Open Access
REVIEW
Mesenchymal stem cells and the angiogenic regulatory network with potential incorporation and modification for therapeutic development
1 Stem Cell Institute, University of Science Ho Chi Minh City, Ho Chi Minh City, Viet Nam
2 Viet Nam National University Ho Chi Minh City, Ho Chi Minh City, Viet Nam
3 Laboratory of Stem Cell Research and Application, University of Science Ho Chi Minh City, Ho Chi Minh City, Viet Nam
* Corresponding Author: PHUC VAN PHAM. Email:
(This article belongs to the Special Issue: Perspectives on Stem Cells and Regenerative Medicine)
BIOCELL 2024, 48(2), 173-189. https://doi.org/10.32604/biocell.2023.043664
Received 14 July 2023; Accepted 23 October 2023; Issue published 23 February 2024
Abstract
Mesenchymal stem cells (MSCs) have been proposed in regenerative medicine, especially for angiogenic purposes, due to their potential to self-renew, differentiate, and regulate the microenvironment. Peripheral vascular diseases, which are associated with reduced blood supply, have been treated but not cured. An effective therapy is to recover blood supply via vessel regeneration in the affected area, and MSCs appear to be promising for such conditions. Several studies aimed to explore the role of MSCs in performing angiogenesis and have revealed numerous potential methods to enhance MSC capacity in vessel formation. Efforts have been made to modify standard MSCs to optimize their prospective characteristics. Thus, understanding the mechanism underlying MSC angiogenic potential, and learning how to control their effect in the in vivo environment is critical for success in clinical settings. This review covers the angiogenesis process and discusses the role of some of the key angiogenic regulators. Possible techniques to enhance the angiogenic capacity of MSCs are also mentioned to suggest potential methods for therapeutic application.Graphic Abstract
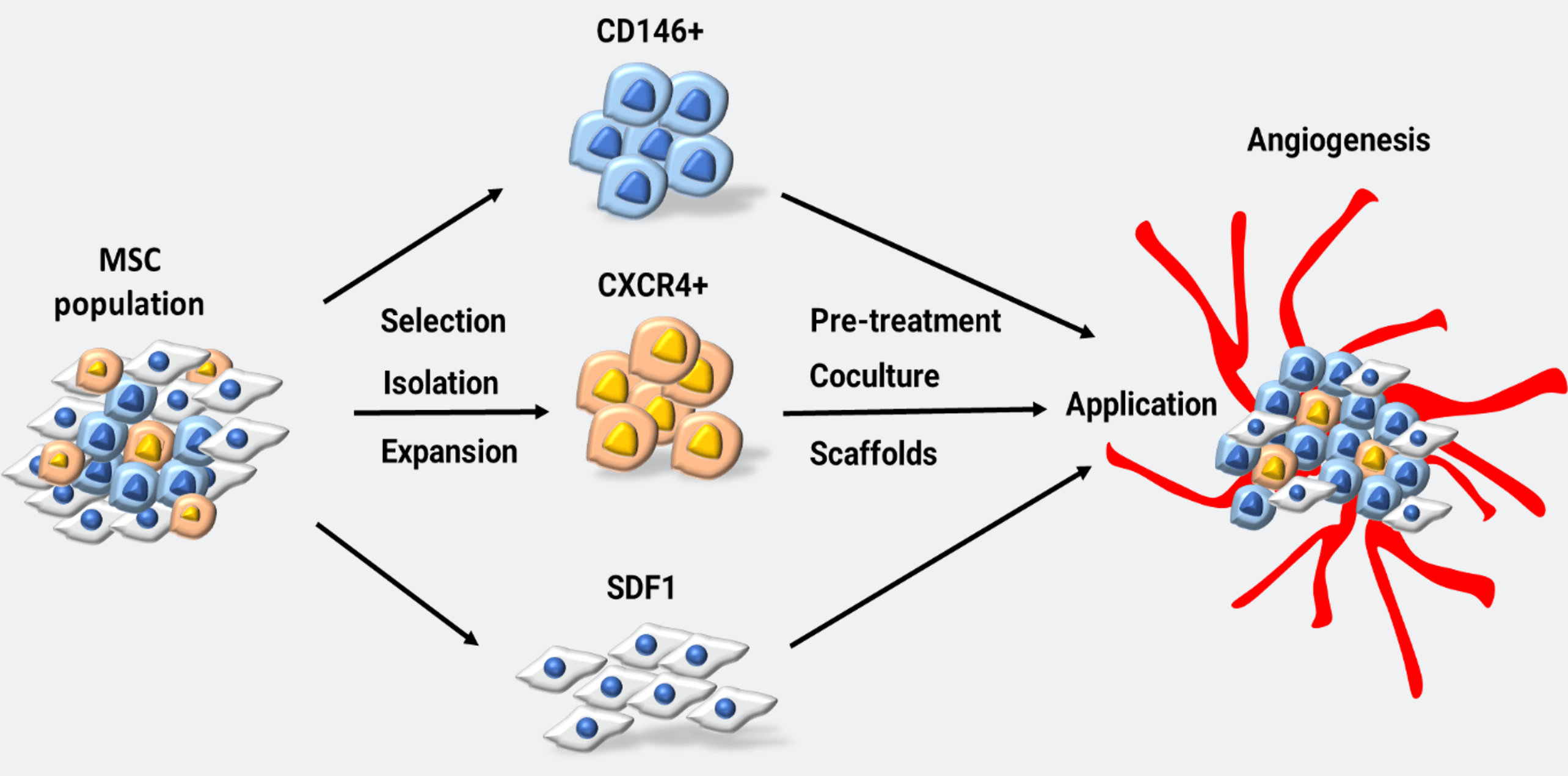
Keywords
The success of regenerative medicine relies on the survival of the newly formed tissues, which must be able to maintain their function in the long term. Therefore, it is important to supply the tissues with sufficient oxygen and nutrients and to remove waste from functional activities. In other words, newly formed tissues must be supplied with a vascular system or blood vessels to survive. The potential to form blood vessels, or so-called angiogenesis, is not only limited to regenerative medicine, but is also meaningful in diseases related to the peripheral vascular system, such as ischemia. In ischemic conditions, blood flow is blocked or prevented in specific regions, resulting in restricted blood supply to the following tissues. In some severe conditions, the restriction of blood supply can cause tissue dysfunction or even tissue death. Thus, a therapeutic approach that can enhance angiogenesis in vivo holds great potential in improving human health. Angiogenesis is referred to as the formation of new blood vessels or the enhancement of vascular branching, which can also be considered as vessel sprouting.
In the search for therapy, mesenchymal stem cells (MSCs) have been shown to be effective in regulating angiogenesis [1–3]. Several studies have been conducted in vitro and in vivo to examine MSCs from different origins and their ability to enhance blood vessel generation [4–7]. In our previous review, we addressed key challenges that hindered the success of cell therapy in the in vivo microenvironment [8]. The application of MSCs for vascular diseases requires overcoming such challenges and performing strict regulation and control over cellular activities, which can be achieved by improving the understanding of how cell functions in the microenvironment. This review will focus on current knowledge of MSC application in angiogenesis and neovascular generation. Blood vessels are formed by the construction of endothelial cells (ECs), which are derived from endothelial precursor cells (EPCs). Phenotypic changes of ECs are largely supported by the regulatory network, under the effect of which, ECs arrange and function distinctively. Those factors that are involved in the regulation of ECs during angiogenesis are important for the formation of a functional vessel when completed. The balance between different growth factors on the activity of ECs is essential for controlling the angiogenic process [9]. The role of MSCs in the formation of blood vessels has been evaluated, which reveals that MSCs can be used by exploiting various cell potentials, including differentiation, chemotaxis, proliferation, and recruitment of ECs to the damaged sites. With the current knowledge and technology in stem cell application, the search for MSC populations to be used in forming new blood vessels appears approachable. Several studies have shown the potential role of different MSC subpopulations in vasculogenesis and angiogenesis [10]. However, challenges are met when MSC transplantation in vivo is limited, with a low survival rate, acute donor cell death, and low proliferation of the grafted cells [11]. It is also found that MSCs hardly differentiate into ECs when injected into a hypoxic area to perform angiogenesis; however, its role in supporting angiogenesis has been shown to be sufficient [12]. Although the combination of cells and drugs appears to increase the effect in treatment, limited information is available about the types of cells and the dosage of drugs, posing a gap in therapeutic intervention. Thus, it is critical to have a better selection of MSCs with a high ability to perform vessel regeneration. In this review, different types of MSC subpopulations effective in supporting angiogenesis and vasculogenesis will be discussed with the regulatory network. Other efforts put into enhancing the angiogenic potential for the application of MSCs in vivo will also be mentioned. Possible incorporation of multi-step preparation of MSCs will also be suggested for future research.
Endothelial Cells and the Role in Angiogenesis
ECs are the main type of cells found in the inside lining (luminal surface) of blood vessels, lymph vessels, and the heart. The single layer of ECs is called endothelium. ECs are derived from mesoderm. Mesodermal stem cells differentiate into hemangioblast, which then generate hematopoietic stem cells and angioblast. Angioblast is known as a source of endothelial progenitor cells, which can differentiate into blood vessel ECs, depending on the regulation of specific transcription factors [13,14]. ECs link to each other to form a barrier between blood and tissues that allows molecules to exchange selectively. There are three main types of endothelial intercellular junctions, including tight junctions, adherent junctions, and gap junctions [15,16]. Tight junctions are related to paracellular permeability regulation and cell polarity. The adherent junctions contribute to controlled cell growth via the contact inhibition mechanism, and support transendothelial migration of white blood cells and permeability of plasma protein in inflammation reaction [17,18]. Gap junctions are described as channel-like structures, that serve as communication structures, allowing the movement of small soluble signal molecules, such as Ca2+ and inositol 1,4,5-trisphosphate, between neighboring cells [19].
ECs play an important role in blood vessel formation. During the vessel formation process, ECs change their morphology and migrate and proliferate to extend or branch the vascular network. In sprouting angiogenesis, specific ECs with filopodia called tip cells, work as the guidance cells, migrating towards vascular endothelial growth factor A (VEGF-A) gradient and promote the division of other cells following ECs (stalk cells) [13,20]. The endothelium also secretes platelet-derived growth factor (PDGF) as a paracrine signal to recruit MSCs and smooth muscle cell progenitors to the newly formed vessel, which is necessary for vascular maturation and function [21].
ECs also contribute to vascular tone regulation by releasing vasodilators and vasoconstrictors, interacting directly with the underlying vascular smooth muscle cells. Vasodilators produced by ECs, such as nitric oxide (NO), prostacyclin, and endothelium-derived hyperpolarizing factor induce smooth muscle cell relaxation that results in increasing blood flow. In contrast, the smooth muscle cells contract under the impact of vasoconstrictive factors such as thromboxane and endothelin-1, which narrows blood vessels and raises blood pressure [22,23]. The dysfunction of these regulatory factors is associated with cardiovascular diseases such as hypertension, atherosclerosis, heart attack, and stroke [24]. Transendothelial migration is an initiative process during an inflammatory response, in which white blood cells, especially neutrophils and monocytes, migrate through the vessel wall into the inflamed area. In response to infection, activated macrophages secrete cytokines such as tumor necrosis factor-alpha and interleukin 1, which induce EC to express adhesion molecules such as selectin (the ligand of integrin), vascular EC adhesion molecule 1, and produce specific cytokines [25]. White blood cells are activated by these cytokines before crawling along the inflammation chemokine gradient based on the interaction with EC adhesion. White blood cells can utilize two ways to come out of blood vessels, paracellular and transcellular routes. The paracellular route refers to the migration of white blood cells via unstable EC junctions, while the transcellular route occurs directly through the cell body [21,25]. These data indicated that ECs play an important role in angiogenesis and are involved in vessel formation and vascular function.
Angiogenesis is an important biological and physiological process inside the human body, occurring in embryogenic development, wound healing, chronic inflammation, and malignant solid tumor development; this process is also crucial for both tissue development and regeneration [26,27]. The formation of new blood vessels occurs in two different fashions: 1/ the appearance of newly formed vessels as a requirement for development, and 2/ the branching of existing vessels. In ischemic conditions, the formation of new vessels from the pre-existing vessels is important to replenish the blood supply to the ischemic sites of the tissues, which, in many cases, may not fulfill the need of local tissues [28]. During angiogenesis, ECs need to escape quiescence, then proliferate, migrate, and undergo tubulogenesis to form functional vessels [29]. Four major sequential events are involved in a complete angiogenic process, which includes sprouting, vessel formation, adaptation to tissue needs, and vascular stabilization [29]. Tip cells sprouting from the main vessels function as guidance cells that interact with the environment to recruit Ecs and mural cells [28]. The angiogenic factors produced from the sprouting area form active gradients that attract ECs migrating to the sites of targets to create vessel walls [29]. During the early stage, growth factors including vascular endothelial growth factor (VEGF) and fibroblast growth factor (FGF) at the target site act to stimulate Ecs to migrate to the target site and induce the secretion of plasminogen activators (Pas), collagenase and matrix metalloproteinase (MMP) to assist Ecs migrating through the existing vessel wall [30,31]. Once Ecs start sprouting, the production of αVβ3-integrins supports cell-cell contact, which further assists the adhesion and migration of Ecs along the new vessel branch [32,33]. Tube formation follows the migration of ECs and is stabilized by the presence of angiopoietin 1 (Ang 1) and Tie 2 [34,35]. Pericyte precursors or MSCs come to the new vessel branch, where they proliferate and differentiate to form mature pericytes under the trigger of PDGF expressed by ECs [36]. Finally, the new vessel is stabilized by the quiescence of ECs, where the cell-cell contact is strengthened, and the new matrix is formed [37].
Mesenchymal stem cells and the potential in angiogenesis
Being considered endothelial cell precursors, MSCs pose potential characteristics to support the angiogenic process. MSCs are multipotent stem cells, which allows the cells to be infinitely expanded in the culture of selected media, while maintaining cell stability and the ability to differentiate into numerous cell types. MSCs can be derived from several tissues, including adipose tissue, umbilical cord, umbilical cord blood, placental membrane, dental tissues, and many more [38]. With different types of tissues, various MSC derivation methods were optimized and published, generating vast knowledge to obtain MSCs efficiently. In addition, the ability to identify and characterize MSCs is also a major concern. However, the current characterization method of MSCs is limited and controversial, which mostly depends on the early published criteria from the International Society for Cellular Therapy [39]. Depending on the origins of the tissues, which the cells are derived from, MSCs are often a mixed population of heterogenous cells with different capacities due to their distinct transcriptomic and proteomic profiles [40–42].
Mesenchymal stem cells enhance angiogenesis via paracrine effects
MSCs have the advantage of immunity modulation, which proposes them for several applications in research and treatment [43,44]. Stem cells often repair damaged tissue primarily through the paracrine mechanism, especially via the secretion of exosomes [45]. For instance, MSCs derived from bone marrow-secreted exosomes that enhance MSC migration and the expression of VEGF, ANG1, and ANG2 genes, are angiogenic-related genes [46]. The ability of MSCs to enhance vascular regeneration was found to be related to VEGF [3]. The Sonic hedgehog signal, a morphogen present in Wharton’s jelly and responsible for angiogenesis during the postembryonic stage, was found to support Wharton’s jelly MSCs (WJ-MSCs) to express VEGF in hypoxic settings in vitro [47]. In WJ-MSCs, the activity of netrin-1, a ligand related to embryonic development, contributed to the potential of vascular regeneration by stimulating EC migration and possibly pro-angiogenic response [48]. In addition, recent studies that combined exosomes from MSCs with hydrogel have shown angiogenic efficacy in treating ischemia via reducing EC apoptosis and enhancing EC proliferation and pro-angiogenic factors, such as VEGF, ANG1, ANG2, and eNOS [41,49]. The combination of human platelet lysate and MSCs has been shown to increase the effectiveness of ischemic treatment [50]. Genetic modifications of MSCs were found to increase the effectiveness of ischemic treatment in mice hind-limb ischemia by increasing Gremlin1 expression (GREM1), which increased cell survival rates compared to non-transgenic MSCs [51]. The potential of MSCs in treating ischemic diseases has also been evaluated in ischemic stroke via enhancing angiogenesis in the ischemic boundary area [52]. In contrast to the ability to stimulate angiogenesis, MSC exosomes were shown to prevent angiogenesis in tumors [53]. Even though the precise mechanism of how MSC exosomes support angiogenesis remains largely unexplored, these evidences suggest a potential role of MSCs in treating diseases associated with low blood supply conditions via their ability to modulate angiogenesis, mostly through the increased expression of pro-angiogenic factors, making MSCs a promising cell therapy for such diseases. The involvement of MSCs in the angiogenic process is described in Fig. 1.
Figure 1: MSC selection and possible methods for angiogenic purpose. MSCs can be selected and isolated for specific subpopulations. These subpopulations are then expanded to form pure cell sources. From the available cell sources, other techniques such as pre-treatment, co-culturing and scaffolds can be implied enhance angiogenic capacity for application (MSC: mesenchymal stem cells).
Subpopulations of mesenchymal stem cells supporting angiogenesis
Isolation of MSC subpopulations supporting angiogenesis has been done in various studies, aiming to select suitable candidates for therapeutic application. In this section, we highlighted some of the promising MSC subpopulations that may be effective in enhancing and regulating angiogenesis, either alone or in combination with other priming methods (Fig. 2).
Figure 2: MSCs support angiogenic process via paracrine effects. Tip cells sprouting from the main vessel mark the formation of new vascular branch. Tip cells produce growth factors including VEGF, FGF, Pas, MMP to direct the migration of stalk cells and EC cells and mediate stalk cell proliferation. ANG1 and TIE-2 are secreted at the new site to attract ECs to migrate to the new vessel branch. PDGF is secreted by tip cells to prevent ECs from forming new tip cells, ECs then produce PDGF to recruit precursor cells or MSCs to form pericytes, stabilising the new vessels. MSCs produce a variety of pro-angiogenic factors to the microenvironment to regulate vessel formation and ensuring the stabilisation of new vessels. (VEGF: vascular endothelial growth factor; MSCs: mesenchymal stem cells; FGF: fibroblast growth factor; MMP: matrix metalloproteinase; ANG-1: angiopoietin 1; TIE-2: tyrosine kinase receptor; ECs: endothelial cells).
CD146+ mesenchymal stem cells CD146 emerged as an endothelial biomarker and is a target molecule of angiogenesis-related diseases. CD146 plays a fundamental role in angiogenesis [54]. CD146 marker has 2 isoforms: shCD146 and lgCD146. A study of ischemic disease has shown that shCD146 isoform increased the angiogenic potential of EPC both in in vitro and in vivo studies [55]. The role in angiogenesis of CD146 was shown by the knockdown of CD146 via CD146 siRNA, which led to reduced cell turnover and proliferation of cells [56]. In a study of pulmonary arterial hypertension (PAH), under hypoxic conditions, hypoxia inducible factor-1α (HIF-1α) regulated the expression of CD146 marker, which in turn, promoted HIF-1α replication via NF-kB, leading to angiogenesis in pulmonary hypertension [57]. In an ischemic study, it was shown that stem cell properties of peripheral blood endothelial progenitor cells stimulated by soluble CD146 via miRNA-21 improved ischemia in mice [58]. Another study in mice on hind-limb ischemia disease showed that sCD146 exhibited angiogenic properties and promoted angiogenesis [59]. sCD146 also enhanced the therapeutic capacity of endothelial progenitor cells and aided in angiogenesis by activating the proteolytic process of shCD146 [60]. These findings reinforce the important role of sCD146 in angiogenesis.
In studying the role of CD146 in vascular regeneration, MSCs can be considered a promising candidate for research and treatment because of their superiority. The expression of CD146 in MSCs has been described in numerous studies [61,62]. The function of CD146 in MSCs also differs depending on its high or low expression level. In a study of human MSCs, an increase in expression of CD146 increased cellular motility, whereas a decrease in expression of CD146 increased bone regeneration [63]. Another study showed the role of CD146 MSCs in bone regeneration [64]. In a recent study, the transplantation of MSCs expressing CD146 significantly increased capillary density in mice with ischemic limbs when compared with non-transgenic MSCs, the mechanism of which was related to the stimulation of VEGF expression in muscles of the affected mice [10]. CD146 is considered to be an important biological marker in the application of the treatment of several human conditions [65]. On the other hand, the overlap in the expression and function of CD146 in each pathology is different, leading to the adjustment of CD146 capability in application. For application in therapeutic development, signaling pathway or factors associated with CD146 needs to be explored to better understand this particular biomarker.
C-X-C chemokine receptor type 4 (CXCR4+) mesenchymal stem cells
CXCR4 receptor is a cell surface receptor that plays an important role in angiogenesis [66]. CXCR4 supports MSCs to move to the medulla via a homing mechanism [67]. The stromal cell-derived factor 1α (SDF-1α) and its receptor CXCR4 signaling pathway is a commonly known pathway that contributes to vascular regeneration in ischemic pathologies [68]. The SDF-1α/CXCR4 signaling pathway was shown to support blood vessel formation in the retina [69]. An experiment on a rat model of brain damage due to hypoxia-ischemic brain lesion showed that SDF-1α/CXCR4 axis mediated the movement of MSCs to the wounded location [70]. The SDF-1α/CXCR4 axis also plays an important role in promoting HP-MSC (hypoxic) treatment of renal ischemia/reperfusion injury [71]. There has been research showing that under hypoxic conditions, CXCR4 expression is increased by activating HIF-1α in MSCs [72]. The movement of MSCs from umbilical cord blood in humans was found to be related to SDF-1α/CXCR4 axis via Akt, ERK, and p38 signaling pathways [73]. The presence of CXCR4 on MSCs increased the ability of MSCs to move to the site of injury [74]. A study of coronary artery disease showed that the decrease in expression of CXCR4 led to a decrease in the ability of new endothelial progenitor cells to form new blood vessels [75]. G-CFS granulocyte stimulation factor also supported SDF-1α/CXCR4 axis to mobilize bone marrow-derived CXCR4+ cells to the site of heart damage after myocardial infarction [76]. In another study, overexpression of CXCR4 on MSCs also promoted angiogenesis to improve ischemic heart damage, most probably through the paracrine effects, as MSCs with high levels of CXCR4 showed increased expression of VEGF, Cyclin A2, and transforming growth factor (TGF)-β2 [4]. The potential of CXCR4+ MSCs has several benefits in treating ischemic diseases. However, during the expansion of the MSCs culture, it often happened to have decreased expression or even loss of expression of CXCR4. Recently, a research team increased CXCR4 expression in MSCs through intravenous transfusion and showed an improvement in injectable muscle infarction through noninvasive treatment [77]. Recognizing the potential of the SDF-1α/CXCR4 axis, a research team recently developed a technique to attach recombinant CXCR4 onto the surface of MSCs to improve the treatment of ischemic diseases [78]. Although the potential of the SDF-1α/CXCR4 axis in studies of vascular regeneration or MSCs is promising, many studies have shown the contribution of the SDF-1α/CXCR4 signaling pathway in metastatic carcinoma [79,80]. Therefore, further study of the CXCR4 receptor and its signaling pathways is necessary to apply their potential benefits in the treatment with stem cell therapy.
Stromal cell-derived factor 1α expressing mesenchymal stem cells
SDF-1 is the chemotactic factor (also known as CXCR-12) that plays an important role in migration, proliferation, cell survival, and other specific tissue functioning [81,82]. The role of SDF-1 in angiogenesis has been indicated both in vitro and in vivo research [83]. The SDF-1/CXCR4 axis was found to be involved in the migration and homing of MSCs to the ischemic or injury site [84,85], and was shown to promote the secretion of pro-survival and angiogenic factors in MSCs in response to hypoxic conditions [86]. Data from these studies proposed a potential use of SDF-1 expressing MSCs in therapeutic development, which could be achieved simply by incubating MSCs in hypoxic conditions or pre-treating the cells with SDF-1 in vitro. MSCs modified with transfected SDF-1 has been shown to enhance capillary formation in mouse infarcted heart and improve heart function in the treated mice [82]. Overexpression of SDF-1 in MSCs was also shown to be effective in promoting ECs in microvascular regeneration as co-culture of SDF-1-overexpressed MSCs with ECs showed a significant increase in tube formation, and SDF-1 content in the exosomes of these MSCs was suggested to perform similar effects, most probably via PIK3 pathway [83]. Selection of the MSC subpopulation with high expression of SDF-1 would be promising for future application in angiogenesis.
Efforts to Enhance Mesenchymal Stem Cell Potential in Angiogenesis
Pre-treatment of mesenchymal stem cells
Pre-treatment of MSCs with different chemicals and reagents is a widely exploited method to push the angiogenic potential of MSCs. For example, at low concentrations, the application of Carvacrol, a monoterpene phenol that can be found in herbs, was shown to enhance the VEGF expression of MSCs [87]. Particularly, after 48h incubation, MSCs treated with 25 μM Carvacrol showed significantly increased expression of endothelial factors such as VE-cadherin and vWF, and enhanced angiogenic paracrine effect when co-cultured with HUVECs [87]. Apple extract is another plant ingredient that was found effective in enhancing paracrine effects in MSCs. When MSCs were treated with 1% apple extract for 3 days, the expression of VEGF was significantly increased in the cells, which was correlated with increased mTOR activity [88]. As mTOR is a major cell signaling responding to environmental metabolites and connecting cell metabolism with cell growth [89], this evidence may represent a close relationship between the paracrine effect and MSC metabolism. In fact, Deschepper and colleagues showed that treatment targeting MSC metabolism, such as the use of glucose, was important for angiogenic potential in MSC culture in extremely hypoxic or near-anoxic conditions [90]. The addition of glucose over 0.1 g/L could enhance angiopoietin-2 and VEGF-C expression in MSCs after 3 and 7 days of exposure to near-anoxic conditions, and when ectopically implanted in a mouse model, MSCs with glucose treatment showed significant vascularisation on mouse skin [90].
Pre-treatment of MSCs with different types of hormones was also sufficient in promoting angiogenic potential [91–93]. Steroid hormones are important for angiogenesis during pregnancy, which is required to support fetal development [94–96]. Estrogen, for example, is a sex steroid known to enhance the proliferation and migration of ECs, which was shown to stimulate the expression of angiogenic factors and adhesion molecules in the uterine and vascular tissues, and triggered the release of NO by the vascular cells [96]. Growth hormones such as angiotensin II, endothelin, growth hormone-releasing hormone or vasopressin have been shown to exert different effects on angiogenesis by regulating the expression of pro-angiogenic and angiogenic factors such as PDGF, ANG-2, VEGF, and hepatocyte growth factor [97]. Therefore, the use of hormones is expected to stimulate and enhance the angiogenic potential of MSCs. Mihai and colleagues showed that when MSCs were cultured with 17β-estradiol (E2) for 4 days, the expression of angiogenic genes, including VEGF and ANG, was significantly increased [91]. This was correlated with the release of NO and enhanced angiogenesis of MSCs in animal models [91]. Another study reported that the use of dihydrotestosterone (DHT) significantly induced the production of NO and the expression of angiogenin and MMP-9 in MSCs, and DHT-treated MSCs when co-cultured with cardiac tissue slices showed a significant increase in VEGF and MMP-2 expression [92]. Ma et al. (2016) used the growth hormone-releasing hormone agonist, JI34, have found that MSCs treated with JI34 showed enhanced migration and paracrine effect on angiogenesis with the increase in SDF-1 and VEGF-A expression, which was correlated with the activation of STAT3 [93]. In in vivo studies, the authors demonstrated that cell therapy using JI34-treated MSCs improved vascularisation and increased ECs recruitment to ischemic hind-limbs in mice, which was also associated with faster recovery and reduced tissue damage [93]. This evidence suggested that hormones could be potential candidates for enhancing MSC angiogenic capacity. However, the precise mechanism of action remains unresolved, raising questions about the safety of priming MSCs in sex hormones, as these hormones are regulators of multiple cell pathways, including the pathogenesis of cancers [98,99]. Several other types of treatment and stimulation are used with an effort to enhance the angiogenic potential of MSCs in culture (Table 1), which indicates that the selection of the right stimuli may be important to promote MSCs as a cell therapy for vascularisation. Further studies may be directed to understand the mechanism as well as the efficiency and safety of multiple types of stimulation on different MSC lines, which will help to select the best combination in improving MSC angiogenic ability.
Oxygen is an essential supplement to cells, and oxygen homeostasis plays a key role in cell survival. Oxygen serves as the last electron acceptor in the electron transport chain, which occurs in mitochondria to produce energy in eukaryotic cells. However, oxygen is also a strong oxidant and can produce harmful reactive oxygen species (ROS) that could damage nucleic acids and proteins, and lead to cell death [100]. Two conditions in terms of oxygen concentration are often used in research: a/ normoxia, the condition with oxygen tension similar to an air atmosphere, in which oxygen accounts for approximately 21% of the air, while b/ hypoxia, which is defined as the continuous or temporary condition lacking oxygen supply to tissue [101]. Hypoxic conditions could appear as a result of rapid cell proliferation, as in embryogenesis, tumorigenesis or vascular network disruption [102].
HIF-1 is an important transcription factor in hypoxic response. It is a heterodimeric complex, which is composed of two distinct subunits: a stable and constitutively expressed β-subunit (HIF-1β), formed by 774 amino acid residues, and an oxygen-regulated α-subunit (HIF-1α), which consists of 826 amino acid contributing to oxygen sensing. The two HIF-1 subunits belong to the basic helix-loop-helix Per-Arnt-Sim (bHLH-PAS) transcription factor family. Both HLH motif and PAS domain are necessary for DNA binding and subunit dimerization [101]. The genes related to hypoxic response are triggered when the HIF-1 heterodimeric complex binds to hypoxia response elements (HRE) situated in their promoters. Moreover, the transcriptional activation of these target genes requires the interaction of HIF-1α and the coactivators such as CBP, p300, SRC-1, and TIF2 via the amino-terminal and the carboxy-terminal transactivation domains (N-TAD and C-TAD) of HIF-1α [102,103]. The oxygen sensitivity of HIF-1α is determined by the oxygen-dependent degradation (ODD) domain. In normoxia, the prolyl residues in the ODD domain are hydroxylated by prolyl hydroxylases (PHD 1–3) followed by the binding of HIF-1α to the von Hippel–Lindau protein (VHL), ubiquitin ligase complex elongin B/C and recruiting a ubiquitin-conjugating enzyme which ubiquitinates HIF-1α. As a result, the marked HIF-1α is degraded by proteasome [101,104].
Angiogenesis could be stimulated by hypoxic conditions via the HIF-1 pathway. Several studies have demonstrated that HIF-1 enhanced the expression of several angiogenic genes, such as VEGF, PDGF, and angiopoietin 1 and 2 (Ang-1 and -2) [105,106]. Especially in the Matrigel plug assay, the loss of HIF-1α disrupted the VEGF-dependent migration and proliferation of murine ECs [107]. Besides, it was shown that the SDF1/CXCR4 chemotaxis was expressed higher in hypoxia-preconditioned MSCs and was upregulated in glioblastoma by HIF-1. Microarray analysis indicated the transcriptional regulation of hypoxic conditions and HIF-1 on multiple genes involved in the cell cycle, nucleic acid metabolism, and replication [106].
Hypoxia-induced angiogenesis is a potential approach for cardiovascular disease treatment, wound healing, and tissue engineering [108]. Understanding the regulatory mechanism of hypoxia has raised considerations for two distinct possible strategies. The first strategy relies on the transplantation of hypoxia-preconditioned cells, which express significant factors promoting angiogenesis [108]. In murine models of hind-limb ischemia, hypoxic preconditioned MSCs (cultured in 2% O2) exhibited efficient vascular regeneration, which was illustrated by a significant increase in blood flow recovery and reduction in necrosis of muscle tissues [109]. At 25 days post-surgery, all normoxia-cultured MSC-treated mice experienced foot necrosis or toe loss, whereas approximately 90% of the hypoxia-preconditioned MSC-treated group were rescued from ischemic limbs [109]. Recent research compared the pro-angiogenic capacity of exosomes from human adipose-derived MSCs, which were cultured in normoxia (norADSC-Exo) and in hypoxia (hypADSC-Exo). HypADSC-Exo significantly improved the proliferation, migration, and tube-formation ability of HUVECs and supported the survival of the subcutaneous fat grafted in nude mouse models [110].
On the other hand, the second promising strategy for promoting angiogenesis via a hypoxic-induced pathway focuses on utilizing gene therapy to overexpress or stabilize HIF-1 to induce angiogenesis [108]. Researchers have explored a novel action of hydralazine, a clinically used vasodilator, in inducing angiogenesis through in vitro and in vivo examinations. This medicine inhibited HIF degradation by the prolyl hydroxylase domain enzyme, upregulated HIF-targeted genes, and promoted vascular formation in implanted polyether sponges in mouse models [111]. The injection of BM-MSCs transfected with a vector encoding overexpressing mutant HIF-1α in an ovine acute myocardial infarction model was shown to decline infarct size, improve cardiac function, stimulate angiogenesis and arteriogenesis, and perform cardioprotection, with the injected cells remained in sheep for up to 60 days [112].
Culturing on hydrogel matrices and scaffolds
Providing a good environment for MSCs before grafting can have positive effects on cell survival and proliferation. The survival of MSCs in vivo has been shown to be reduced partly due to the lack of matrix materials around the cells [11]. Similar to the culture condition, the adherent surface for MSC culture is known to have positive effects on the cell behavior, which could be used to change gene expression and enhance the cell potential in supporting angiogenesis (Table 1) [119,120,122,127]. For example, when testing the stiffness of gelatin methacrylate-synthesized hydrogel surface on paracrine excretion of MSCs, the expression of VEGF in MSCs culture was shown to be maximized on hydrogel matrix of the concentration of 5% and compression of 5 kPa, which provided the best conditioned media for HUVECs cultured on Matrigel [119]. Surface stiffness was also found to be responsible for the increased expression of VEGF-A. In a study, Rustad and colleagues showed that MSCs cultured on fabricated collagen-pullulan hydrogels significantly expressed higher VEGF than MSCs cultured on plates, which was consequently associated with higher healing potential when administered in animal models [120]. Similarly, the use of rolled collagen scaffolds as an adherent surface was found to enhance VEGF expression of MSCs in vitro and increased wound healing effects with high capillary density when the MSC-containing scaffolds were grafted to wounded diabetic mice [121]. In an animal study, multilayers of MSCs on collagen matrix were shown to be more effective in neovessel formation and brought better outcomes to cardiac infarct area when compared to direct injection of MSCs [123]. These data suggested that the use of scaffolds from different materials and physical forms is promising for enhancing the efficiency of MSCs in application.
The combination of fabricated scaffolds with cytokine has also been implicated in improving MSC vascularisation [122]. Accordingly, VEGF was loaded via incubation onto the porous hydroxyapatite scaffolds coated with multilayers of collagen/heparin, which was then used for MSC culture [122]. The study showed that MSCs grown on such scaffolds were able to induce ECs differentiation in vitro, and the implantation of the scaffolds into rat models enhanced vascularisation with increased vessel formation [122]. The authors suggested that the binding of various growth factors onto the HEP layers and the slow release of VEGF into the environment could be responsible for improving the stable differentiation of MSCs into ECs [122]. However, these conclusions required further validation with experimental data. In fact, several types of scaffolds have been examined for enhancing the potential of MSCs in angiogenesis, which have shown various successes [127]. Together with the given examples, several data have indicated that not only culture conditions and media affect the function of MSCs in angiogenesis, but the way of constructing cells within a culturing scaffold, including 2D and 3D structures and adherent surface (Table 1), can also influence the cell potential in supporting vascularisation.
The action around the effects of adherent surface on MSC secretome has been pointed towards the involvement of oxygen tension and hypoxic condition, which were shown to be correlated with HIF-mediated vascularisation [119–121]. Different materials used in scaffold designs were suggested to work by inducing ECs migration and proliferation, as well as the recruitment of mural cells [127]. At the same time, the adherent response to surface stiffness led to the translocation of surface protein to the nucleus, which stimulated the expression of angiogenic factors [128]. Several signaling pathways were proposed to be responsible for the angiogenic effects induced by surface materials, including ROS-mediated p38-MAPK, phosphatidylinositol 3-kinase/protein kinase B (PI3K/Akt), extracellular regulated protein kinases (ERK) and HIF-1 [127]. However, the precise mechanism remains to be resolved. It must be noted that the transition between the in vitro and in vivo environment of MSCs may provide variation in the cell function. Therefore, understanding the precise mechanism of how culturing scaffolds or surfaces affect MSCs behavior will be important to unravel the key factors that can be targeted for supporting angiogenesis in the in vivo environment.
Application of Mesenchymal Stem Cells in Relation to Angiogenic Regulatory Network
A complex regulatory network of transcription factors and signaling pathways are required to ensure the precise and correct formation of the vascular network [129]. Manipulation of the transcription factor network is often aimed to enhance angiogenesis in different conditions and models, which showed effective vessel generation [130–132]. Therefore, the application of MSCs to support angiogenesis also implied the regulation of angiogenic factors. In this section, we review some of the key regulators that have been shown to increase the healing and angiogenic potential of MSCs in vitro and in vivo (Summarized in Table 2).
P38-Mitogen-Activated Protein Kinase
P38 mitogen-activated protein kinase (P38-MAPK) signaling is a ubiquitous stress response pathway that is involved in multiple cellular activities, including cell survival, proliferation, migration, and other tissue-related processes. Stress and extracellular stimuli trigger a cascade of GTPases upstream of MAPK, resulting in the phosphorylation of p38 and signal transition to regulate cell activity [133]. In stem cell biology, p38-MAPK is also involved in the differentiation and cell fate decisions that are important for cell function [134]. P38-MAPK is activated through the binding of VEGF to VEGFR2 in HUVECs to regulate cell migration, which is important for vessel formation [135]. The inhibition of p38-MAPK was shown to affect capillary sprouting and enhance angiogenesis induced by VEGF in human lung-derived microvascular endothelial cells (HLMECs) in vitro and in vivo [136], while the activation of p38 phosphorylation was shown to lead to EC apoptosis [137]. The inhibition of p38 was also shown to regulate stress response to remodel actin into stress fibers [138]. In addition, when p38 activity was blocked by either reagents or shRNA-p38, chondrogenic differentiation induced by TGF-β1 was suppressed in BM-MSCs [139]. Recently, p38 inhibition has been shown to control MSC commitment to endothelial phenotypes and regulate the angiogenic program in MSCs via the activity of TGF-β1 [140]. Deletion of p38 in MSCs also enhanced tumor formation and angiogenesis when implanted in vivo [140]. Besides, using the p38 inhibitor also promoted MSCs to rejuvenate and acquire the characteristics of hematopoietic stem cells [134]. These data suggested a role of p38 inhibition in MSC differentiation and in angiogenesis, including the recruitment and commitment of ECs. Employing p38 inhibitors and other proteins in the p38-MAPK pathway may provide a way to control angiogenesis when MSCs are used in application. However, the underlying mechanism of p38 in angiogenesis remains a critical question to be resolved.
Notch signaling has been shown to be important for the specification of venous and arterial vessels and the maintenance of tip/stalk cell ratio [9]. Notch protein works in a feedback loop with VEGF to promote the stability of vessel formation by limiting tip-cell phenotypes in ECs and ensuring the correct sprouting process [141]. Accordingly, low-Notch activity in ECs is favorable to tip cell acquisition, while high Notch activity is correlated with stalk cell phenotypes. Notch secreted from MSCs was shown to be important for the formation of new vessels in aortic ECs in vitro and support tube-like formation in the infarcted region of mouse hearts [142]. It was found that Notch activation of Jagged-1 protein is involved in the differentiation of the MSCs into ECs, and the deletion of the Notch gene resulted in the decrease of Jagged-1 protein and reduced the expression of genes in ECs [143]. Thus, targeting Notch protein may be critical to the angiogenic potential of MSCs.
(FGF belongs to the family of heparin-binding factors, which exert their effects through the affinity binding to tyrosine kinase FGF receptors (FGFRs) [144]. More than 20 genes encoding FGFs in humans have been identified as forming either acidic or basic FGFs, and mice deficient for FGFs were either lethal or had multiple defects [145], indicating the essential role of FGFs in development. The binding of FGF/FGFR causes the phosphorylation and activation of downstream signaling molecules to regulate multiple cell activities during angiogenesis, including migration, proliferation, morphogenesis, and vessel formation [144]. Moreover, FGF was also found to involve in variant splicing of VEGFR1 through SR (serine-rich/arginine) proteins (SRSFs) 1, 2 and SR phosphorylating kinases (SRSKs) 1, 2, leading to EC sprouting and neo-vessel outgrowth [146], suggesting that FGF can also regulate other pro-angiogenic factors in angiogenesis. FGFs also work in dual action with PDGFs to increase angiogenesis in vivo [147–149]. Accordingly, transfection of FGF and PDGF plasmids into rat-infarcted heart muscles improved capillary and arteriolar density after 24 h of treatment [148]. The combination of FGF and PDGF proteins was also shown to enhance PDGFR expression and improve corneal neovascularisation, hind-limb collateral growth, and blood perfusion in rat and rabbit models [149]. In MSCs, FGFs are key factors involved in the regulatory function of the cells, including promoting vessel formation [150]. Among soluble FGFs in exosome vesicles secreted by MSCs, basic FGF (bFGF) has been shown to dominantly exert angiogenic potential [151,152]. MSCs transfected with vector constructed with FGF and PDGF genes efficiently promote collateral vessel formation in rat hind-limb ischemic models [153]. Such evidence suggested that as a key regulator in angiogenesis, implying FGFs in therapeutic development either with protein injection or via MSCs transplantation are promising for vessel-related diseases. Particularly, FGFs appeared as the master regulator that can be used efficiently in coordination with other factors to significantly improve angiogenesis, the confirmation of which warrants a thorough examination.
Vascular endothelial growth factor
VEGF is a heterodimeric glycoprotein, a member of the PDGF/VEGF growth factor family, produced by different cell types, including tumor cells, immune cells, platelets, MSCs, and ECs [154–158]. The VEGF family includes VEGF-A, VEGFB, VEGF-C, VEGF-D, placental growth factor, VEGF-E (Orf-VEGF), and Trimeresurus flavoviridis svVEGF. Except for VEGF-E (Orf-VEGF) and T. flavoviridis svVEGF, which respectively originate from parapoxvirus and snake venom, the remaining five members of the VEGF family are encoded by the mammalian genome [159]. Furthermore, after transcription, the mRNA alternative splicing process produces various VEGF isoforms with different biochemical features [160]. In the VEGF molecular structure, there are eight conserved cysteine residues, including six residues, forming three intramolecular bisulfidic bonds to make three loop structures, and the other two cysteines contributing to the construction of homodimer by forming two intermolecular bisulfidic bonds [161].
The VEGF receptor (VEGFR) is a kind of tyrosine kinase receptor, which has an extracellular domain for ligand binding, a transmembrane domain, and a cytoplasmic domain that performs tyrosine kinase activity [162]. There are three main types of VEGFR, namely VEGFR-1 (Flt-1), VEGFR2 (Flk-1 or KDR), and VEGFR-3 (Flt-4). Notably, VEGFR-1 has a soluble form, called sFlt-1. Different VEGF-VEGFR complexes have a variety of functions. For example, the interaction between VEGF-A and VEGFR-1/VEGFR-2 forms a crucial regulator in both normal and pathological angiogenesis, while VEGF-C and VEGF-D with their receptor VEGFR-3 mainly participate in lymphangiogenesis [159].
VEGF/VEGFR system can influence multiple steps in angiogenesis. First, VEGF promotes the differentiation of endothelial progenitor cells into ECs [163]. During sprouting angiogenesis, VEGF-A with its receptor VEGF-2 plays a key role in selecting tip cells, and works as chemokines that direct their migration [164]. VEGF regulates EC proliferation and survival, and prevents apoptosis. Also, VEGF is an inducible signal for tubulogenesis, the process of the formation of vascular tube [165]. In fact, due to their central role in vessel growth regulation, it has been shown that abnormalities in VEGF-VEGFR levels may cause serious disorders. In the research using mouse embryos, lacking a single VEGF allele led to abnormal blood vessel development and even embryonic death at early stages [166]. The role of VEGF signaling in vascularization was demonstrated using mutant mouse embryos, which expressed two- to three-fold VEGF levels. The result showed that the VEGF overexpression caused cardiac impairment and lethality in 12.5- to 14-day embryos [167]. While the gradient of VEGF affects the migration of tip cells, the concentration of VEGF is important for the proliferation of stalk cells [168], most probably due to the fact that VEGF works in response to NOTCH to maintain the correct selection of tip- and stalk cells to prevent excessive vessel formation [141]. Furthermore, VEGF, when secreted and performing action inside ECs, can produce a survival factor to prevent ECs from apoptosis under general conditions [169], which is important for the stability of the functioning vessels. VEGF secreted by MSCs has been shown to be involved in the modeling of blood vessels of pancreatic tumors both in vitro and in vivo experiments, indicating the role of MSCs as an efficient secretor of the angiogenic pool [12].
Many studies have shown that activation of the VEGF-VEGFR signaling pathway could stimulate vascular regeneration in conditions such as myocardial infarction, cardiovascular ischemia, and hind-limb ischemia [170]. The combination of VEGF with biomaterials as a VEGF-releasing system was shown to improve neovascularization in tissue engineering. Two weeks after implantation into mouse models, the surface of the human recombinant VEGF-incorporated scaffold was completely covered by new blood vessels, which was confirmed by confocal microscopy and immunohistochemistry analysis [171]. In terms of cancer therapy, the inhibitors of VEGF-dependent angiogenesis, particularly in tumors, have emerged as prospective anti-cancer agents. For example, in 2006, the FDA approved Bevacizumab (Avastin®), a recombinant humanized monoclonal antibody against VEGF, in combination with Carboplatin and Paclitaxel as a treatment for patients with unresectable, locally advanced, recurrent, or metastatic, nonsquamous and non-small cell lung cancer [172]. Additionally, the anti-cancer effect of vialinin A, a natural compound in mushrooms, was suggested after researchers explored that it prevented VEGF-induced proliferation, migration, and tube formation of HUVECs in vitro and suppressed new blood vessel formation in a Matrigel plug assay in mice [173]. Eriocalyxin B, isolated from the plant Isodon eriocalyx var. laxiflora, also inhibits VEGFR-2 signaling, thus reducing tumor vascularization and growth in the 4T1 breast tumor model [174]. These data indicated that VEGF is the key angiogenic factor that can be employed in combination with MSCs to enhance vessel formation. However, the level of VEGF-induced angiogenesis should be thoroughly examined to prevent excessive vascularisation.
Platelet-derived growth factor/PDGF ligands and their receptors (PDGF/PDGFR)
PDGF signaling is formed by the binding between PDGFR, which works via the phosphorylation of tyrosine residues on the activated molecules, sending signals to mediate cell response. Several signal transduction molecules are involved in PDGF activation, including PI3K, AKT, MAP, Src, phospholipase C-υ (PLC-υ), and Ras GTPase-activating protein (Ras-GAP) [175,176]. PDGF/PDGFR interaction forms different types of homodimers and heterodimers, which mediate cell and tissue activities, including migration and differentiation, and vasculogenesis and organogenesis [175]. The recruitment of pericytes to the site of sprouting is required for the maturation of new vessels and is regulated by the duet action of PDGF and its receptor PDGFR, especially PDGF-B/PDGFR-β [177–179]. PDGF is secreted by tip cells to the neighboring area to stop ECs from becoming tip cells and recruit pericytes to stabilize the structure of the new vessels [180]. Any disruption in PDGF/PDGFR expression has been shown to result in the malformation of blood vessels, and the knockout of PDGFR and PDGF-B in mice was shown to be lethal at the early stage of development [181].
Glicoma-associated MSCs treated with PDGF-BB showed significantly increased tube formation on Matrigel; however, depending on the concentration of PDGF in the treatment, tube stabilization was shown to be affected [182]. In vivo, the inhibition of PDGF-BB was found to reduce vessel formation by promoting EC migration, proliferation, and formation [176]. Treatment of PDGF-BB inhibitor also decreased the expression of pro-angiogenic factors such as VEGF-A, MMP-2, MMP-9, and increased the expression of anti-angiogenic molecules, such as TSP-1, TSP-2, ADAMTS-1, and ADAMTS-2 in mouse corneas [176]. Condition medium from chronic lymphocytic leukemic cells (CLL) were shown to contain various levels of PDGF, which activated PDGFR phosphorylation in MSC culture and promoted MSCs to produce VEGF via PK3K pathway [183]. The subpopulation of MSCs with high expression of PDGF-β was shown to express high levels of pro-angiogenic factors such as VEGF, PDGFA, PDGFB, and bFGF, and enhanced angiogenic ability [184]. These data suggest the important roles of the PDGF factor and PDGF/PDGFR signaling in vessel formation. The implication of the PDGF/PDGFR pathway in constructing MSCs with thorough examination is promising for future application in vascular-related diseases.
In this review, we have discussed the potential use of MSCs to effectively promote and support vasculogenesis and angiogenesis via various regulatory effects, including alleviation of cell apoptosis, enhancing pro-angiogenic factors, and improving EC migration and proliferation, thus offering opportunities for clinical application. We also point out that pre-culture and pre-treatment of MSCs are promising to increase survival, proliferation rate, and precise homeostasis, and enhance angiogenic potential. However, from what has been discussed, it is suspected that a single method to either pre-treat MSCs or select MSC subpopulations pre-transplantation might be met with low angiogenic efficacy in vivo. Thus, the combination of different strategies mentioned in this review may bring better consequences.
For instance, the integration between MSC subpopulations, growing platforms, and transcription factors may result in better outcomes in vascular diseases. Since angiogenic sprouting and neovascular formation are comprised of multiple processes, solutions for future cell therapy may require either multiple steps of treatments or a combination of multiple cell types and technologies, which are warranted for future research. In other words, a matrix combination of MSC subpopulation selection, with key angiogenic regulators on specific scaffolds may be worth a go for future intervention direction. Perhaps a serial treatment method, which targets each of the vessel formation and maturation processes, may be valuable in future applications.
Acknowledgement: None.
Funding Statement: The authors received no specific funding for this study.
Author Contributions: VTTN and PVP suggested the ideas for the manuscript, VTTN, KDP, HTQC drafted the manuscript. VTTN and PDP drafted the figures. PVP corrected the manuscript, revised the figures. Authors significantly contributed to this work. All authors read and approved the final manuscript.
Availability of Data and Materials: All data generated or analyzed during this study are included in this published article.
Ethics Approval: Not applicable.
Conflicts of Interest: The authors declare that they have no conflicts of interest to report regarding the present study.
References
1. Kasper G, Dankert N, Tuischer J, Hoeft M, Gaber T, Glaeser JD, et al. Mesenchymal stem cells regulate angiogenesis according to their mechanical environment. Stem Cells. 2007;25(4):903–10. [Google Scholar] [PubMed]
2. Edwards SS, Zavala G, Prieto CP, Elliott M, Martinez S, Egana JT, et al. Functional analysis reveals angiogenic potential of human mesenchymal stem cells from Wharton’s jelly in dermal regeneration. Angiogenesis. 2014;17(4):851–66. [Google Scholar] [PubMed]
3. Katagiri W, Kawai T, Osugi M, Sugimura-Wakayama Y, Sakaguchi K, Kojima T, et al. Angiogenesis in newly regenerated bone by secretomes of human mesenchymal stem cells. Maxillofac Plast Reconstr Surg. 2017;39(1):8. [Google Scholar] [PubMed]
4. Wu SZ, Li YL, Huang W, Cai WF, Liang J, Paul C, et al. Paracrine effect of CXCR4-overexpressing mesenchymal stem cells on ischemic heart injury. Cell Biochem Funct. 2017;35(2):113–23. [Google Scholar] [PubMed]
5. Zhang L, Zhou Y, Sun X, Zhou J, Yang P. CXCL12 overexpression promotes the angiogenesis potential of periodontal ligament stem cells. Sci Rep. 2017;7(1):10286. [Google Scholar] [PubMed]
6. Serra J, Alves CPA, Brito L, Monteiro GA, Cabral JMS, Prazeres DMF, et al. Engineering of human mesenchymal stem/stromal cells with vascular endothelial growth factor-encoding minicircles for angiogenic ex vivo gene therapy. Hum Gene Ther. 2019;30(3):316–29. [Google Scholar] [PubMed]
7. Li Z, Yang A, Yin X, Dong S, Luo F, Dou C, et al. Mesenchymal stem cells promote endothelial progenitor cell migration, vascularization, and bone repair in tissue-engineered constructs via activating CXCR2-Src-PKL/Vav2-Rac1. FASEB J. 2018;32(4):2197–211. [Google Scholar] [PubMed]
8. van Nguyen TT, Vu NB, van Pham P. Mesenchymal stem cell transplantation for ischemic diseases: mechanisms and challenges. Tissue Eng Regen Med. 2021;18(4):587–611. [Google Scholar] [PubMed]
9. Kolte D, McClung JA, Aronow WS. Chapter 6-vasculogenesis and angiogenesis. In: Aronow WS, McClung JA (eds.) Translational Research in Coronary Artery Disease. Boston: Academic Press; 2016. p. 49–65. [Google Scholar]
10. Chen T, Ye B, Tan J, Yang H, He F, Khalil RA. CD146+Mesenchymal stem cells treatment improves vascularization, muscle contraction and VEGF expression, and reduces apoptosis in rat ischemic hind limb. Biochem Pharmacol. 2021;190:114530. [Google Scholar] [PubMed]
11. Daadi MM, Li Z, Arac A, Grueter BA, Sofilos M, Malenka RC, et al. Molecular and magnetic resonance imaging of human embryonic stem cell-derived neural stem cell grafts in ischemic rat brain. Mol Ther. 2009;17(7):1282–91. [Google Scholar] [PubMed]
12. Beckermann BM, Kallifatidis G, Groth A, Frommhold D, Apel A, Mattern J, et al. VEGF expression by mesenchymal stem cells contributes to angiogenesis in pancreatic carcinoma. Br J Cancer. 2008;99(4):622–31. [Google Scholar]
13. Adair TH, Montani JP. Angiogenesis. California: Morgan & Claypool Life Sciences; 2010. [Google Scholar]
14. Bautch VL, Caron KM. Blood and lymphatic vessel formation. Cold Spring Harb Perspect Biol. 2015;7(3):a008268. [Google Scholar] [PubMed]
15. Dejana E, Tournier-Lasserve E, Weinstein BM. The control of vascular integrity by endothelial cell junctions: molecular basis and pathological implications. Dev Cell. 2009;16(2):209–21. [Google Scholar] [PubMed]
16. Michiels C. Endothelial cell functions. J Cell Physiol. 2003;196(3):430–43. [Google Scholar]
17. Bazzoni G, Dejana E. Endothelial cell-to-cell junctions: molecular organization and role in vascular homeostasis. Physiol Rev. 2004;84(3):869–901. [Google Scholar] [PubMed]
18. Cerutti C, Ridley AJ. Endothelial cell-cell adhesion and signaling. Exp Cell Res. 2017;358(1):31–8. [Google Scholar] [PubMed]
19. Figueroa XF, Duling BR. Gap junctions in the control of vascular function. Antioxid Redox Signal. 2009;11(2):251–66. [Google Scholar] [PubMed]
20. Potente M, Gerhardt H, Carmeliet P. Basic and therapeutic aspects of angiogenesis. Cell. 2011;146(6):873–87. [Google Scholar] [PubMed]
21. Wittchen ES. Endothelial signaling in paracellular and transcellular leukocyte transmigration. Front Biosci. 2009;14(14):2522–45. [Google Scholar]
22. Villar I, Francis S, Webb A, Hobbs A, Ahluwalia A. Novel aspects of endothelium-dependent regulation of vascular tone. Kidney Int. 2006;70(5):840–53. [Google Scholar] [PubMed]
23. Sandoo A, van Zanten JJV, Metsios GS, Carroll D, Kitas GD. The endothelium and its role in regulating vascular tone. Open Cardiovasc Med J. 2010;4(1):302–12. [Google Scholar]
24. Barton M, Baretella O, Meyer MR. Obesity and risk of vascular disease: importance of endothelium-dependent vasoconstriction. Br J Pharmacol. 2012;165(3):591–602. [Google Scholar] [PubMed]
25. Daniel AE, Van Buul JD. Endothelial junction regulation: a prerequisite for leukocytes crossing the vessel wall. J Innate Immun. 2013;5(4):324–35. [Google Scholar] [PubMed]
26. Cho HH, Kim YJ, Kim JT, Song JS, Shin KK, Bae YC, et al. The role of chemokines in proangiogenic action induced by human adipose tissue-derived mesenchymal stem cells in the murine model of hindlimb ischemia. C Cell Physiol Biochem. 2009;24(5–6):511–8. [Google Scholar]
27. Irvin MW, Zijlstra A, Wikswo JP, Pozzi A. Techniques and assays for the study of angiogenesis. Exp Biol Med. 2014;239(11):1476–88. [Google Scholar]
28. Betz C, Lenard A, Belting HG, Affolter M. Cell behaviors and dynamics during angiogenesis. Development. 2016;143(13):2249–60. [Google Scholar] [PubMed]
29. Nitzsche F, Muller C, Lukomska B, Jolkkonen J, Deten A, Boltze J. Concise review: MSC adhesion cascade-insights into homing and transendothelial migration. Stem Cells. 2017;35(6):1446–60. [Google Scholar] [PubMed]
30. Pepper MS, Ferrara N, Orci L, Montesano R. Vascular endothelial growth factor (VEGF) induces plasminogen activators and plasminogen activator inhibitor-1 in microvascular endothelial cells. Biochem Biophys Res Commun. 1991;181(2):902–6. [Google Scholar] [PubMed]
31. Pepper MS, Mandriota SJ, Jeltsch M, Kumar V, Alitalo K. Vascular endothelial growth factor (VEGF)-C synergizes with basic fibroblast growth factor and VEGF in the induction of angiogenesis in vitro and alters endothelial cell extracellular proteolytic activity. J Cell Physiol. 1998;177(3):439–52. [Google Scholar] [PubMed]
32. Eliceiri BP, Cheresh DA. The role of alphav integrins during angiogenesis. Mol Med. 1998;4(12):741–50. [Google Scholar]
33. Brooks PC, Montgomery AM, Rosenfeld M, Reisfeld RA, Hu T, Klier G, et al. Integrin αvβ3 antagonists promote tumor regression by inducing apoptosis of angiogenic blood vessels. Cell. 1994;79(7):1157–64. [Google Scholar] [PubMed]
34. Gluzman Z, Koren B, Preis M, Cohen T, Tsaba A, Cosset FL, et al. Endothelial cells are activated by angiopoeitin-1 gene transfer and produce coordinated sprouting in vitro and arteriogenesis in vivo. Biochem Biophys Res Commun. 2007;359(2):263–836. [Google Scholar] [PubMed]
35. Suri C, Jones PF, Patan S, Bartunkova S, Maisonpierre PC, Davis S, et al. Requisite role of angiopoietin-1, a ligand for the TIE2 receptor, during embryonic angiogenesis. Cell. 1996;87(7):1171–80. [Google Scholar] [PubMed]
36. Hellberg C, Ostman A, Heldin CH. PDGF and vessel maturation. Recent Results Cancer Res. 2010;180:103–14. [Google Scholar] [PubMed]
37. Klagsbrun M, Moses MA. Molecular angiogenesis. Chem & Biol. 1999;6(8):R217–24. [Google Scholar]
38. Ullah I, Subbarao RB, Rho GJ. Human mesenchymal stem cells-current trends and future prospective. Biosci Rep. 2015;35(2):e00191. [Google Scholar] [PubMed]
39. Dominici M, Le Blanc K, Mueller I, Slaper-Cortenbach I, Marini F, Krause D, et al. Minimal criteria for defining multipotent mesenchymal stromal cells. The international society for cellular therapy position statement. Cytotherapy. 2006;8(4):315–7. [Google Scholar] [PubMed]
40. Wilson A, Hodgson-Garms M, Frith JE, Genever P. Multiplicity of mesenchymal stromal cells: finding the right route to therapy. Front Immunol. 2019;10:1112. [Google Scholar] [PubMed]
41. Huang Y, Li Q, Zhang K, Hu M, Wang Y, Du L, et al. Single cell transcriptomic analysis of human mesenchymal stem cells reveals limited heterogeneity. Clin Transl Med. 2019;10(5):368. [Google Scholar]
42. Liu X, Rui T, Zhang S, Ding Z. Heterogeneity of MSC: origin, molecular identities, and functionality. Stem Cells Int. 2019;2019:9281520. [Google Scholar]
43. Uccelli A, Moretta L, Pistoia V. Mesenchymal stem cells in health and disease. Nat Rev Immunol. 2008;8(9):726–36. [Google Scholar] [PubMed]
44. M.Kaplan J, E.Youd M, A.Lodie T. Immunomodulatory activity of mesenchymal stem cells. Curr Stem Cell Res Ther. 2011;6(4):297–316. [Google Scholar]
45. Gnecchi M, Danieli P, Malpasso G, Ciuffreda MC. Paracrine mechanisms of mesenchymal stem cells in tissue repair. Methods Mol Biol. 2016;2016:123–46. [Google Scholar]
46. Takeuchi R, Katagiri W, Endo S, Kobayashi T. Exosomes from conditioned media of bone marrow-derived mesenchymal stem cells promote bone regeneration by enhancing angiogenesis. PLoS One. 2019;14(11):e0225472. [Google Scholar] [PubMed]
47. Zavala G, Prieto CP, Villanueva AA, Palma V. Sonic hedgehog (SHH) signaling improves the angiogenic potential of Wharton’s jelly-derived mesenchymal stem cells (WJ-MSC). Stem Cell Res Ther. 2017;8(1):1–17. [Google Scholar]
48. Prieto CP, Ortiz MC, Villanueva A, Villarroel C, Edwards SS, Elliott M, et al. Netrin-1 acts as a non-canonical angiogenic factor produced by human Wharton’s jelly mesenchymal stem cells (WJ-MSC). Stem Cell Res Ther. 2017;8(1):1–15. [Google Scholar]
49. Zhang K, Zhao X, Chen X, Wei Y, Du W, Wang Y, et al. Enhanced therapeutic effects of mesenchymal stem cell-derived exosomes with an injectable hydrogel for hindlimb ischemia treatment. ACS Appl Mater Interfaces. 2018;10(36):30081–91. [Google Scholar] [PubMed]
50. Robinson ST, Douglas AM, Chadid T, Kuo K, Rajabalan A, Li H, et al. A novel platelet lysate hydrogel for endothelial cell and mesenchymal stem cell-directed neovascularization. Acta Biomaterialia. 2016;36(10):86–98. [Google Scholar] [PubMed]
51. Xiang Q, Hong D, Liao Y, Cao Y, Liu M, Pang J, et al. Overexpression of gremlin1 in mesenchymal stem cells improves hindlimb ischemia in mice by enhancing cell survival. J Cell Physiol. 2017;232(5):996–1007. [Google Scholar] [PubMed]
52. Xin H, Li Y, Cui Y, Yang JJ, Zhang ZG, Chopp M. Systemic administration of exosomes released from mesenchymal stromal cells promote functional recovery and neurovascular plasticity after stroke in rats. J Cereb Blood Flow Metab. 2013;33(11):1711–5. [Google Scholar] [PubMed]
53. Park S-R. Exosomes derived from mesenchymal stem cells suppress angiogenesis by down-regulating VEGF expression in breast cancer cells. Cancer Res. 2014;74(19_Supplement):170. [Google Scholar]
54. Wang E. Understanding genomic alterations in cancer genomes using an integrative network approach. Cancer Lett. 2013;340(2):261–9. [Google Scholar]
55. Kebir A, Harhouri K, Guillet B, Liu JW, Foucault-Bertaud A, Lamy E, et al. CD146 short isoform increases the proangiogenic potential of endothelial progenitor cells in vitro and in vivo. Circ Res. 2010;107(1):66–75. [Google Scholar] [PubMed]
56. Kang Y, Wang F, Feng J, Yang D, Yang X, Yan X. Knockdown of CD146 reduces the migration and proliferation of human endothelial cells. Cell Res. 2006;16(3):313–8. [Google Scholar]
57. Luo Y, Teng X, Zhang L, Chen J, Liu Z, Chen X, et al. Publisher correction: CD146-HIF-1α hypoxic reprogramming drives vascular remodeling and pulmonary arterial hypertension. Nat Commun. 2019;10(1):4098. [Google Scholar] [PubMed]
58. Essaadi A, Nollet M, Moyon A, Stalin J, Simoncini S, Balasse L, et al. Stem cell properties of peripheral blood endothelial progenitors are stimulated by soluble CD146 via miR-21: potential use in autologous cell therapy. Sci Rep. 2018;8(1):9387. [Google Scholar]
59. Harhouri K, Kebir A, Guillet B, Foucault-Bertaud A, Voytenko S, Piercecchi-Marti MD, et al. Soluble CD146 displays angiogenic properties and promotes neovascularization in experimental hind-limb ischemia. Blood. 2010;115(18):3843–51. [Google Scholar]
60. Stalin J, Harhouri K, Hubert L, Garrigue P, Nollet M, Essaadi A, et al. Soluble CD146 boosts therapeutic effect of endothelial progenitors through proteolytic processing of short CD146 isoform. Cardiovasc Res. 2016;111(3):240–51. [Google Scholar]
61. Chirieleison SM, Feduska JM, Schugar RC, Askew Y, Deasy BM. Human muscle-derived cell populations isolated by differential adhesion rates: phenotype and contribution to skeletal muscle regeneration in Mdx/SCID mice. Tissue Eng Part A. 2012;18(3–4):232–41. [Google Scholar]
62. Lv FJ, Tuan RS, Cheung KM, Leung VY. Concise review: the surface markers and identity of human mesenchymal stem cells. Stem Cells. 2014;32(6):1408–19. [Google Scholar] [PubMed]
63. Harkness L, Zaher W, Ditzel N, Isa A, Kassem M. CD146/MCAM defines functionality of human bone marrow stromal stem cell populations. Stem Cell Res Ther. 2016;7(1):1–13. [Google Scholar]
64. Aicher WK, Bühring HJ, Hart M, Rolauffs B, Badke A, Klein G. Regeneration of cartilage and bone by defined subsets of mesenchymal stromal cells—potential and pitfalls. Adv Drug Deliv Rev. 2011;63(4–5):342–51. doi:10.1016/j.addr.2010.12.004. [Google Scholar] [CrossRef]
65. Joshkon A, Heim X, Dubrou C, Bachelier R, Traboulsi W, Stalin J, et al. Role of CD146 (MCAM) in physiological and pathological angiogenesis—contribution of new antibodies for therapy. Biomedicines. 2020;8(12):633. [Google Scholar]
66. Tachibana K, Hirota S, Iizasa H, Yoshida H, Kawabata K, Kataoka Y, et al. The chemokine receptor CXCR4 is essential for vascularization of the gastrointestinal tract. Nature. 1998;393(6685):591–4. [Google Scholar] [PubMed]
67. Wynn RF, Hart CA, Corradi-Perini C, O’Neill L, Evans CA, Wraith JE, et al. A small proportion of mesenchymal stem cells strongly expresses functionally active CXCR4 receptor capable of promoting migration to bone marrow. Blood. 2004;104(9):2643–5. [Google Scholar]
68. Petit I, Jin D, Rafii S. The SDF-1-CXCR4 signaling pathway: a molecular hub modulating neo-angiogenesis. Trends Immunol. 2007;28(7):299–307. [Google Scholar]
69. Unoki N, Murakami T, Nishijima K, Ogino K, van Rooijen N, Yoshimura N. SDF-1/CXCR4 contributes to the activation of tip cells and microglia in retinal angiogenesis. Invest Ophthalmol Vis Sci. 2010;51(7):3362–71. [Google Scholar] [PubMed]
70. Yu Q, Liu L, Lin J, Wang Y, Xuan X, Guo Y, et al. SDF-1α/CXCR4 axis mediates the migration of mesenchymal stem cells to the hypoxic-ischemic brain lesion in a rat model. Cell J. 2015;16(4):440–7. [Google Scholar] [PubMed]
71. Liu H, Liu S, Li Y, Wang X, Xue W, Ge G, et al. The role of SDF-1-CXCR4/CXCR7 axis in the therapeutic effects of hypoxia-preconditioned mesenchymal stem cells for renal ischemia/reperfusion injury. PLoS One. 2012;7(4):e34608. [Google Scholar] [PubMed]
72. Liu H, Xue W, Ge G, Luo X, Li Y, Xiang H, et al. Hypoxic preconditioning advances CXCR4 and CXCR7 expression by activating HIF-1α in MSCs. Biochem Biophys Res Commun. 2010;401(4):509–15. [Google Scholar]
73. Ryu CH, Park SA, Kim SM, Lim JY, Jeong CH, Jun JA, et al. Migration of human umbilical cord blood mesenchymal stem cells mediated by stromal cell-derived factor-1/CXCR4 axis via Akt, ERK, and p38 signal transduction pathways. Biochem Biophys Res Commun. 2010;398(1):105–10. [Google Scholar] [PubMed]
74. Li N, Yang YJ, Qian HY, Li Q, Zhang Q, Li XD, et al. Intravenous administration of atorvastatin-pretreated mesenchymal stem cells improves cardiac performance after acute myocardial infarction: role of CXCR4. Am J Transl Res. 2015;7(6):1058–70. [Google Scholar] [PubMed]
75. Walter DH, Haendeler J, Reinhold J, Rochwalsky U, Seeger F, Honold J, et al. Impaired CXCR4 signaling contributes to the reduced neovascularization capacity of endothelial progenitor cells from patients with coronary artery disease. Circulation Res. 2005;97(11):1142–51. [Google Scholar] [PubMed]
76. Misao Y, Takemura G, Arai M, Ohno T, Onogi H, Takahashi T, et al. Importance of recruitment of bone marrow-derived CXCR4+ cells in post-infarct cardiac repair mediated by G-CSF. Cardiovasc Res. 2006;71(3):455–65. [Google Scholar] [PubMed]
77. Cheng Z, Ou L, Zhou X, Li F, Jia X, Zhang Y, et al. Targeted migration of mesenchymal stem cells modified with CXCR4 gene to infarcted myocardium improves cardiac performance. Mol Ther. 2008;16(3):571–9. [Google Scholar] [PubMed]
78. Won YW, Patel AN, Bull DA. Cell surface engineering to enhance mesenchymal stem cell migration toward an SDF-1 gradient. Biomaterials. 2014;35(21):5627–35. [Google Scholar] [PubMed]
79. Chao CC, Lee CW, Chang TM, Chen PC, Liu JF. CXCL1/CXCR2 paracrine axis contributes to lung metastasis in osteosarcoma. Cancers. 2020;12(2):459. [Google Scholar] [PubMed]
80. Ma Y, Xia Z, Ye C, Lu C, Zhou S, Pan J, et al. AGTR1 promotes lymph node metastasis in breast cancer by upregulating CXCR4/SDF-1α and inducing cell migration and invasion. Aging. 2019;11(12):3969–92. [Google Scholar] [PubMed]
81. Janowski M. Functional diversity of SDF-1 splicing variants. Cell Adh Migr. 2009;3(3):243–9. [Google Scholar] [PubMed]
82. Tang J, Wang J, Guo L, Kong X, Yang J, Zheng F, et al. Mesenchymal stem cells modified with stromal cell-derived factor 1α improve cardiac remodeling via paracrine activation of hepatocyte growth factor in a rat model of myocardial infarction. Mol Cells. 2010;29(1):9–19. [Google Scholar] [PubMed]
83. Gong XH, Liu H, Wang SJ, Liang SW, Wang GG. Exosomes derived from SDF1-overexpressing mesenchymal stem cells inhibit ischemic myocardial cell apoptosis and promote cardiac endothelial microvascular regeneration in mice with myocardial infarction. J Cell Physiol. 2019;234(8):13878–93. [Google Scholar] [PubMed]
84. Yu Q, Liu L, Lin J, Wang Y, Xuan X, Guo Y, et al. SDF-1α/CXCR4 axis mediates the migration of mesenchymal stem cells to the hypoxic-ischemic brain lesion in a rat model. Cell J. 2015;16(4):440–7. [Google Scholar]
85. Jin W, Liang X, Brooks A, Futrega K, Liu X, Doran MR, et al. Modelling of the SDF-1/CXCR4 regulated in vivo homing of therapeutic mesenchymal stem/stromal cells in mice. PeerJ. 2018;6(26):e6072. [Google Scholar] [PubMed]
86. Liu X, Duan B, Cheng Z, Jia X, Mao L, Fu H, et al. SDF-1/CXCR4 axis modulates bone marrow mesenchymal stem cell apoptosis, migration and cytokine secretion. Protein Cell. 2011;2(10):845–54. [Google Scholar] [PubMed]
87. Matluobi D, Araghi A, Maragheh BFA, Rezabakhsh A, Soltani S, Khaksar M, et al. Carvacrol promotes angiogenic paracrine potential and endothelial differentiation of human mesenchymal stem cells at low concentrations. Microvasc Res. 2018;115(1):20–7. [Google Scholar]
88. Lee J, Shin MS, Kim MO, Jang S, Oh SW, Kang M, et al. Apple ethanol extract promotes proliferation of human adult stem cells, which involves the regenerative potential of stem cells. Nutr Res. 2016;36(9):925–36. [Google Scholar]
89. Saxton RA, Sabatini DM. mTOR signaling in growth, metabolism, and disease. Cell. 2017;168(6):960–76. [Google Scholar] [PubMed]
90. Deschepper M, Manassero M, Oudina K, Paquet J, Monfoulet LE, Bensidhoum M, et al. Proangiogenic and prosurvival functions of glucose in human mesenchymal stem cells upon transplantation. Stem Cells. 2013;31(3):526–35. [Google Scholar] [PubMed]
91. Mihai MC, Popa MA, Suica VI, Antohe F, Jackson EK, Simionescu M, et al. Mechanism of 17β-estradiol stimulated integration of human mesenchymal stem cells in heart tissue. J Mol Cell Cardiol. 2019;133:115–24. [Google Scholar] [PubMed]
92. Popa MA, Mihai MC, Constantin A, Şuică V, Ţucureanu C, Costache R, et al. Dihydrotestosterone induces pro-angiogenic factors and assists homing of MSC into the cardiac tissue. J Mol Endocrinol. 2018;60(1):1–15. [Google Scholar] [PubMed]
93. Ma Q, Xia X, Tao Q, Lu K, Shen J, Xu Q, et al. Profound actions of an agonist of growth hormone-releasing hormone on angiogenic therapy by mesenchymal stem cells. Arterioscler Thromb Vasc Biol. 2016;36(4):663–72. [Google Scholar] [PubMed]
94. Chang K, Lubo Z. Review article: steroid hormones and uterine vascular adaptation to pregnancy. Reprod Sci. 2008;15(4):336–48. [Google Scholar]
95. Maliqueo M, Echiburú B, Crisosto N, Echiburú, Crisosto N. Sex steroids modulate uterine-placental vasculature: implications for obstetrics and neonatal outcomes. Front Physiol. 2016;7:152. [Google Scholar]
96. Losordo DW, Isner JM. Estrogen and angiogenesis: a review. Arterioscler Thromb Vasc Biol. 2001;21(1):6–12. [Google Scholar]
97. Clapp C, Thebault S, Jeziorski MC, Martínez de la Escalera G. Peptide hormone regulation of angiogenesis. Physiol Rev. 2009;89(4):1177–215. [Google Scholar] [PubMed]
98. Woodward WA, Wachsberger P, Burd R, Dicker AP. Effects of androgen suppression and radiation on prostate cancer suggest a role for angiogenesis blockade. Prostate Cancer Prostatic Dis. 2005;8(2):127–32. [Google Scholar] [PubMed]
99. Yager JD. Mechanisms of estrogen carcinogenesis: the role of E2/E1-quinone metabolites suggests new approaches to preventive intervention—a review. Steroids. 2015;99:56–60. [Google Scholar]
100. Redza-Dutordoir M, Averill-Bates DA. Activation of apoptosis signalling pathways by reactive oxygen species. Biochimica et Biophysica Acta (BBA)-Molecular Cell Res. 2016;1863(12):2977–92. [Google Scholar]
101. Tamamouna V, Pitsouli C. The hypoxia-inducible factor-1α in angiogenesis and cancer: insights from the drosophila model. In: Gene expression and regulation in mammalian cells: transcription toward the establishment of novel therapeutics. London, UK Publisher: IntechOpen; 2018. p. 209. [Google Scholar]
102. Ziello JE, Jovin IS, Huang Y. Hypoxia-inducible factor (HIF)-1 regulatory pathway and its potential for therapeutic intervention in malignancy and ischemia. Yale J Biol Med. 2007;80(2):51–60. [Google Scholar] [PubMed]
103. Diaz-Gonzalez JA, Russell J, Rouzaut A, Gil-Bazo I, Montuenga L. Targeting hypoxia and angiogenesis through HIF-1α inhibition. Cancer Biol Ther. 2005;4(10):1055–62. [Google Scholar] [PubMed]
104. Chowdhury R, Leung IK, Tian Y-M, Abboud MI, Ge W, Domene C, et al. Structural basis for oxygen degradation domain selectivity of the HIF prolyl hydroxylases. Nature Commun. 2016;7(1):1–10. [Google Scholar]
105. Yoshida D, Kim K, Noha M, Teramoto A. Hypoxia inducible factor 1-α regulates of platelet derived growth factor-B in human glioblastoma cells. J Neurooncol. 2006;76(1):13–21. [Google Scholar] [PubMed]
106. Manalo DJ, Rowan A, Lavoie T, Natarajan L, Kelly BD, Ye SQ, et al. Transcriptional regulation of vascular endothelial cell responses to hypoxia by HIF-1. Blood. 2005;105(2):659–69. [Google Scholar] [PubMed]
107. Tang N, Wang L, Esko J, Giordano FJ, Huang Y, Gerber HP, et al. Loss of HIF-1α in endothelial cells disrupts a hypoxia-driven VEGF autocrine loop necessary for tumorigenesis. Cancer Cell. 2004;6(5):485–95. [Google Scholar] [PubMed]
108. Hadjipanayi E, Schilling AF. Hypoxia-based strategies for angiogenic induction: the dawn of a new era for ischemia therapy and tissue regeneration. Organogenesis. 2013;9(4):261–72. [Google Scholar] [PubMed]
109. Han YS, Lee JH, Yoon YM, Yun CW, Noh H, Lee SH. Hypoxia-induced expression of cellular prion protein improves the therapeutic potential of mesenchymal stem cells. Cell Death Dis. 2016;7(10):e2395. [Google Scholar] [PubMed]
110. Han Y, Ren J, Bai Y, Pei X, Han Y. Exosomes from hypoxia-treated human adipose-derived mesenchymal stem cells enhance angiogenesis through VEGF/VEGF-R. Int J Biochem Cell Biol. 2019;109(1):59–68. [Google Scholar] [PubMed]
111. Knowles HJ, Tian YM, Mole DR, Harris AL. Novel mechanism of action for hydralazine: induction of hypoxia-inducible factor-1 α, vascular endothelial growth factor, and angiogenesis by inhibition of prolyl hydroxylases. Circ Res. 2004;95(2):162–9. [Google Scholar] [PubMed]
112. Hnatiuk AP, Ong SG, Olea FD, Locatelli P, Riegler J, Lee WH, et al. Allogeneic mesenchymal stromal cells overexpressing mutant human hypoxia-inducible factor 1-α (HIF 1-α) in an ovine model of acute myocardial infarction. J Am Heart Assoc. 2016;5(7):e003714. [Google Scholar] [PubMed]
113. Pourjafar M, Saidijam M, Mansouri K, Ghasemibasir H, Karimi Dermani F, Najafi R. All-trans retinoic acid preconditioning enhances proliferation, angiogenesis and migration of mesenchymal stem cell in vitro and enhances wound repair in vivo. Cell Prolif. 2017;50(1):e12315. [Google Scholar] [PubMed]
114. Sun X, Fang B, Zhao X, Zhang G, Ma H. Preconditioning of mesenchymal stem cells by sevoflurane to improve their therapeutic potential. PLoS One. 2014;9(3):e90667. [Google Scholar] [PubMed]
115. Khan M, Akhtar S, Mohsin S, S. NK, Riazuddin S. Growth factor preconditioning increases the function of diabetes-impaired mesenchymal stem cells. Stem Cells Dev. 2011;20(1):67–75. [Google Scholar] [PubMed]
116. Hersant B, Sid-Ahmed M, Braud L, Jourdan M, Baba-Amer Y, Meningaud JP, et al. Platelet-rich plasma improves the wound healing potential of mesenchymal stem cells through paracrine and metabolism alterations. Stem Cells Int. 2019;2019(3):1234263. [Google Scholar] [PubMed]
117. Naderi N, Griffin MF, Mosahebi A, Butler PE, Seifalian AM. Adipose derived stem cells and platelet rich plasma improve the tissue integration and angiogenesis of biodegradable scaffolds for soft tissue regeneration. Molecular Biol Rep. 2020;47(3):2005–13. [Google Scholar]
118. Batlle R, Andres E, Gonzalez L, Llonch E, Igea A, Gutierrez-Prat N, et al. Regulation of tumor angiogenesis and mesenchymal-endothelial transition by p38α through TGF-β and JNK signaling. Nat Commun. 2019;10(1):3071. [Google Scholar]
119. Nasser M, Wu Y, Danaoui Y, Ghosh G. Engineering microenvironments towards harnessing pro-angiogenic potential of mesenchymal stem cells. Mater Sci Eng C Mater Biol Appl. 2019;102:75–84. [Google Scholar] [PubMed]
120. Rustad KC, Wong VW, Sorkin M, Glotzbach JP, Major MR, Rajadas J, et al. Enhancement of mesenchymal stem cell angiogenic capacity and stemness by a biomimetic hydrogel scaffold. Biomaterials. 2012;33(1):80–90. [Google Scholar] [PubMed]
121. Assi R, Foster TR, He H, Stamati K, Bai H, Huang Y, et al. Delivery of mesenchymal stem cells in biomimetic engineered scaffolds promotes healing of diabetic ulcers. Regenerative Med. 2016;11(3):245–60. [Google Scholar]
122. Jin K, Li B, Lou L, Xu Y, Ye X, Yao K, et al. In vivo vascularization of MSC-loaded porous hydroxyapatite constructs coated with VEGF-functionalized collagen/heparin multilayers. Sci Rep. 2016;6(1):19871. [Google Scholar]
123. Simpson DL, Dudley SCJr. Modulation of human mesenchymal stem cell function in a three-dimensional matrix promotes attenuation of adverse remodelling after myocardial infarction. J Tissue Eng Regen Med. 2013;7(3):192–202. [Google Scholar]
124. Liang T, Zhu L, Gao W, Gong M, Ren J, Yao H, et al. Coculture of endothelial progenitor cells and mesenchymal stem cells enhanced their proliferation and angiogenesis through PDGF and Notch signaling. FEBS Open Bio. 2017;7(11):1722–36. [Google Scholar]
125. Zhang B, Yang S, Zhang Y, Sun Z, Xu W, Ye S. Co-culture of mesenchymal stem cells with umbilical vein endothelial cells under hypoxic condition. J Huazhong Univ Sci Technolog Med Sci. 2012;32(2):173–80. [Google Scholar]
126. Joensuu K, Uusitalo-Kylmälä L, Hentunen TA, Heino TJ. Angiogenic potential of human mesenchymal stromal cell and circulating mononuclear cell cocultures is reflected in the expression profiles of proangiogenic factors leading to endothelial cell and pericyte differentiation. J Tissue Eng Regen Med. 2018;12(3):775–83. [Google Scholar]
127. Liu W, Zhang G, Wu J, Zhang Y, Liu J, Luo H, et al. Insights into the angiogenic effects of nanomaterials: mechanisms involved and potential applications. J Nanobiotechnology. 2020;18(1):9. [Google Scholar] [PubMed]
128. Xie J, Zhang D, Ling Y, Yuan Q, Chenchen Z, Wei D, et al. Substrate elasticity regulates vascular endothelial growth factor A (VEGFA) expression in adipose-derived stromal cells: implications for potential angiogenesis. Colloids Surf B Biointerfaces. 2019;175:576–85. [Google Scholar]
129. Hamik A, Wang B, Jain MK. Transcriptional regulators of angiogenesis. Arterioscler Thromb Vasc Biol. 2006;26(9):1936–47. [Google Scholar]
130. Zhang Y, Liu J, Lin J, Zhou L, Song Y, Wei B, et al. The transcription factor GATA1 and the histone methyltransferase SET7 interact to promote VEGF-mediated angiogenesis and tumor growth and predict clinical outcome of breast cancer. Oncotarget. 2016;7(9):9859–75. [Google Scholar] [PubMed]
131. Xie J, Zhang D, Ling Y, Yuan Q, Chenchen Z, Wei D, et al. An engineered vascular endothelial growth factor-activating transcription factor induces therapeutic angiogenesis in ApoE knockout mice with hindlimb ischemia. J Vasc Surg. 2006;44(1):166–75. [Google Scholar]
132. Shaabani E, Sharifiaghdam M, Faridi-Majidi R, De Smedt SC, Braeckmans K, Fraire JC. Gene therapy to enhance angiogenesis in chronic wounds. Mol Ther Nucleic Acids. 2022;29:871–99. [Google Scholar]
133. Corre I, Paris F, Huot J. The p38 pathway, a major pleiotropic cascade that transduces stress and metastatic signals in endothelial cells. Oncotarget. 2017;8(33):55684–714. [Google Scholar]
134. Budgude P, Kale V, Vaidya A. Pharmacological inhibition of p38 MAPK rejuvenates bone marrow derived-mesenchymal stromal cells and boosts their hematopoietic stem cell-supportive ability. Stem Cell Rev. 2021;17(6):2210–22. [Google Scholar]
135. Rousseau S, Houle F, Kotanides H, Witte L, Waltenberger J, Landry J, et al. Vascular endothelial growth factor (VEGF)-driven actin-based motility is mediated by VEGFR2 and requires concerted activation of stress-activated protein kinase 2 (SAPK2/p38) and geldanamycin-sensitive phosphorylation of focal adhesion kinase. J Biol Chem. 2000;275(14):10661–72. [Google Scholar]
136. Issbrücker K, Marti HH, Hippenstiel S, Springmann G, Voswinckel R, Gaumann A, et al. p38 MAP kinase—a molecular switch between VEGF-induced angiogenesis and vascular hyperpermeability. FASEB J. 2003;17(2):262–4. [Google Scholar]
137. Gratton JP, Morales-Ruiz M, Kureishi Y, Fulton D, Walsh K, Sessa WC. Akt down-regulation of p38 signaling provides a novel mechanism of vascular endothelial growth factor-mediated cytoprotection in endothelial cells. J Biol Chem. 2001;276(32):30359–65. [Google Scholar]
138. Lamalice L, Houle F, Jourdan G, Huot J. Phosphorylation of tyrosine 1214 on VEGFR2 is required for VEGF-induced activation of Cdc42 upstream of SAPK2/p38. Oncogene. 2004;23(2):434–45. [Google Scholar] [PubMed]
139. Ma N, Teng X, Zheng Q, Chen P. The regulatory mechanism of p38/MAPK in the chondrogenic differentiation from bone marrow mesenchymal stem cells. J Orthop Surg Res. 2019;14(1):434. [Google Scholar]
140. Batlle R, Andrés E, Gonzalez L, Llonch E, Igea A, Gutierrez-Prat N, et al. Regulation of tumor angiogenesis and mesenchymal-endothelial transition by p38α through TGF-β and JNK signaling. Nat Commun. 2019;10(1):3071. [Google Scholar] [PubMed]
141. Hellström M, Phng LK, Hofmann JJ, Wallgard E, Coultas L, Lindblom P, et al. Dll4 signalling through Notch1 regulates formation of tip cells during angiogenesis. Cah Rev The. 2007;445(7129):776–80. doi:10.1038/nature05571. [Google Scholar] [PubMed] [CrossRef]
142. Xuan W, Khan M, Ashraf M. Extracellular vesicles from notch activated cardiac mesenchymal stem cells promote myocyte proliferation and neovasculogenesis. Front Cell Dev Biol. 2020;8:11. [Google Scholar]
143. Cheng Y, Gu W, Zhang G, Guo X. Notch1 activation of Jagged1 contributes to differentiation of mesenchymal stem cells into endothelial cells under cigarette smoke extract exposure. BMC Pulm Med. 2022;22(1):139. [Google Scholar] [PubMed]
144. Presta M, Dell’Era P, Mitola S, Moroni E, Ronca R, Rusnati M. Fibroblast growth factor/fibroblast growth factor receptor system in angiogenesis. Cytokine Growth Factor Rev. 2005;16(2):159–78. [Google Scholar]
145. Itoh N, Ornitz DM. Evolution of the Fgf and Fgfr gene families. Trends Genet. 2004;20(11):563–9. [Google Scholar]
146. Jia T, Jacquet T, Dalonneau F, Coudert P, Vaganay E, Exbrayat-Héritier C, et al. FGF-2 promotes angiogenesis through a SRSF1/SRSF3/SRPK1-dependent axis that controls VEGFR1 splicing in endothelial cells. BMC Biol. 2021;19(1):173. [Google Scholar]
147. Li J, Wei Y, Liu K, Yuan C, Tang Y, Quan Q, et al. Synergistic effects of FGF-2 and PDGF-BB on angiogenesis and muscle regeneration in rabbit hindlimb ischemia model. Microvasc Res. 2010;80(1):10–7. [Google Scholar]
148. Hao X, Månsson-Broberg A, Gustafsson T, Grinnemo KH, Blomberg P, Siddiqui AJ, et al. Angiogenic effects of dual gene transfer of bFGF and PDGF-BB after myocardial infarction. Biochem Biophys Res Commun. 2004;315(4):1058–63. [Google Scholar]
149. Cao R, Bråkenhielm E, Pawliuk R, Wariaro D, Post MJ, Wahlberg E, et al. Angiogenic synergism, vascular stability and improvement of hind-limb ischemia by a combination of PDGF-BB and FGF-2. Nat Med. 2003;9(5):604–13. [Google Scholar] [PubMed]
150. Kwon HM, Hur SM, Park KY, Kim CK, Kim YM, Kim HS, et al. Multiple paracrine factors secreted by mesenchymal stem cells contribute to angiogenesis. Vascul Pharmacol. 2014;63(1):19–28. [Google Scholar] [PubMed]
151. Lindner V, Lappi DA, Baird A, Majack RA, Reidy MA. Role of basic fibroblast growth factor in vascular lesion formation. Circ Res. 1991;68(1):106–13. [Google Scholar] [PubMed]
152. Krawczenko A, Bielawska-Pohl A, Paprocka M, Kraskiewicz H, Szyposzynska A, Wojdat E, et al. Microvesicles from human immortalized cell lines of endothelial progenitor cells and mesenchymal stem/stromal cells of adipose tissue origin as carriers of bioactive factors facilitating angiogenesis. Stem Cells Int. 2020;2020:1289380. [Google Scholar]
153. Yin T, He S, Su C, Chen X, Zhang D, Wan Y, et al. Genetically modified human placenta‐derived mesenchymal stem cells with FGF‐2 and PDGF‐BB enhance neovascularization in a model of hindlimb ischemia. Mol Med Rep. 2015;12(4):5093–9. [Google Scholar]
154. Hoeres T, Wilhelm M, Smetak M, Holzmann E, Schulze-Tanzil G, Birkmann J. Immune cells regulate VEGF signalling via release of VEGF and antagonistic soluble VEGF receptor-1. Clin Exp Immunol. 2018;192(1):54–67. [Google Scholar]
155. Kagiwada H, Yashiki T, Ohshima A, Tadokoro M, Nagaya N, Ohgushi H. Human mesenchymal stem cells as a stable source of VEGF-producing cells. J Tissue Eng Regen Med. 2008;2(4):184–9. [Google Scholar]
156. Webb NJ, Bottomley MJ, Watson CJ, Brenchley PE. Vascular endothelial growth factor (VEGF) is released from platelets during blood clotting: implications for measurement of circulating VEGF levels in clinical disease. Clin Sci. 1998;94(4):395–404. [Google Scholar]
157. Lee S, Chen TT, Barber CL, Jordan MC, Murdock J, Desai S, et al. Autocrine VEGF signaling is required for vascular homeostasis. Cell. 2007;130(4):691–703. [Google Scholar]
158. Mulligan JK, Rosenzweig SA, Young MRI. Tumor secretion of VEGF induces endothelial cells to suppress T cell functions through the production of PGE2. J Immunother. 2010;33(2):126–35. [Google Scholar]
159. Shibuya M. Vascular endothelial growth factor (VEGF) and its receptor (VEGFR) signaling in angiogenesis: a crucial target for anti-and pro-angiogenic therapies. Genes Cancer. 2011;2(12):1097–105. [Google Scholar]
160. Gabhann FM, Popel AS. Systems biology of vascular endothelial growth factors. Microcirculation. 2008;15(8):715–38. [Google Scholar]
161. Muller YA, Christinger HW, Keyt BA, de Vos AM. The crystal structure of vascular endothelial growth factor (VEGF) refined to 1.93 Å resolution: multiple copy flexibility and receptor binding. Structure. 1997;5(10):1325–38. [Google Scholar]
162. Shibuya M. Vascular endothelial growth factor and its receptor system: physiological functions in angiogenesis and pathological roles in various diseases. J Biochem. 2013;153(1):13–9. [Google Scholar]
163. Li L, Liu H, Xu C, Deng M, Song M, Yu X, et al. VEGF promotes endothelial progenitor cell differentiation and vascular repair through connexin 43. Stem Cell Res Ther. 2017;8(1):237. [Google Scholar] [PubMed]
164. Blanco R, Gerhardt H. VEGF and Notch in tip and stalk cell selection. Cold Spring Harb Perspect Med. 2013;3(1):a006569. [Google Scholar]
165. Tomanek RJ, Ishii Y, Holifield JS, Sjogren CL, Hansen HK, Mikawa T. VEGF family members regulate myocardial tubulogenesis and coronary artery formation in the embryo. Circ Res. 2006;98(7):947–53. [Google Scholar] [PubMed]
166. Carmeliet P, Ferreira V, Breier G, Pollefeyt S, Kieckens L, Gertsenstein M, et al. Abnormal blood vessel development and lethality in embryos lacking a single VEGF allele. Nature. 1996;380(6573):435–9. [Google Scholar]
167. Miquerol L, Langille BL, Nagy A. Embryonic development is disrupted by modest increases in vascular endothelial growth factor gene expression. Development. 2000;127(18):3941–6. [Google Scholar]
168. Gerhardt H, Golding M, Fruttiger M, Ruhrberg C, Lundkvist A, Abramsson A, et al. VEGF guides angiogenic sprouting utilizing endothelial tip cell filopodia. J Cell Bio. 2003;161(6):1163–77. [Google Scholar]
169. Alon T, Hemo I, Itin A, Pe’er J, Stone J, Keshet E. Vascular endothelial growth factor acts as a survival factor for newly formed retinal vessels and has implications for retinopathy of prematurity. Nat Med. 1995;1(10):1024–8. [Google Scholar] [PubMed]
170. Long J, Wang S, Zhang Y, Liu X, Zhang H, Wang S. The therapeutic effect of vascular endothelial growth factor gene-or heme oxygenase-1 gene-modified endothelial progenitor cells on neovascularization of rat hindlimb ischemia model. J Vasc Surg. 2013;58(3):756–65.e2. [Google Scholar]
171. Zigdon-Giladi H, Khutaba A, Elimelech R, Machtei EE, Srouji S. VEGF release from a polymeric nanofiber scaffold for improved angiogenesis. J Biomed Mater Res A. 2017;105(10):2712–21. [Google Scholar]
172. Cohen MH, Gootenberg J, Keegan P, Pazdur R. FDA drug approval summary: bevacizumab (Avastin®) plus carboplatin and paclitaxel as first-line treatment of advanced/metastatic recurrent nonsquamous non-small cell lung cancer. Oncologist. 2007;12(6):713–8. [Google Scholar] [PubMed]
173. Sonowal H, Shukla K, Kota S, Saxena A, Ramana KV. Vialinin A, an edible mushroom-derived p-terphenyl antioxidant, prevents VEGF-induced neovascularization in vitro and in vivo. Oxid Med Cell Longev. 2018;2018(1):1–10. [Google Scholar]
174. Zhou X, Yue GGL, Liu M, Zuo Z, Lee JKM, Li M, et al. Eriocalyxin B, a natural diterpenoid, inhibited VEGF-induced angiogenesis and diminished angiogenesis-dependent breast tumor growth by suppressing VEGFR-2 signaling. Oncotarget. 2016;7(50):82820. [Google Scholar]
175. Ball SG, Shuttleworth CA, Kielty CM. Mesenchymal stem cells and neovascularization: role of platelet-derived growth factor receptors. J Cell Mol Med. 2007;11(5):1012–30. [Google Scholar]
176. Chen L, Wu H, Ren C, Liu G, Zhang W, Liu W, et al. Inhibition of PDGF-BB reduces alkali-induced corneal neovascularization in mice. Mol Med Rep. 2021;23(4):238. [Google Scholar]
177. Abramsson A, Lindblom P, Betsholtz C. Endothelial and nonendothelial sources of PDGF-B regulate pericyte recruitment and influence vascular pattern formation in tumors. J Clin Invest. 2003;112(8):1142–51. [Google Scholar]
178. Bjarnegård M, Enge M, Norlin J, Gustafsdottir S, Fredriksson S, Abramsson A, et al. Endothelium-specific ablation of PDGFB leads to pericyte loss and glomerular, cardiac and placental abnormalities. Development. 2004;131(8):1847–57. [Google Scholar]
179. Gaengel K, Genové G, Armulik A, Betsholtz C. Endothelial-mural cell signaling in vascular development and angiogenesis. Arterioscler Thromb Vasc Biol. 2009;29(5):630–8. [Google Scholar] [PubMed]
180. Raica M, Cimpean AM. Platelet-derived growth factor (PDGF)/PDGF receptors (PDGFR) axis as target for antitumor and antiangiogenic therapy. Pharmaceuticals. 2010;3(3):572–99. [Google Scholar]
181. Betsholtz C. Insight into the physiological functions of PDGF through genetic studies in mice. Cytokine Growth Factor Rev. 2004;15(4):215–28. [Google Scholar]
182. Zhang Q, Xiang W, Xue BZ, Yi DY, Zhao HY, Fu P. Growth factors contribute to the mediation of angiogenic capacity of glioma-associated mesenchymal stem cells. Oncol Lett. 2021;21(3):215. [Google Scholar]
183. Ding W, Knox TR, Tschumper RC, Wu W, Schwager SM, Boysen JC, et al. Platelet-derived growth factor (PDGF)-PDGF receptor interaction activates bone marrow-derived mesenchymal stromal cells derived from chronic lymphocytic leukemia: implications for an angiogenic switch. Blood. 2010;116(16):2984–93. [Google Scholar]
184. Wang S, Mo M, Wang J, Sadia S, Shi B, Fu X, et al. Platelet-derived growth factor receptor beta identifies mesenchymal stem cells with enhanced engraftment to tissue injury and pro-angiogenic property. Cell Mol Life Sci. 2018;75(3):547–61. [Google Scholar]
Cite This Article
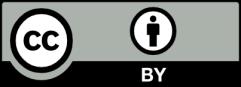
This work is licensed under a Creative Commons Attribution 4.0 International License , which permits unrestricted use, distribution, and reproduction in any medium, provided the original work is properly cited.