber time ZT7-lights on and ZT19-lights off). Tshb mRNA expression in the PD varied during the day, peaking at ZT7 and dropping at ZT22 (Fig. 4A). CK1α protein levels also fluctuated during the daytime, with higher levels at ZT22 than at ZT7 (Fig. 4B). These results show that Tshb mRNA and CK1α protein expressions levels peaked at opposite phases of the 24-h light:dark cycle.
Figure 4: CK1α-PKC/ERK/CREB and CK1α-Wnt/β-catenin signaling pathways are associated with the rhythm of mice PD-TSH synthesis in mice. (A) Tshb mRNA fluctuations analyzed using RT-qPCR at ZT7, ZT18, and ZT22, with Gapdh used for normalization; n = 3. (B) Relationship between the circadian fluctuation of pituitary CK1α and TSH levels in mice, detected with WB. The internal loading control was α-tubulin; n = 3. *p < 0.05, ns = p > 0.05; n = 3. (C, D) Mouse pituitary tissues were collected at different time points (ZT7, ZT18, and ZT22), and the proteins involved in regulating TSH synthesis were detected by WB; p-PKC, p-ERK1/2, p-CREB, p-GSK3β, active-β-catenin, and p-β-catenin levels were normalized to total PKC, ERK1/2, CREB, GSK3β, and β-catenin levels, respectively. *p < 0.05, **p < 0.01, ns = p > 0.05; n = 3.
The PKC/ERK/CREB signaling pathway regulates pituitary TSH synthesis, and previously, we showed that CK1α modulates this signaling cascade to regulate TSH synthesis [19]. CK1α is a component of the β-catenin degradation complex and plays a negative regulatory role in Wnt/β-catenin signaling [23,24]. Therefore, we used WB to investigate circadian-related phosphorylation of key proteins in the PKC/ERK/CREB and Wnt/β-catenin signaling pathways. Phosphorylated PKC, ERK, and CREB levels in the mouse pituitary were significantly higher during the day (7:00) than at night (22:00) (Fig. 4C). Phosphorylated GSK3β in the Wnt pathway did not exhibit significant circadian variation, but ratios of active β-catenin and phosphorylated β-catenin to total β-catenin downstream of CK1α were significantly higher at night than during the day (Fig. 4D). Therefore, the activation of the CK1α-PKC/ERK/CREB and CK1α/Wnt/β-catenin signaling pathways is associated with the circadian rhythm of murine pituitary TSH synthesis.
Melatonin promotes PD-TSH synthesis via the CK1α/PKC/ERK/CREB pathway
We then examined the effect of melatonin on the PKC/ERK/CREB and Wnt/β-catenin signaling pathways. Results from WB indicated that exogenous melatonin enhanced p-PKC, p-ERK1/2, and p-CREB protein expression in PD (Fig. 5A) but had no effect on the mediators of Wnt/β-catenin signaling (Fig. 5B).
Figure 5: Melatonin promotes PD-TSH synthesis by CK1α/PKC/ERK/CREB (A, B) Effect of melatonin (6 μg/100 μL) on protein expression in PKC/ERK/CREB and Wnt signaling pathways. Relative p-ERK1/2, p-CREB, p-GSK3β, active-β-catenin, and p-β-catenin levels were normalized to total PKC, ERK1/2, CREB, GSK3β, and β-catenin levels, respectively. *p < 0.05, ***p < 0.001, ns = p > 0.05; n = 5. (C) Protein components of the PKC/ERK/CREB pathway were analyzed after pre-incubation with 10 μM pyrvinium or pyrvinium followed by 10 µM melatonin treatment for 10 min in primary cultured murine pituitary cells. PKC, ERK, and CREB were internal loading controls. *p < 0.05, **p < 0.01, ns = p > 0.05; n = 5.
CK1α regulates the production of TSH by modulating the PKC/ERK/CREB pathway in pituitary tissues [19]. We verified whether melatonin affected PD-TSH synthesis in a CK1α-dependent fashion by acting on the PKC/ERK/CREB signaling pathway. After pre-treating primary pituitary cells with pyrvinium for 6 h, followed by 10 min of melatonin treatment, we observed that pyrvinium blunted melatonin-induced upregulation of phosphorylated PKC and CREB levels (Fig. 5C).
Here, we successfully demonstrated that melatonin regulates TSH synthesis in the PD by downregulating cytoplasmic CK1α activity and upregulating the PKC/ERK/CREB signaling pathway (Fig. 6).
Figure 6: Melatonin regulates PD-TSH transcription via CK1α. Melatonin activates PKC through the inhibition of CK1α, increasing ERK1/2 phosphorylation, activating CREB, and promoting TSH transcription. The arrows indicate stimulation, and the thicker bar indicates inhibition; the lightest bar indicates nullification of inhibition.
The mRNA transcription and alpha chain synthesis, but not secretion, of a TSH-like product can be regulated by melatonin [2]. Reference [2] also reported that there may be two methods by which MLT regulates the function of PD. First, melatonin may affect the PT-hypothalamic axis; second, it may have anterograde action involving the PT and PD. Melatonin regulates the synthesis and secretion of PRL in the PD by inhibiting the release of one or more PRL-releasing factors (i.e., “tubulin”) from the PT [25]. Conversely, there is evidence that melatonin can also affect the secretion of a TSH-like product from the PT in a non-photoperiodic manner [3]. Melatonin administration inhibits Tshb mRNA expression in the PT of photoperiod-sensitive mice [4]. Other research on rodent has shown that high melatonin levels inhibit the mitotic activity of thyroid follicular cells. Melatonin also inhibits T4 levels, reducing the feedback effect of thyroid hormones on TSH levels [26,27]. While these findings suggest the importance of melatonin, they provide little insight regarding the mechanisms underlying the regulation of PD-TSH synthesis by non-photoperiodic melatonin in the pituitary gland. It is reported that melatonin can increase the expression of GH and PRL in primary pituitary cells of adult female baboons and release them in a dose-dependent manner, but does not affect the expression or release of ACTH or TSH [28]. The evidence in our study showed that non-photoperiodic melatonin can also affect the synthesis of PD-TSH in mouse pituitary and primary pituitary cells.
Our previous study found that CK1α affects TSH synthesis in the PD [19]. In this study, circadian variations in CK1α and Tshb mRNA levels peaked at opposite phases of the 24-h light:dark cycle. CK1α is a component of the β-catenin degradation complex and plays a negative regulatory role in Wnt/β-catenin signaling [23,24]. It has been reported that several genes involved in the Wnt/β-catenin signaling pathway are also photoperiodically regulated [17] In addition, the expression of genes encoding TSH subunits is regulated by CREB [29]. Our results regarding the expression patterns of key proteins (p-PKC, p-ERK, p-CREB, active β-catenin, and phosphorylated β-catenin) in TSH rhythm clearly show that the Wnt and PKC signaling pathways are involved in photoperiodic pituitary PD-TSH synthesis.
MLT is highly lipophilic and passes rapidly through the cell membrane [30]. Additionally, receptor-dependent pathway mainly involves melatonin binding to intracellular proteins, nuclear receptors, and membrane receptors; it appears to be the main mechanism of melatonin action on in mammals [31]. Notably, in this study, immunofluorescence staining showed that 45% of MT1 and TSH-β signals co-localized. Therefore, we speculated that MLT could act on pituitary PD-TSH cells via MT1. MLT lowers STOC and Ca2+ spark amplitude through the melatonin MT1/MT2 receptors PLC/PKC signaling pathway. Large-conductance Ca2+-activated K+ (BKCa) channels are abundantly expressed in vascular smooth muscle cells and play an important role in vascular tone regulation [32]. We further studied the effects of daily subcutaneous injection of melatonin for 3 weeks on the expression of key proteins in PKC/ERK/CREB and Wnt signaling pathways. Surprisingly, exogenous melatonin activated the pituitary PKC/ERK/CREB signaling pathway, but had no significant effects on the Wnt pathway, but had no significant effects on the Wnt pathway. It is suggested that PKC signaling is involved in mouse pituitary PD-TSH synthesis in a non-photoperiodic manner.
Besides being activated by melatonin, both MT1 and MT2 are G-coupled transmembrane receptors involved in most signaling cascades [33,34]. In particular, MT1 activation promotes MAPK1/2 and ERK1/2 phosphorylation [34], critical to the regulation of TSH synthesis. In this study, we observed CK1α and MT1 colocalization in the PD. MLT has a short half-life [35]. In vitro cell experiments showed that melatonin could induce the response of the kinase CK1α and the cascade of the PKC/ERK/CREB pathway in a short time. Further, treatment of primary pituitary cells with the CK1α agonist pyrvinium could blunt the effect of melatonin on the expression of key proteins in the PKC/ERK/CREB signal pathway, thus affecting the synthesis of pituitary PD-TSH.
We have fully confirmed the effect of MLT on pituitary PD-TSH through PKC/ERK/CREB signaling pathway mediated by CK1α.
Some limitations of the study remain. The studies conducted on this topic have mainly use animal models. While animals provide valuable insights into biological processes, they may not fully apply to humans due to differences in physiology and regulation. Another limitation is the complexity of the regulatory mechanisms involved in the synthesis of TSH. While CK1α has been identified as a key in melatonin-mediated regulation, there may be other probable factors and pathways that have not been fully elucidated; focusing solely on CK1α overlooks other important contributors to TSH synthesis. Additionally, the studies conducted on this topic have mainly focused on the effects of melatonin in normal conditions. However, changes in metabolism and the environment, and the phenomenon of neuroendocrine biological clock are related to the activity of the suprachiasmatic nucleus of the hypothalamus and are closely related to the circadian rhythm of melatonin secretion. Melatonin synthesis and TSH regulation may be altered in various pathological conditions, such as thyroid and sleep disorders, which could potentially affect the regulatory role of melatonin and CK1α. Finally, we attempted to use the CRE luciferase reporter gene experiment to check the regulation of Tshb transcription in primary cells after melatonin treatment, but the transfection efficiency of primary cells was low, which hindered the related molecular experiments.
Acknowledgement: None.
Funding Statement: This work was supported by the Priority Academic Program Development of Jiangsu Higher Education Institutions (PAPD).
Author Contributions: All authors contributed to the study conception and design. Material preparation and data collection and analysis were performed by Bingjie Wang, Yewen Zhou, Tongjuan Niu, and Mengqing Yin. The first draft of the manuscript was written by Bingjie Wang and Sheng Cui. All authors commented on previous versions of the manuscript, and have read and approved the final version.
Availability of Data and Materials: The datasets generated during and/or analyzed during the current study are available from the corresponding author on reasonable request.
Ethics Approval: Animal studies were conformed to the ethical guidelines of the Helsinki Declaration, and were reviewed and approved by Yangzhou University’s Institutional Animal Care and Use Committees (IACUC) (SYXK2017-0044).
Conflicts of Interest: The authors declare that they have no conflicts of interest to report regarding the present study.
References
1. Mikami S, Miyasaka S, Taniguchi K. Light and electron microscopic immunocytochemistry of the pituitary gland of the tortoise. Arch Histol Cytol. 1985;48:373–88. doi:10.1679/aohc.48.373. [Google Scholar] [PubMed] [CrossRef]
2. Ciani E, Haug TM, Maugars G, Weltzien FA, Falcón J, Fontaine R. Effects of melatonin on anterior pituitary plasticity: a comparison between mammals and teleosts. Front Endocrinol. 2020;11:605111. doi:10.3389/fendo2020. [Google Scholar] [CrossRef]
3. Ikegami K, Liao XH, Hoshino Y, Ono H, Ota W, Ito Y, et al. Tissue-specific posttranslational modification allows functional targeting of thyrotropin. Cell Rep. 2014;9:801–10. doi:10.1016/j.celrep.2014.10.006. [Google Scholar] [PubMed] [CrossRef]
4. Ono H, Hoshino Y, Yasuo S, Watanabe M, Nakane Y, Murai A, et al. Involvement of thyrotropin in photoperiodic signal transduction in mice. Proc Natl Acad Sci USA. 2008;105:18238–42. doi:10.1073/pnas.0808952105. [Google Scholar] [PubMed] [CrossRef]
5. Bockmann J, Böckers TM, Vennemann B, Niklowitz P, Müller J, Wittkowski W, et al. Short photoperiod-dependent down-regulation of thyrotropin-alpha and -beta in hamster pars tuberalis-specific cells is prevented by pinealectomy. Endocrinol. 1996;137:1804–13. doi:10.1210/endo.137.5.8612518. [Google Scholar] [PubMed] [CrossRef]
6. Aninye IO, Matsumoto S, Sidhaye AR, Wondisford FE. Circadian regulation of Tshb gene expression by Rev-Erbα (NR1D1) and nuclear corepressor 1 (NCOR1). J Biol Chem. 2014;289:17070–77. doi:10.1074/jbc.M114.569723. [Google Scholar] [PubMed] [CrossRef]
7. Samanta S. Melatonin: an endogenous miraculous indolamine, fights against cancer progression. J Cancer Res Clin. 2020;146:1893–922. doi:10.1007/s00432-020-03292-w. [Google Scholar] [PubMed] [CrossRef]
8. Ahmad SB, Ali A, Bilal M, Rashid SM, Wani AB, Bhat RR, et al. Melatonin and health:insights of melatonin action, biological functions, and associated disorders. Cell Mol Neurobiol. 2023;43:2437–58. doi:10.1007/s10571-023-01324-w. [Google Scholar] [PubMed] [CrossRef]
9. Oishi A, Gbahou F, Jockers R. Melatonin receptors, brain functions, and therapies. Handb Clin Neurol. 2021;179:345–56. doi:10.1016/B978-0-12-819975-6. [Google Scholar] [CrossRef]
10. Wu YH, Zhou JN, Balesar R, Unmehopa U, Bao A, Jockers R, et al. Distribution of MT1 melatonin receptor immunoreactivity in the human hypothalamus and pituitary gland: colocalization of MT1 with vasopressin, oxytocin, and corticotropin-releasing hormone. J Comp Neurol. 2006;499:897–910. doi:10.1002/cne.21152. [Google Scholar] [PubMed] [CrossRef]
11. Nikolaev G, Robeva R, Konakchieva R. Membrane melatonin receptors activated cell signaling in physiology and disease. Int J Mol Sci. 2021;23:471. doi:10.3390/ijms23010471. [Google Scholar] [PubMed] [CrossRef]
12. Chen L, He X, Zhang Y, Chen X, Lai X, Shao J, et al. Melatonin receptor type 1 signals to extracellular signal-regulated kinase 1 and 2 via Gi and Gs dually coupled pathways in HEK-293 cells. Biochem. 2014;53:2827–39. doi:10.1021/bi500092e. [Google Scholar] [PubMed] [CrossRef]
13. Vanselow K, Kramer A. Role of phosphorylation in the mammalian circadian clock. Cold Spring Harb Symp Quant Biol. 2007;72:167–76. doi:10.1101/sqb.2007.72.036. [Google Scholar] [PubMed] [CrossRef]
14. Lam VH, Li YH, Liu X, Murphy KA, Diehl JS, Kwok RS, et al. CK1α collaborates with DOUBLETIME to regulate PERIOD function in the drosophila circadian clock. J Neurosci. 2018;38:10631–10643. doi:10.1523/JNEUROSCI.0871-18.2018. [Google Scholar] [PubMed] [CrossRef]
15. Buijs FN, León-Mercado L, Guzmán-Ruiz M, Guerrero-Vargas NN, Romo-Nava F, Buijs RM. The circadian system: a regulatory feedback network of periphery and brain. Physiol. 2016;31:170–81. doi:10.1152/physiol.00037.2015. [Google Scholar] [PubMed] [CrossRef]
16. Jiang S, Zhang M, Sun J, Yang X. Casein kinase 1α: biological mechanisms and theranostic potential. Cell Commun Signal. 2018;16:23. doi:10.1186/s12964-018-0236-z. [Google Scholar] [PubMed] [CrossRef]
17. Helfer G, Ross AW, Morgan PJ. Neuromedin U partly mimics thyroid-stimulating hormone and triggers Wnt/β-catenin signaling in the photoperiodic response of F344 rats. J Neuroendocrinol. 2012;25:1264–72. doi:10.1111/jne.12116. [Google Scholar] [PubMed] [CrossRef]
18. Mao L, Dauchy RT, Blask DE, Slakey LM, Xiang S, Yuan L, et al. Circadian gating of epithelial-to-mesenchymal transition in breast cancer cells via melatonin-regulation of GSK3β. Mol Endocrinol. 2012;26:1808–20. doi:10.1210/me.2012-1071. [Google Scholar] [PubMed] [CrossRef]
19. Wang BJ, Zhang JL, Zhang D, Lu CY, Liu H, Gao Q, et al. Casein kinase 1α as a novel factor affects thyrotropin synthesis via PKC/ERK/CREB signaling. Int J Mol Sci. 2023;24:7034. doi:10.3390/ijms24087034. [Google Scholar] [PubMed] [CrossRef]
20. Urbani C, Mattiello A, Ferri G, Raggi F, Russo D, Marconcini G, et al. PCB153 reduces apoptosis in primary cultures of murine pituitary cells through the activation of NF-κB mediated by PI3K/Akt. Mol Cell Endocrinol. 2021;520:111090. doi:10.1016/j.mce.2020.111090. [Google Scholar] [PubMed] [CrossRef]
21. Magliocco G, Le Bloc’h F, Thomas A, Desmeules J, Daali Y. Simultaneous determination of melatonin and 6- hydroxymelatonin in human overnight urine by LC-MS/MS. J Chromatogr B. 2021;1181:122938. doi:10.1016/j.jchromb.2021.122938. [Google Scholar] [PubMed] [CrossRef]
22. van der Spoel E, Roelfsema F, van Heemst D. Within-person variation in serum thyrotropin concentrations: main sources, potential underlying biological mechanisms, and clinical implications. Front Endocrinol. 2021;12:619568. doi:10.3389/fendo.2021.619568. [Google Scholar] [PubMed] [CrossRef]
23. Shen C, Nayak A, Melendez RA, Wynn DT, Jackson J, Lee E, et al. Caseink kinase 1α as a regulator of Wnt-driven cancer. Int J Mol Sci. 2020;21:5940. doi:10.3390/ijms21165940. [Google Scholar] [PubMed] [CrossRef]
24. He Z, Wang X, Zheng X, Yang C, He H, Song Y. Fam83h mutation causes mandible underdevelopment via CK1α- mediated Wnt/β-catenin signaling in male C57/BL6J mice. Bone. 2023;172:116756. doi:10.1016/j.bone.2023.116756. [Google Scholar] [PubMed] [CrossRef]
25. Dardente H, Wood S, Ebling F, Sáenz de Miera C. An integrative view of mammalian seasonal neuroendocrinology. J Neuroendocrinol. 2019;31:e12729. doi:10.1111/jne.12729. [Google Scholar] [PubMed] [CrossRef]
26. Wajs E, Lewiński A. Inhibitory influence of late afternoon melatonin injections and the counter-inhibitory action of melatonin-containing pellets on thyroid growth process in male Wistar rats: comparison with effects of other indole substances. J Pineal Res. 1992;13:158–66. doi:10.1111/j.1600-079X.1992.tb00071.x. [Google Scholar] [PubMed] [CrossRef]
27. Wright ML, Pikula A, Babski AM, Labieniec KE, Wolan RB. Effect of melatonin on the response of the thyroid to thyrotropin stimulation in vitro. Gen Comp Endocr. 1997;108:298–305. doi:10.1006/gcen.1997.6979. [Google Scholar] [PubMed] [CrossRef]
28. Ibáñez-Costa A, Córdoba-Chacón J, Gahete MD, Kineman RD, Castaæo RD, Luque RM. Melatonin regulates somatotrope and lactotrope function through common and distinct signaling pathways in cultured primary pituitary cells from female primates. Endocrinol. 2015;156:1100–10. doi:10.1210/en.2014-1819. [Google Scholar] [PubMed] [CrossRef]
29. Hashimoto K, Zanger K, Hollenberg AN, Cohen LE, Radovick S, Wondisford FE. cAMP response element-binding protein-binding protein mediates thyrotropin-releasing hormone signaling on thyrotropin subunit genes. J Biol Chem. 2000;275:33365–72. doi:10.1074/jbc.M006819200. [Google Scholar] [PubMed] [CrossRef]
30. Abdollahzade N, Majidinia M, Babri S. Melatonin: a pleiotropic hormone as a novel potent therapeutic candidate in arsenic toxicity. Mol Biol Rep. 2021;48:6603–18. doi:10.1007/s11033-021-06669-3. [Google Scholar] [PubMed] [CrossRef]
31. Yu H, Dickson EJ, Jung SR, Koh DS, Hille B. High membrane permeability for melatonin. J Gen Physiol. 2015;147:63–76. doi:10.1085/jgp.201511526. [Google Scholar] [PubMed] [CrossRef]
32. Xu Z, Wu Y, Zhang Y, Zhang H, Shi L. Melatonin activates BKCa channels in cerebral artery myocytes via both direct and MT receptor/PKC-mediated pathway. Eur J Pharmacol. 2019;842:177–88. doi:10.1016/j.ejphar.2018.10.032. [Google Scholar] [PubMed] [CrossRef]
33. Boiko DI, Shkodina AD, Hasan MM, Bardhan M, Kazmi SK, Chopra H, et al. Melatonergic receptors (Mt1/Mt2) as a potential additional target of novel drugs for depression. Neurochem Res. 2022;47:2909–24. doi:10.1007/s11064-022-03646-5. [Google Scholar] [PubMed] [CrossRef]
34. Chen M, Cecon E, Karamitri A, Gao W, Gerbier R, Ahmad R, et al. Melatonin MT1 and MT2 receptor ERK signaling is differentially dependent on Gi/o and Gq/11 proteins. J Pineal Res. 2020;68:e12641. doi:10.1111/jpi.12641. [Google Scholar] [PubMed] [CrossRef]
35. Kim HK, Yang KI. Melatonin and melatonergic drugs in sleep disorders. Transl Clin Pharmacol. 2022;30:163–71. doi:10.12793/tcp.2022.30.e21. [Google Scholar] [PubMed] [CrossRef]
Supplementary Materials
Cite This Article
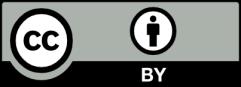
This work is licensed under a Creative Commons Attribution 4.0 International License , which permits unrestricted use, distribution, and reproduction in any medium, provided the original work is properly cited.