Open Access
ARTICLE
Changes in bioenergetics and neuroprotective properties of mesenchymal stromal cells after LPS treatment
1 A.N. Belozersky Research Institute of Physico-Chemical Biology, Lomonosov Moscow State University, Moscow, 119991, Russia
2 V.I. Kulakov National Medical Research Center of Obstetrics, Gynecology and Perinatology, Moscow, 117997, Russia
* Corresponding Author: EGOR PLOTNIKOV. Email:
(This article belongs to the Special Issue: Mitochondrial Dynamics and Oxidative Stress in Disease: Cellular Mechanisms and Therapeutic Targets)
BIOCELL 2024, 48(12), 1827-1834. https://doi.org/10.32604/biocell.2024.058496
Received 13 September 2024; Accepted 15 November 2024; Issue published 30 December 2024
Abstract
Background: The active use of stem and progenitor cells in the therapy of various diseases requires the development of approaches for targeted modification of their properties. One such approach is the induction of a pro- or anti-inflammatory phenotype. Methods: In this study, we investigated the effect of a pro-inflammatory environment in vitro on multipotent mesenchymal stromal cells (MSC) by incubation with lipopolysaccharide (LPS). iCELLigence real-time cell analysis system was used for monitoring cell culture growth. Cell energy metabolism was assessed using the Seahorse XFp Analyzer. For the rat stroke experiment, we used a photoinduced thrombosis (PT) model; after 24 h of surgery, vehicle or MSC or LPS-treated MSC was injected i.v. With magnetic resonance imaging (MRI) we evaluated the volume of ischemic brain injury. For the effect of MSC on neurological deficit after PT we used three methods: limb placement test, cylinder test, and beam-walking test. Results: LPS exposure led to a significant increase in cell growth rate and to changes in their energy metabolism: glycolytic activity increased significantly in the MSC, and non-glycolytic acidification also increased, while basic respiratory parameters were maintained. With MRI we didn’t reveal changes in the volume of brain damage between all rat groups. Neurological deficit was less only with using untreated MSC injection. Conclusion: Using LPS-treated MSC in the therapy of ischemic stroke in rats, we did not observe an increase in the neuroprotective properties of the cells, but instead noted some decrease in their therapeutic efficacy. We attribute these changes to the formation of a pro-inflammatory phenotype in MSC.Keywords
Abbreviations
2-DG | 2-deoxy-D-glucose |
CCCP | Carbonyl cyanide m-chlorophenyl hydrazone |
CI | Cell index |
DAPI | 4′,6-diamidino-2-phenylindole |
ECAR | Extracellular acidification rate |
FBS | Fetal bovine serum |
IDO | Indoleamine 2,3-dioxygenase |
IFN-γ | Interferon-gamma |
Il | Interleukin |
LPS | Lipopolysaccharide |
LPT | Limb placement test |
MCAO | Middle cerebral artery occlusion |
MMP | Metalloproteinases |
MRI | Magnetic resonance imaging |
MSC | Mesenchymal stromal cells |
OCR | Oxygen consumption rate |
PGE2 | Prostaglandin E2 |
PT | Photoinduced thrombosis |
TNF-α | Tumor necrosis factor alpha |
Stroke, a sudden disruption of cerebral blood flow, is now the second leading cause of death and the most common cause of disability worldwide, and its prevalence is steadily increasing with increasing life expectancy [1,2]. Stroke, together with other ischemic diseases of the brain, is one of the main factors limiting the length of healthy human life. Therefore, the prevention of strokes and the treatment of their consequences are urgent tasks for medicine and basic research. It was shown that the number of ischemic stroke cases, deaths, and disability-adjusted life years increased worldwide from 1990 (4.07, 2.05, and 40.50 million, respectively) to 2020 (7.86, 3.15, and 62.53 million, respectively) [2]. The number of ischemic strokes is expected to rise to 9.62 million by 2030 [2]. There are several experimental animal models of ischemic stroke that mimic human stroke and can be used to explore new treatment approaches [3]. In this study, we investigated a model of ischemic stroke caused in rats by photodynamically induced brain injury (photoinduced thrombosis, PT). The main advantages of PT are an easy-to-perform ischemic model with high reproducibility and less mortality [3].
Current methods for the treatment of ischemic stroke are very limited and are represented by two main approaches: thrombolytic therapy (intravenous administration of tissue plasminogen activator within 4.5 h of symptom onset) [4] and mechanical thrombectomy [5]. These approaches have a rather narrow therapeutic window, which limits their application, and are not always able to prevent the development of neurological deficits. Therapy based on mesenchymal multipotent stromal cells (MSC) and stem cell-derived exosomes has recently emerged as a new strategy for the treatment of various diseases, including stroke [6–8]. This is due to the unique properties of these cells, which include ease of isolation, multipotent differentiation potential, and high paracrine activity [9,10]. It has been shown that incubation of MSC with lipopolysaccharide (LPS) or their co-cultivation with leukocytes affects their production of the cytokines interleukins (IL) as IL-1a, IL-6, tumor necrosis factor alpha (TNF-α), the metalloproteinases (MMP) MMP-2 and MMP-9, resulting in an inflammatory phenotype of the cells [11,12]. In a traumatic brain injury model, the acquisition of an inflammatory phenotype by MSC not only did not reduce their therapeutic efficacy but also enabled them to more effectively reduce the volume of brain injury and exhibit more pronounced neuroprotection [13]. However, LPS’s effects on the cellular physiology of MSC, in particular energy metabolism, and the possibility of using such cells in the treatment of ischemic stroke have not yet been studied.
In this study, we investigated the effect of LPS on MSC in vitro and evaluated their neuroprotective properties in an ischemic photodynamically-induced stroke model in rats.
Assessment of LPS effect on MSC in vitro
MSC for experiments were isolated from the tibial and femoral bones of 7–9-day-old outbred rat pups by mechanical dissociation of bone marrow under sterile conditions [14]. Cells were cultured in a medium consisting of Dulbecco’s Modified Eagle Medium/Nutrient Mixture F-12 (DMEM/F-12) (1:1) (C425p and C600p, PanEco, Moscow, Russia), 10% fetal bovine serum (FBS, FB-1285, BioSera, Cholet, France), 1% glutamine (F030, PanEco, Moscow, Russia), 1% vitamins (F315, PanEco, Moscow, Russia), 1% amino acids (25-030-CI, Corning, NY, USA), and 1% penicillin (10,000 units/mL) and streptomycin (10,000 μg/mL) (PenStrep, PS-B, Capricorn, Ebsdorfergrund, Germany). The cells were maintained at 37°C with 5% CO2 in a humidified atmosphere. The cells were used for experiments after the 2nd passage. All cultures were tested for contamination with mycoplasma and its absence was confirmed.
LPS treatment of the cells was performed in a culture medium with LPS from E. coli 0127: B8 (L3129, Sigma, USA) at a concentration of 10 ng/mL [15]. Cultures incubated in a medium with the same composition but without LPS were used as a control. Cell culture growth was monitored using the real-time cell analyzer iCELLigence™ (ACEA Biosciences, Inc., San Diego, CA, USA). Cell energy metabolism was assessed using the Seahorse XFp Analyzer (Seahorse Bioscience, North Billerica, MA, USA) according to the manufacturer’s recommendations. Three measurements were performed in basal conditions and four measurements after injections of compounds affecting bioenergetics: D-glucose (10 mM, D8375, Sigma Aldrich, St. Louis, MO, USA), oligomycin (4.5 μM, 0815255, Macklin, Pudong, Shanghai, China), carbonyl cyanide m-chlorophenyl hydrazone (CCCP) (10 μM, C2759, Sigma-Aldrich, St. Louis, MO, USA), rotenone (2.5 μM, R8875, Sigma-Aldrich, St. Louis, MO, USA) and antimycin (4 μM, A-8674, Sigma-Aldrich, St. Louis, MO, USA). Seahorse XFp measured the oxygen consumption rate (OCR) in the wells, from which the respiration parameters were calculated, and the extracellular acidification rate (ECAR), from which the glycolysis parameters were calculated. The total measurement time was 130 min. Data analysis was performed using XFp Wave 2.6.1 software (wave 2.6.1., Seahorse Bioscience). The ECAR and OCR values obtained were normalized to the number of cells in the wells, which was determined by staining the cell nuclei with 4′,6-diamidino-2-phenylindole (DAPI, D1306, Thermo Fisher Scientific, Waltham, MA, USA) after metabolic analysis.
Modeling of photoinduced thrombosi
Experiments were performed on outbred male rats (weight 500–650 g, age 4 months, n = 36) obtained from the “Stolbovaya” nursery of the Scientific Center for Biomedical Technologies of the Federal Medical and Biological Agency” and maintained with a 12/12-h light/dark cycle at constant temperature (22 ± 2°C) in the animal facility of A.N. Belozersky Institute of Physico-Chemical Biology. All procedures of rat experiments were approved by the Animal Ethics Committee of the A.N. Belozersky Institute of Physico-Chemical Biology: Protocol 2/20 dated 12 February 2020.
The previously described photothrombosis protocol was used [16,17]. Rose Bengal dye (198250, Sigma-Aldrich, St. Louis, MO, USA) in sterile saline was injected into the jugular vein (3%, 40 mg/kg) under isoflurane anesthesia. After 5 min a laser with a wavelength of λ = 550 nm was aimed at the intact skull surface using the RWD 71000 automated stereotaxic instrument (RWD Life Science, Sugar Land, TX 77478, USA) according to Bregma’s stereotaxic coordinates: 1 mm anterior and 2.5 mm lateral for irradiation for 15 min. 24 h after PT induction, the rats were randomly divided into three groups: PT group received saline i.v. (n = 12), PT + MSC group received untreated MSC at a dose of 3 million cells/kg (n = 13), PT + MSCLPS group received 3 million cells/kg LPS-treated MSC (n = 11). The saline or cell suspensions were injected in the jugular vein under isoflurane (114318, laboratorios Karizoo, Barcelona, Spain) anesthesia (4% induction; 1.8% maintenance) induction with Kent Scientific SomnoSuite™ Low-Flow Anesthesia System with Integrated Digital Vaporizer (Torrington, CT, USA). The experiments with human cells were approved by the Research Ethics Board of the V.I. Kulakov National Medical Research Center for Obstetrics, Gynecology and Perinatology (Protocol No. 2 from 18 February 2022). The study was conducted by the World Health Organization’s Declaration of Helsinki, and all participants gave informed consent. Cells were cultured in DMEM/F12 (1:1) medium with 10% FBS without antibiotics. Incubation with LPS was performed for 24 h with the addition of 10 ng/mL.
Evaluation of the neurological deficit
Neurological status was assessed using the Limb Placement Test (LPT) at 7, 14, and 21 days after PT. A previously described protocol was used [18] with modifications by Jolkkonen et al. [19]. The following scores were used for each task: 2 points—normal response; 1 point—delayed and/or incomplete response; 0—no response. The total score for seven tasks was evaluated.
To assess the neurological deficit, a cylinder test was performed on day 14 after PT. The rats were placed in a glass cylinder and the number of wall touches with the forelimbs was recorded for 5 min. The proportion of wall touches with the limb on the side of the damaged hemisphere was calculated, which may indicate a neurological deficit after the injury [20].
The beam walking test [21] was performed on days 14 and 21 after PT. A special setup was used for the test, which consisted of a dark box with a double board at the exit that tapered towards the box. The board consists of a lower, wider part and a narrower upper part. Before the test, the animals underwent training in which they learned to walk on the board towards the box. For the test, each rat was placed in a dark box filled with sawdust for 5 min. The animal was then placed on the other end of the board and walked back on its own. In total, each animal underwent this test 3 times in succession as part of a test. To assess the neurological deficit, we counted the total step number, half faults (slips), and foot faults (placing the limb on the bottom board). The deficit was assessed according to the following formula:
Magnetic resonance imaging (MRI)
The study was performed on day 21 after PT. The work was performed on a tomograph with a magnetic field induction of 7 Tesla and a gradient system of 105 mT/m (BioSpec 70/30, Bruker, Germany). Animals were anesthetized with isoflurane (1.5%–2%) and placed in a positioning device with a stereotaxic and thermoregulation system as previously described [22].
A standard rat brain examination protocol was used, including the acquisition of T2-weighted images. A linear transmitter with an inner diameter of 72 mm was used to transmit the radiofrequency signals and a rat brain surface receiver coil was used to detect the radiofrequency signals. The following pulse sequences (PS) were used: RARE-PS based on a spin echo with the parameters: TR = 6000 ms, TE = 63.9 ms, slice thickness 0.8 mm with a step of 0.8 mm, matrix size 256 × 384, resolution 0.164 × 0.164 mm/pixel. The total scanning time for one animal was approximately 25 min. The degree of brain damage was assessed by graphical analysis of the MRI images with calculation of the volume of the damaged brain area using ImageJ 1.52k software (National Institutes of Health image software, Bethesda, MD, USA) in mm3.
Statistical analysis of quantitative data was performed using GraphPad Prism 6 software (GraphPad Software, Boston, MA, USA). The Shapiro-Wilk test was used to check that the sample values conformed to the normal distribution. If the compared samples passed the test for normal distribution, Student’s t-test was used when comparing two groups. If three groups of animals were compared at three different time points when calculating the LPT data, a two-way ANOVA test was used. The Holm-Sidak correction for multiple comparisons was used to analyze the data from the metabolic tests. The results of all experiments are expressed as mean ± standard error of the mean (SEM). The significance level was set at p < 0.05 (*p < 0.05, **p < 0.01). In the iCELLigence study n = 4 in each group. In the Seahorse XFp analysis, the number of replicates for each group was 4. The PT group contains 12 rats, PT + MSC contains 13 rats, and PT + MSCLPS contains 11 rats.
Evaluation of the proliferation of MSC under the influence of LPS
To estimate the growth rate of MSC cultures, we used real-time determination of the cell index, which is an integrative feature of cell number calculated by the iCELLigence device based on electrical impedance (Fig. 1A). In addition, the dependencies of cell index (CI) on time for each culture (the first derivative), which characterizes the rate of cell growth, were determined (Fig. 1A). The median value of the derivative was calculated at the point of linear cell growth. The obtained parameter reflects the kinetics of proliferation of MSC during the period of linear growth of the cell cultures. Statistical analysis revealed a significant increase in the growth rate of MSC incubated with LPS (Fig. 1B).
Figure 1: The effect of LPS on the proliferation of MSC (n = 4). (A) The kinetics of MSC proliferation: the change in the mean cell index (CI) over time and the dependence of the cell index slope in the cultures studied. (B) The mean slope of the cell index of MSC in the range of 20–30 h (mean ± SEM, **p < 0.01, Student’s t-test).
As a result of analyzing the proliferation rate of MSC in real-time, a significant increase was observed in cells incubated several times with LPS at a concentration of 10 ng/mL in 20–30 h from the start of the analysis compared to control cultures. This indicates an increase in the proliferative potential of MSC treated with LPS.
Analysis of the energy metabolism of MSC after LPS exposure
The energy metabolism of MSC, processed, and untreated LPS was analyzed. Each curve in Fig. 2A describes the change in the acidification parameter of the medium (ECAR) in the cells during the study period under basal conditions (in the absence of glucose) and after the addition of glucose or respiratory inhibitors (oligomycin, rotenone) and the CCCP. Analysis of the energy status of the MSC cultures showed that LPS caused a significant increase in the following parameters: basal glycolysis, glycolytic ability, and non-glycolytic acidification (Fig. 2B). Another important effect of LPS exposure on MSC is a significant decrease in glycolytic reserve (Fig. 2B).
Figure 2: Assessment of glycolytic activity in MSC (n = 4). (A) Change in the rate of extracellular acidification by MSC cultures; (B) The values of glycolytic parameters of MSC calculated by ECAR. Data are presented as mean ± SEM (*p < 0.05, Student’s t-test, n = 4 in each group).
Respiration of MSC treated with LPS was also evaluated separately under basal conditions (cells were in a reaction medium) and after sequential addition of substances affecting cell respiration (Fig. 3A). A comparative analysis of oxygen consumption rate (OCR) showed that the cultures did not differ significantly in any of the estimated respiration parameters, indicating a similar level of oxidative phosphorylation in control cultures of MSC and in cultures exposed to LPS (Fig. 3B).
Figure 3: The effect of LPS on the respiration of MSC (n = 4). (A) The rate of oxygen consumption of MSC cultures; (B) The values of MSC respiration parameters calculated from the rate of oxygen consumption under different conditions. The data are presented as mean ± SEM, n = 4 in each group.
The effect of MSC on the volume of brain damage in the PT model
Analysis of the volume of ischemic brain injury in rats by MRI (Fig. 4A,B) was performed on day 14 after PT modeling and revealed no significant differences between the groups of animals injected with saline (PT), a suspension of MSC (PT + MSC) and a suspension of MSC treated with LPS (PT + MSCLPS). The average damage volume in the PT group was (41 ± 8.3) mm3, in the PT + MSC group (44.4 ± 9) mm3, and in the PT + MSCLPS group (48 ± 9) mm3. We attribute the lack of effect of MSC on infarct volume to the fact that the cells were injected 24 h after stroke simulation when the lesion was already fully formed and it was very difficult to influence its size.
Figure 4: Evaluation of the neuroprotective effect of MSC and MSCLPS on the severity of brain damage after PT in rats. (A) Representative T2-weighted MRI images of brain slices taken 21 days after stroke induction (the scale bar is 5 mm); (B) The damage volume assessed by MRI. Data are presented as mean ± SEM. In the PT group n = 12, in PT + MSC group n = 13, PT + MSCLPS group n = 11.
The effect of MSC on neurological deficit after PT
PT caused a significant decrease in neurological status in rats in the LPT test from 14 points before surgery to 3.2, 4.6, and 4.8 points in animals 7 days after PT in the PT, PT + MSC, and PT + MSCLPS groups, respectively (Fig. 5A). Intact MSC caused an improvement in neurological status in both periods analyzed, indicating their neuroprotective effect. On day 14 of the test, a significant difference was observed between the three study groups. In the PT + MSC group, the scores were 1.8 times higher than in the PT group and 2.3 times higher than in the PT + MSCLPS group.
Figure 5: Assessment of the neuroprotective effect of MSC and MSCLPS after induction of brain damage in rats. (A) The effect of MSC and MSCLPS on neurological status determined by the LPT test within 21 days after PT, data are presented as mean ± SEM, **p < 0.01 (two-way ANOVA); In PT group n = 12, in PT + MSC group n = 13, PT + MSCLPS group n = 11; (B) The effect of MSC and MSCLPS on asymmetry of forelimb use estimated by the cylinder test 21 days after PT, the three-color column reflects 100%.
Fig. 5B shows the data of the “Cylinder” test performed on the 14th day after PT. There is a slight decrease in the use of a damaged paw in the MSCLPS group (1.8 out of 100%) compared to PT and PT + MSC (13% and 19%, respectively).
The “Beam-walking” test revealed no statistically significant differences between the desired groups (Fig. 6A–C). A more pronounced deficiency was observed for the hind paw compared to the front paw (Fig. 6A,B). There was a tendency to decrease the severity of the deficiency in the PT + MSC group, calculated for the hind limb of the animals (p = 0.057) (Fig. 6B).
Figure 6: Assessment of the severity of the neurological deficit (%) using the beam-walking test. (A) Calculation of the effect of MSC and MSCLPS on the severity of the deficits in the forelimb of the animals; (B) Calculation of the effect of MSC and MSCLPS on the severity of the deficits in the hindlimb of the animals; (C) Summarized data on the deficits in the forelimb and hindlimb of the rats. Data are presented as mean ± SEM, two-way ANOVA, In PT group n = 12, in PT + MSC group n = 13, PT + MSCLPS group n = 11.
The main approaches to the treatment of ischemic stroke are limited by the time window of several hours (4.5 h). Recently, several treatment methods have been developed that can be used at a later stage. In particular, the use of MSC (cell therapy) is possible within 48 h of symptoms [23] or even has some efficacy in restoring neurological deficits when administered one month after a stroke [24]. It has also been shown that MSC therapy 24 h after a stroke (middle cerebral artery occlusion, MCAO model) led to a significant improvement in the recovery of sensory and motor functions [25]. MSC have many advantages: they are able to avoid the recipient’s immune response, they are easy to obtain, propagate and potentially store for a long period of time. In addition, it is possible to modify their properties in vitro to modulate their therapeutic potential [11]. Previously, it has been shown that the MSC phenotype induced by a proinflammatory environment can enhance the neuroprotective potential of cell therapy in a traumatic brain injury model [13].
In this work, we have shown for the first time the effect of LPS on the key physiological parameters of MSC: the proliferative capacity of MSC and glycolysis. Thus, one of the most interesting effects that LPS had on MSC was an increase in their glycolytic activity with unchanged respiration parameters. That is, in the MSC exposed to LPS, there was a shift in energy metabolism towards aerobic glycolysis, which should increase their resistance to hypoxia and could also provide an additional influx of NADH equivalents. Interestingly, our results are consistent with data from other authors on changes in MSC energy metabolism towards glycolysis induced by other proinflammatory factors like TNF-α and interferon-gamma (IFN-γ) [26–28]. Metabolic reprogramming towards glycolysis has been shown to enhance the immunomodulatory potential of MSC through increased production of indoleamine 2,3-dioxygenase (IDO) and prostaglandin E2 (PGE2) [26,27]. IDO-mediated conversion of tryptophan to kynurenine causes suppression of the whole T-cell population, while regulatory T cells are stimulated [29]. It has also been shown that MSC treated with IFN-γ has greater therapeutic potential in the treatment of ischemic brain injury (MCAO) [30]. Therefore, we expected that cells with a predominance of glycolysis would also have great neuroprotective properties.
When using MSC 24 h after modeling ischemic photodynamic-induced stroke, we found the opposite effect than expected. The cells treated with LPS had lower therapeutic efficacy. Fig. 5 shows that the use of native stem cells on the 7th and 14th day after the stroke leads to an improvement in the neurological status of the animals. This effect is lost when cells incubated with LPS are used. The same trend was observed when measuring the neurological deficit of rats in the beam-walking test (Fig. 6). In the above-mentioned work where female rats were subjected to cell therapy in the MCAO model [30], MSC were injected into the bloodstream 3 h after reperfusion and at a greater number of 5 × 106 cells/kg. These differences may partly explain the discrepancy in the results. Despite the fact that in our work we could not show an improvement in the therapeutic ability of MSC after exposure to LPS in the ischemic stroke model, the possibility of changing the properties of cells during cell therapy remains an interesting task and requires further research. It can be assumed that a stroke caused by photothrombosis has a different pathogenesis than other models in which proinflammatory primed MSC are effective. This points to the need to specifically modify the properties of MSC and test them in different stroke models.
Limitations of this study: (1) Ischemic stroke is a heterogeneous disease with complex pathophysiology and there is no single model that mimics all aspects of human stroke. Therefore, the results from the rat experiments must be carefully extrapolated to human stroke. (2) We used cell therapy 24 h after stroke onset. We did not try other time points for MSC injection, possibly an earlier time could be more effective. But in real life where people with stroke arrive at the hospital within a few hours such a therapy is impossible. It was shown that arrival at the hospital 4.5 h after stroke symptoms onset was an independent predictor of a high degree of functional disability [31,32]. And this is a very important issue that we have tried to take into account.
The incubation of MSC with LPS led to a significant increase in the growth rate and a change in the energy metabolism of the cells. When such MSC were used to treat ischemic strokes in rats, a slight decrease in therapeutic efficacy was observed.
Acknowledgement: None.
Funding Statement: The study was supported by Russian science foundation (grant 21-75-30009).
Author Contributions: The authors confirm contribution to the paper as follows: study conception and design: Elmira Yakupova, Valentina Babenko, Alexey Bocharnikov, Kseniya Fedulova; analysis and interpretation of results: Elmira Yakupova, Valentina Babenko, Denis Silachev, Egor Plotnikov; draft manuscript preparation: Elmira Yakupova and Egor Plotnikov. All authors reviewed the results and approved the final version of the manuscript.
Availability of Data and Materials: The datasets used and analyzed during the current study are available from the corresponding authors upon reasonable request.
Ethics Approval: All procedures of rat experiments were approved by the Animal Ethics Committee of the A.N. Belozersky Institute of Physico-Chemical Biology (Protocol 2/20 dated 12 February 2020). The experiments with human cells were approved by the Research Ethics Board of the V.I. Kulakov National Medical Research Center for Obstetrics, Gynecology and Perinatology (Protocol No. 2 from 18 February 2022). The study was conducted by the World Health Organization’s Declaration of Helsinki, and all participants gave informed consent.
Conflicts of Interest: The authors declare no conflicts of interest to report regarding the present study.
References
1. GBD 2019 Stroke Collaborators. Global, regional, and national burden of stroke and its risk factors, 1990–2019: a systematic analysis for the Global Burden of Disease Study 2019. Lancet Neurol. 2021;20(10):795–820. [Google Scholar]
2. Pu L, Wang L, Zhang R, Zhao T, Jiang Y, Han L. Projected global trends in ischemic stroke incidence, deaths and disability-adjusted life years from 2020 to 2030. Stroke. 2023;54(5):1330–9. doi:10.1161/STROKEAHA.122.040073. [Google Scholar] [PubMed] [CrossRef]
3. Li Y, Zhang J. Animal models of stroke. Animal Model Exp Med. 2021;4(3):204–19. doi:10.1002/ame2.12179. [Google Scholar] [PubMed] [CrossRef]
4. Hacke W, Kaste M, Bluhmki E, Brozman M, Dávalos A, Guidetti D, et al. Thrombolysis with alteplase 3 to 4.5 hours after acute ischemic stroke. N Engl J Med. 2008;359(13):1317–29. doi:10.1056/NEJMoa0804656. [Google Scholar] [PubMed] [CrossRef]
5. Jovin TG, Chamorro A, Cobo E, de Miquel MA, Molina CA, Rovira A, et al. Thrombectomy within 8 hours after symptom onset in ischemic stroke. N Engl J Med. 2015;372(24):2296–306. doi:10.1056/NEJMoa1503780. [Google Scholar] [PubMed] [CrossRef]
6. Liu X, Jia X. Neuroprotection of stem cells against ischemic brain injury: from bench to clinic. Transl Stroke Res. 2024;15(4):691–713. [Google Scholar] [PubMed]
7. Pinosanu LR, Wolff N, Olaru DG, Popa-Wagner A. Stem cell treatments in preclinical relevant stroke models. Curr Health Sci J. 2023;49(4):487–94. [Google Scholar] [PubMed]
8. Dehghani L, Hashemi SM, Saadatnia M, Zali A, Oraee-Yazdani S, Heidari Keshel S, et al. Stem cell-derived exosomes as treatment for stroke: a systematic review. Stem Cell Rev Rep. 2021;17(2):428–38. [Google Scholar] [PubMed]
9. Li W, Shi L, Hu B, Hong Y, Zhang H, Li X, et al. Mesenchymal stem cell-based therapy for stroke: current understanding and challenges. Front Cell Neurosci. 2021;15:628940. [Google Scholar] [PubMed]
10. Gopalarethinam J, Nair AP, Iyer M, Vellingiri B, Subramaniam MD. Advantages of mesenchymal stem cell over the other stem cells. Acta Histochem. 2023;125(4):152041. [Google Scholar] [PubMed]
11. Plotnikov E, Danilina T, Pulkova N, Pevzner I, Zorova L, Silachev D, et al. Fp037 influence of inflammation on MMSC: anti-inflammatory priming or switching to inflammatory phenotype. Nephrol Dial Transplant. 2018;33(1):i59–60. [Google Scholar]
12. Maghsood F, Johari B, Ghahhari NM, Moradi M, Kadivar M. Conditioned medium of lipopolysaccharide-treated embryonic stem cell-derived mesenchymal stem cells modulates in vitro secretion of inflammatory cytokines. Thai J Pharm Sci. 2020;44(3):8. [Google Scholar]
13. Danilina TI, Silachev DN, Pevzner IB, Gulyaev MV, Pirogov YA, Zorova LD, et al. The influence of proinflammatory factors on the neuroprotective efficiency of multipotent mesenchymal stromal cells in traumatic brain injury. Bull Exp Biol Med. 2017;163(4):528–34. [Google Scholar] [PubMed]
14. Babenko VA, Silachev DN, Danilina TI, Goryunov KV, Pevzner IB, Zorova LD, et al. Age-related changes in bone-marrow mesenchymal stem cells. Cells. 2021;10(6):1273. doi:10.3390/cells10061273. [Google Scholar] [PubMed] [CrossRef]
15. Wang K, Chen Z, Jin L, Zhao L, Meng L, Kong F, et al. LPS-pretreatment adipose-derived mesenchymal stromal cells promote wound healing in diabetic rats by improving angiogenesis. Injury. 2022;53(12):3920. [Google Scholar] [PubMed]
16. Watson BD, Dietrich WD, Busto R, Wachtel MS, Ginsberg MD. Induction of reproducible brain infarction by photochemically initiated thrombosis. Ann Neurol. 1985;17(5):497–504. [Google Scholar] [PubMed]
17. Romanova GA, Shakova FM, Kovaleva OI, Pivovarov VV, Khlebnikova NN, Karganov MY. Relationship between changes in rat behavior and integral biochemical indexes determined by laser correlation spectroscopy after photothrombosis of the prefrontal cortex. Bull Exp Biol Med. 2004;137(2):135–8. [Google Scholar] [PubMed]
18. De Ryck M, Van Reempts J, Borgers M, Wauquier A, Janssen PA. Photochemical stroke model: flunarizine prevents sensorimotor deficits after neocortical infarcts in rats. Stroke. 1989;20(10):1383–90. [Google Scholar] [PubMed]
19. Jolkkonen J, Puurunen K, Rantakömi S, Härkönen A, Haapalinna A, Sivenius J. Behavioral effects of the alpha(2)-adrenoceptor antagonist, atipamezole, after focal cerebral ischemia in rats. Eur J Pharmacol. 2000;400(2–3):211–9. [Google Scholar] [PubMed]
20. Rattka M, Fluri F, Krstić M, Asan E, Volkmann J. A novel approach to assess motor outcome of deep brain stimulation effects in the hemiparkinsonian rat: staircase and cylinder test. J Vis Exp. 2016;(111):53951. doi:10.3791/53951. [Google Scholar] [PubMed] [CrossRef]
21. Shallert T, Woodlee MT, Fleming SM. Disentangling multipletypes of recovery from brain injury. Pharmacol Cereb Ischemia. 2002;2002:201–16. [Google Scholar]
22. Silachev DN, Uchevatkin AA, Pirogov YA, Zorov DB, Isaev NK. Comparative evaluation of two methods for studies of experimental focal ischemia: magnetic resonance tomography and triphenyltetrazoleum detection of brain injuries. Bull Exp Biol Med. 2009;147(2):269–72. doi:10.1007/s10517-009-0489-z. [Google Scholar] [PubMed] [CrossRef]
23. Vu Q, Xie K, Eckert M, Zhao W, Cramer SC. Meta-analysis of preclinical studies of mesenchymal stromal cells for ischemic stroke. Neurology. 2014;82(14):1277–86. doi:10.1212/WNL.0000000000000278. [Google Scholar] [PubMed] [CrossRef]
24. Shen LH, Li Y, Chen J, Zacharek A, Gao Q, Kapke A, et al. Therapeutic benefit of bone marrow stromal cells administered 1 month after stroke. J Cereb Blood Flow Metab. 2007;27(1):6–13. doi:10.1038/sj.jcbfm.9600311. [Google Scholar] [PubMed] [CrossRef]
25. Nalamolu KR, Chelluboina B, Fornal CA, Challa SR, Pinson DM, Wang DZ, et al. Stem cell treatment improves post stroke neurological outcomes: a comparative study in male and female rats. Stroke Vasc Neurol. 2021;6(4):519–27. doi:10.1136/svn-2020-000834. [Google Scholar] [PubMed] [CrossRef]
26. Liu Y, Yuan X, Muñoz N, Logan TM, Ma T. Commitment to aerobic glycolysis sustains immunosuppression of human mesenchymal stem cells. Stem Cells Transl Med. 2019;8(1):93–106. [Google Scholar] [PubMed]
27. Jitschin R, Böttcher M, Saul D, Lukassen S, Bruns H, Loschinski R, et al. Inflammation-induced glycolytic switch controls suppressivity of mesenchymal stem cells via STAT1 glycosylation. Leukemia. 2019;33(7):1783–96. [Google Scholar] [PubMed]
28. Contreras-Lopez R, Elizondo-Vega R, Luque-Campos N, Torres MJ, Pradenas C, Tejedor G, et al. The ATP synthase inhibition induces an AMPK-dependent glycolytic switch of mesenchymal stem cells that enhances their immunotherapeutic potential. Theranostics. 2021;11(1):445–60. [Google Scholar] [PubMed]
29. Munn DH, Mellor AL. IDO in the Tumor microenvironment: inflammation, counter-regulation, and tolerance. Trends Immunol. 2016;37(3):193–207. [Google Scholar] [PubMed]
30. Tobin MK, Stephen TKL, Lopez KL, Pergande MR, Bartholomew AM, Cologna SM, et al. Activated mesenchymal stem cells induce recovery following stroke via regulation of inflammation and oligodendrogenesis. J Am Heart Assoc. 2020;9(7):e013583. [Google Scholar] [PubMed]
31. Moraes MA, Jesus PA, Muniz LS, Baccin CA, Barreto ABM, Sales RS, et al. Arrival time at a referral hospital and functional disability of people with stroke: a cohort study. Sao Paulo Med J. 2023;141(6):e2022510. doi:10.1590/1516-3180.2022.0510.r1.27022023. [Google Scholar] [PubMed] [CrossRef]
32. Sheikh Hassan M, Yucel Y. Factors influencing early hospital arrival of patients with acute ischemic stroke, cross-sectional study at teaching hospital in Mogadishu Somalia. J Multidiscip Healthc. 2022;15:2891–9. doi:10.2147/JMDH.S392922. [Google Scholar] [PubMed] [CrossRef]
Cite This Article
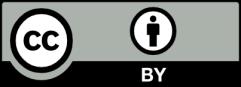
This work is licensed under a Creative Commons Attribution 4.0 International License , which permits unrestricted use, distribution, and reproduction in any medium, provided the original work is properly cited.