Open Access
ARTICLE
Cold exposure induces ovarian dysfunction through endoplasmic reticulum stress-mediated apoptosis
1 College of Basic Medicine, Hebei University of Chinese Medicine, Shijiazhuang, 050200, China
2 College of Integrative Chinese and Western Medicine, Hebei University of Chinese Medicine, Shijiazhuang, 050200, China
3 Hebei Key Laboratory of Integrative Medicine on Liver-Kidney Patterns, Shijiazhuang, 050091, China
4 College of Acupuncture and Massage, Hebei University of Chinese Medicine, Shijiazhuang, 050200, China
5 College of Pharmacy, Hebei University of Chinese Medicine, Shijiazhuang, 050200, China
* Corresponding Author: XIUMEI CHENG. Email:
# Xiaodan Song and Yuqing Wang contributed equally to this article
(This article belongs to the Special Issue: Cell Death in Health and Disease: Diversity, Complexity, and Dynamics)
BIOCELL 2024, 48(12), 1761-1772. https://doi.org/10.32604/biocell.2024.057203
Received 11 August 2024; Accepted 20 November 2024; Issue published 30 December 2024
Abstract
Background: Prior research has established that exposure to low temperatures adversely affects ovarian function, yet the precise mechanisms remain to be elucidated. Methods: Thirty experimental rats, each demonstrating two regular estrous cycles, were assorted randomly into three distinct groups: a control group, a cold exposure group, and a group treated with 4-phenylbutyric acid (4-PBA). To mimic the conditions of cold exposure, rats in the cold exposure and 4-PBA groups were subjected to immersion in ice water for 21 days. After 7 days of exposure to ice water, the 4-PBA group received intraperitoneal injections of a 20 mg/mL solution of 4-PBA at a dose of 100 mg/kg/day for 14 days. Estrous cycles were monitored via analysis of vaginal secretion smears. Serum hormone levels were measured using enzyme-linked immunosorbent assay (ELISA) kits. Ovarian morphology and the ultrastructure of granulosa cells (GCs) were evaluated using hematoxylin and eosin (HE) staining, and transmission electron microscopy (TEM), respectively. Apoptotic levels in GCs were quantified using a terminal deoxyribonucleotidyl transferase-mediated dUTP nick end labeling (TUNEL) kit. Expression of apoptotic markers, endoplasmic reticulum stress (ERS) indicators, and molecules associated with relevant pathways were assessed through immunohistochemistry, western blot, and quantitative real-time polymerase chain reaction (qRT-PCR). Results: Dysregulation of the estrous cycle was notable in rats exposed to cold. The cold exposure group exhibited significantly reduced levels of estradiol (E2) and progesterone (P) compared to controls, alongside an increase in follicular atresia and a reduction in the count of developing follicles. After cold exposure, enhanced apoptosis and ERS were evident, with activation of the protein kinase RNA-like endoplasmic reticulum kinase (PERK)/eukaryotic translation initiation factor 2α (eIF2α)/activating transcription factor 4 (ATF4) signaling pathway in ovarian. Notable changes were also observed in the ultrastructure of ovarian GCs, where both apoptosis and ERS levels were increased. Conversely, treatment with 4-PBA alleviated disturbances in the estrous cycle and hormonal imbalances, improved ovarian morphology, and alleviated apoptosis and ERS, effectively inhibiting the PERK/eIF2α/ATF4 pathway. Additionally, 4-PBA treatment significantly improved the ultrastructure of GCs and alleviated apoptosis and ERS in these cells within the cold exposure group. Conclusion: These findings suggest that ERS plays a significant role in ovarian dysfunction induced by cold exposure, primarily through promoting apoptosis.Keywords
Abbreviations
ERS | Endoplasmic reticulum stress |
UPR | Unfolded protein response |
4-PBA | 4-phenylbutyric acid |
GCs | Granulosa cells |
E2 | Estradiol |
P | Progesterone |
FSH | Follicle-stimulating hormone |
TEM | Transmission electron microscopy |
GRP78 | Glucose-regulated protein 78 |
CHOP | C/EBP homologous protein |
PERK | Protein kinase RNA-like endoplasmic reticulum kinase |
eIF2α | Eukaryotic translation initiation factor 2α |
ATF4 | Activating transcription factor 4 |
Exposure to temperatures outside the optimal range can adversely impact health, with cold environments posing a greater mortality risk than hot ones due to suboptimal thermal conditions [1,2]. Extensive research has conclusively shown that exposure to cold significantly increases the likelihood of developing diseases in the respiratory, cardiovascular, and reproductive systems [3–5]. Particularly in women, prolonged exposure to low temperatures may lead to various reproductive health issues, including irregular menstrual cycle, amenorrhea, and infertility, which severely affect their health and quality of life [6–8]. Studies have indicated that exposure to cold may prolong the estrous cycle, disrupt reproductive hormone levels, cause abnormal follicular development and ovulation, and microcirculation disorders in the reproductive organs in female rats or mice [9–11]. However, further research is needed to understand the mechanisms by which cold exposure disrupts female reproductive function.
The ovary is an essential component of the female reproductive system, with roles in oocyte differentiation and release, as well as in the synthesis and secretion of crucial reproductive hormones that are vital for the menstrual/estrous cycle. Follicles, the primary structural and functional unit within the ovary, are pivotal in determining a female’s reproductive capacity. The follicular microenvironment is influenced by various factors, with recent studies identifying ERS as a significant local factor in various ovarian physiopathology [12]. In ovarian physiology, the ERS is activated in the GCs of growing follicles and is involved in a series of events, including gonadotropin expression, oocyte maturation, and follicular atresia [12]. In ovarian pathology, ERS can be activated by several factors, compromising the health of follicles and oocytes through pro-apoptotic effects and by influencing other local factors in the follicular microenvironment, leading to the occurrence of various reproductive endocrine disorders [12–14]. As the main functional cells of the ovary, abnormal apoptosis of GCs is a key factor in follicular dysplasia and atresia and is closely associated with ovarian dysfunction [14–16].
The endoplasmic reticulum (ER) is an organelle within the cell responsible for protein synthesis, folding, and transport, playing a crucial role in maintaining cellular homeostasis. Accumulation of misfolded or incompletely folded proteins in the ER triggers ERS, leading to the unfolded protein response (UPR) [17]. This response initially aims to restore normal function but can lead to apoptosis and induce pathological damage in tissues if ERS is severe or prolonged [18]. Research has shown that cold, a common environmental stressor, can disrupt the environment of the ER, activating ERS and apoptosis, which damages tissues and organs [19,20]. It remains unclear whether cold exposure-induced ovarian dysfunction is related to ERS-induced apoptosis. Our hypothesis posits that apoptosis induced by ERS may be a critical factor in the ovarian dysfunction observed after exposure to cold. Our study focuses on the roles of ERS and apoptosis in GCs. In addition, 4-PBA was used to intervene in cold-exposed rats. 4-PBA is a specific inhibitor of the ERS, which stabilizes peptide structures and enhances the ability of the ER to fold proteins [21]. Recent studies have confirmed that 4-PBA can alleviate ovarian cell apoptosis and improve ovarian function by inhibiting ERS [22,23].
Specific pathogen-free female Sprague-Dawley rats (six weeks old) were obtained from Beijing Vital River Laboratory Animal Technology Co., Ltd. (Beijing, China) (Permit Number: SCXK (Beijing) 2021-0011). The rats were housed in an environment with suitable light (12-h light/dark cycle), temperature (24 ± 1°C) and humidity (50%–70%) with unrestricted access to food and water.
Establishment of the cold exposure model and experimental design
Following 5 days of acclimatization, vaginal secretions were collected daily for 10 consecutive days to assess the estrous cycle of thirty selected experimental animals, each of which had previously demonstrated two regular estrous cycles. After this period, the animals were equally and randomly assigned to three distinct groups: the control group (n = 10), the cold exposure group (n = 10), and the 4-PBA group (n = 10).
The control group underwent no experimental interventions and was left undisturbed for the duration of the experiment. Conversely, the cold exposure and 4-PBA groups were subjected to a defined cold stress protocol, which involved daily immersion in ice water at 0°C–1°C to a depth of 10 cm for 20 min, consistently over a 21-day period. This protocol was established using the Cheng Xiumei pharmacological method [24], a model frequently employed in pharmacological studies to simulate the physiological effects of cold on women [9,25].
For the 4-PBA treatment preparation, 4-PBA was dissolved in saline. Equimolar amounts of 4-PBA (HY-A0281, MedChemExpress, Shanghai, China) and sodium hydroxide were titrated to prepare an injection solution with a pH of 7.4 and a 4-PBA concentration of 20 mg/mL. Following 7 days of cold exposure, the 4-PBA group was administered intraperitoneal injections of this solution at 100 mg/kg/day for 14 days.
Sample collection and preparation
Upon establishing the experimental model and administering the pharmaceuticals, surgical procedures were conducted on the rats during their diestrus phase. Isoflurane was utilized as an inhalation anesthetic for these procedures. Following anesthesia, serum was obtained by extracting blood from the abdominal aorta, and then the ovaries were excised for subsequent experimental analyses. The experimental protocol received approval from the Ethics Committee of Hebei University of Chinese Medicine (DWLL 2021025). All animals were treated humanely.
Observation of the estrous cycle
Vaginal smears were collected between 8:00 and 8:30 AM. Gently fondling the rat to expose its vagina, followed by the insertion of a sterile cotton swab moistened with saline into the vaginal canal. The swab was carefully rotated two to three times before being removed. The secretions were then uniformly spread onto a clean microscope slide and allowed to air-dry briefly. The samples were subsequently stained with methylene blue solution (Solarbio, G1300, Beijing, China) and observed under an optical microscope (Olympus, DP72CCD, Tokyo, Japan) to determine the current phase of the estrous cycle.
The concentrations of E2, P, and follicle-stimulating hormone (FSH) in the serum were quantified using specialized ELISA kits tailored for rats. These kits, sourced from Elabscience (model numbers E-OSEL-R0001 for E2, E-OSEL-R0007 for P, and E-EL-R0391 for FSH; Wuhan, China), enabled precise measurement of these hormone levels.
Immediately after isolation, the ovaries were fixed in 4% paraformaldehyde (Servicebio, G1101, Wuhan, China) for a period of 24 h. This was followed by a series of steps including dehydration, transparency, wax immersion, and embedding. The tissues were then sectioned to produce slices 4 µm thick, which were mounted on slides. Staining of these sections was carried out using hematoxylin (Servicebio, G1004) and eosin (Servicebio, G1001), enabling detailed examination of ovarian architecture.
Fixation of the ovarian tissues was initially performed using a 2.5% glutaraldehyde solution (Servicebio, G1102), followed by secondary fixation in 1% osmium tetroxide. The dehydration process utilized a graded series of concentrations of alcohol, subsequently embedding the tissues in EMBed 812 resin. Ultrathin sections of 60 nm were stained with 2% uranyl acetate and 2.6% lead citrate. These preparations enabled the detailed examination of the ultrastructure of the GCs via TEM.
A TUNEL assay (G1501, Servicebio) was utilized for the identification of apoptotic cells in ovarian tissue sections. Concurrently, cell nuclei were stained using a DAPI solution (C0065, Solarbio). Observations of the stained specimens were conducted using a fluorescence microscope (Thermo Fisher, EVOS FL, Waltham, MA, USA). For quantitative analysis, the staining intensity was assessed by measuring the average optical density using ImageJ software, version 1.53 (National Institutes of Health, Bethesda, MD, USA), which facilitated precise comparisons of staining levels among different samples.
Protein extraction from ovarian tissues was conducted using RIPA lysis buffer (Servicebio, G2002), supplemented with a protease inhibitor cocktail (MedChemExpress, HY-K0010), phenylmethylsulfonyl fluoride (PMSF, Servicebio, G2008), and a phosphorylation-specific protease inhibitor (Servicebio, G2007). The quantification of protein levels was carried out with the aid of a BCA protein assay kit (Seven, SW101-02, Beijing, China). Proteins, thoroughly mixed with 5× loading buffer (Servicebio, G2013), were denatured by boiling and then separated electrophoretically on SDS-PAGE at 20 µg per sample. Following separation, these proteins were then electroblotted onto polyvinylidene fluoride membranes (Millipore, 24937-79-9, Shanghai, China), and blocked with 5% skim milk (Seven, SW128) to minimize non-specific binding. The membranes were incubated overnight at a temperature of 4°C with specific primary antibodies. After primary antibody incubation, membranes were washed with TBST (Servicebio, G0004) and then incubated with horseradish peroxidase-linked secondary antibodies (1:5000, Proteintech, SA00001-1, SA00001-2, Wuhan, China) for 1 h at room temperature. Post-secondary antibody incubation, membranes underwent further washing with TBST and TBS (Servicebio, G0001). Antigen-antibody complexes were detected using a touch imager system (eBlot, Touch Imager, Shanghai, China). Primary antibodies included in this study were glucose-regulated protein 78 (GRP78) (1:1000, Proteintech, 11587-1-AP), PERK (1:1000, Cell Signaling Technology, 3192, Danvers, MA, USA), phosphorylated PERK (p-PERK) (1:500, Bioss, bs-23340R, Beijing, China), eIF2α (1:1000, Cell Signaling Technology, 5324), phosphorylated eIF2α (p-eIF2α) (1:1000, Cell Signaling Technology, 3398), ATF4 (1:1000, Proteintech, 10835-1-AP), C/EBP homologous protein (CHOP) (1:1000, Immunoway, YM3668, Plano, TX, USA), Caspase-12 (1:1000, Servicebio, GB111695), Caspase-3 (1:1000, Cell Signaling Technology, 9662), B-cell lymphoma 2 (Bcl-2) (1:1000, Proteintech, 26593-1-AP), BCL2-associated X (Bax) (1:5000, Proteintech, 60267-1-Ig), β-tubulin (1:5000, Proteintech, 66240-1-Ig), and GAPDH (1:5000, Proteintech, 60004-1-Ig).
Ovarian paraffin sections underwent dewaxing and rehydration. To inhibit endogenous peroxidase activity, the tissue sections were treated with 3% hydrogen peroxide (ZSGB-BIO, SP-9000, Beijing, China) for 10 min. Antigen retrieval was then carried out using a citric acid-based solution (Solarbio, C1010), heated to between 95°C and 100°C for 20 min. To permeabilize the cell membranes, the tissues were incubated with a permeabilization solution (Servicebio, G1204) containing 0.5% Triton X-100 for 30 min at room temperature. For blocking, goat serum (ZSGB-BIO, SP-9000) was applied at room temperature for 20 min, preparing the sections for an overnight incubation at 4°C with primary antibodies. The following day, these sections were incubated for 1 h at 37°C with secondary antibodies (ZSGB-BIO, SP-9000) and for 15 min at 37°C with horseradish peroxidase-conjugated components (ZSGB-BIO, SP-9000). Staining was performed using DAB (ZSGB-BIO, ZLI-9018) to visualize target proteins under a light microscope, followed by hematoxylin for nuclear staining. Post-staining differentiation was achieved using hydrochloric acid, after which the sections were rinsed in running water to develop a blue hue in the stains. This was followed by final dehydration, clearing, and mounting of the slides. Microscopic analyses of the sections were performed, and the detailed images obtained were quantitatively assessed using ImageJ 1.53. The primary antibodies used in this study included GRP78 (1:150), CHOP (1:100), Bcl-2 (1:100), and Bax (1:500).
RNA isolation was meticulously performed on tissue specimens using the OMEGA Total RNA Extraction Kit (R6934, Dallas, TX, USA), which facilitated the extraction of high-quality total RNA. This extracted RNA subsequently underwent a reverse transcription process to synthesize cDNA, executed with the SweScript RT Kit (Servicebio, G3337). Subsequent quantification of cDNA was carried out in real time using the 2× SYBR Green qPCR Master Mix (Servicebio, G3321). β-actin served as the normalization control to ensure accurate relative quantification of target gene transcripts. Quantitative assessments of these transcripts were performed using the 2−ΔΔCt method, with each measurement conducted in triplicate. Primers for each target gene were designed by Wuhan Servicebio Biotechnology Co. (China), and the primer sequences for GRP78, CHOP, ATF4, Bcl-2, Bax, and β-actin are listed in Table 1.
Data measurements are reported as mean ± standard deviation. To ensure the robustness and uniformity of the results, each experiment was conducted independently at least three times. Statistical analyses were performed using SPSS 25 software (IBM Corporation, Armonk, NY, USA), and graphical representations were generated with GraphPad Prism 8 (GraphPad Software, San Diego, CA, USA). Differences among various experimental groups were assessed using one-way ANOVA when the data met normality criteria. Where variances were homogeneous, Tukey’s test was utilized for post-hoc comparisons. Data that did not conform to a normal distribution were analyzed using nonparametric methods. Statistical significance was set at p < 0.05.
Effects of cold exposure on the estrous cycle and reproductive hormone levels
The stages of the estrous cycle were characterized based on the morphological features of vaginal cells: during proestrus, predominantly small, round nucleated epithelial cells were observed; estrus was marked by the presence of leaf-shaped, anucleate keratinized epithelial cells, metestrus was distinguished by a balanced mix of non-nucleated keratinized epithelial cells, leukocytes, and nucleated epithelial cells with minimal variation; diestrus was dominated by a predominance of leukocytes. In the control group, the estrus cycle was regular for 4 to 5 days. In contrast, rats subjected to cold exposure displayed significantly extended and irregular estrous cycles. Treatment with the ERS inhibitor 4-PBA effectively regularized these cycles. Cellular characteristics across these stages are illustrated in Fig. 1A. ELISA assays indicated a significant reduction in the serum concentrations of E2 and P in cold-exposed rats (p < 0.01), while levels in the 4-PBA treated group were notably higher compared to the cold exposure group (p < 0.01). FSH levels did not show significant differences across the groups (p > 0.05), as shown in Fig. 1B.
Figure 1: Effects of cold exposure on the estrous cycle and reproductive hormone levels in rats. (A) Identification of cell characteristics of the estrous cycle. a. Proestrus, b. Estrus, c. Metestrus, d. Diestrus. (B) Serum levels of E2, P, and FSH in rats were determined by ELISA. Compared with the control group, **p < 0.01; compared with the cold exposure group, ##p < 0.01. n = 6.
Effects of cold exposure on morphological changes in the ovary and GCs ultrastructure
Fig. 2A illustrates structurally intact ovaries in the control group, displaying various developmental stages of follicles, the corpus luteum, and some atretic follicles. The GCs in this group were tightly and orderly aligned. In contrast, the cold exposure group exhibited a reduction in follicle numbers across all stages, with primordial and primary follicles being predominant, and GCs arranged sparsely. A significant increase in atretic follicles was noted, characterized by collapsed walls and contracted oocytes. The administration of 4-PBA increased the number of healthy follicles and a decrease in atretic follicles. Fig. 2B reveals that in the control group, the nuclear membranes of the GCs were well-defined and intact, and the ER and mitochondria retained typical morphology. Conversely, in the cold exposure group, there were disruptions in nuclear membranes, ER dilation, absence of distinct cristae in mitochondria, and pronounced mitochondrial vacuolation. Treatment with 4-PBA effectively ameliorated these damages to the ER and mitochondria within GCs.
Figure 2: Effects of cold exposure on morphological changes in the ovary and GCs ultrastructures. (A) Histological morphological assessment of ovarian tissues (scale bar = 200 µm). Yellow arrows indicate atretic follicles. (B) Ultrastructure of the ovarian GCs (scale bar = 1 µm).
Effect of cold exposure on ovarian cells apoptosis
Fig. 3A illustrates a significant increase in the number of apoptotic GCs in the ovaries of the cold exposure group compared to the control. Cold exposure dramatically decreased Bcl-2 protein levels and increased Bax protein levels in GCs, as shown in Fig. 3B. At the gene level, ovarian Bcl-2 mRNA levels were significantly reduced, while Bax mRNA levels were markedly elevated in the cold exposure group, as depicted in Fig. 3C. Additionally, cold exposure decreased ovarian Bcl-2 protein expression and increased Bax protein expression, along with elevated ratios of cleaved caspase-3/pro caspase-3 and cleaved caspase-12/pro caspase-12, as demonstrated in Fig. 3D. Intervention with 4-PBA effectively moderated the increase in apoptosis among GCs, attenuated the reduction of Bcl-2, and mitigated the elevation in Bax, cleaved caspase-3, and cleaved caspase-12 levels.
Figure 3: Effect of cold exposure on ovarian cells apoptosis in rat ovaries. (A) Levels of ovarian GCs apoptosis (scale bar = 100 µm). (B) Expression of Bcl-2 and Bax in GCs (scale bar = 50 µm). (C) Bcl-2 and Bax mRNA expression. (D) The protein levels of Bcl-2, Bax, cleaved caspase-3, and cleaved caspase-12 in the ovaries. Compared with the control group, *p < 0.05, **p < 0.01; compared with the cold exposure group, #p < 0.05, ##p < 0.01. n = 3.
Effects of cold exposure on ERS markers
To explore the underlying mechanisms of cold exposure to ovarian dysfunction, markers of ERS were analyzed. In the cold exposure group, the expression of GRP78 and CHOP in ovarian GCs was found to be elevated (Fig. 4A). Notably, both protein and mRNA levels of GRP78 and CHOP were significantly higher in the ovaries compared to the control group (Fig. 4B,C). Furthermore, the ERS inhibitor 4-PBA effectively reduced the expression levels of GRP78 and CHOP in the ovaries. These findings indicate that cold exposure induces ERS in the ovaries.
Figure 4: Effects of cold exposure on ERS markers in rat ovaries. (A) Expression of GRP78 and CHOP in GCs (scale bar = 50 µm). (B) GRP78 and CHOP mRNA expression. (C) The protein levels of GRP78 and CHOP. Compared with the control group, *p < 0.05, **p < 0.01; compared with the cold exposure group, #p < 0.05, ##p < 0.01. n = 3.
Effects of cold exposure on the PERK/eIF2α/ATF4 pathway
Subsequent investigations explored the role of the PERK/eIF2α/ATF4 pathway in ERS induced by cold exposure. In rats subjected to cold exposure, there was a significant increase in the protein expression of p-PERK, p-eIF2α, and ATF4 in ovarian tissues, as shown in Fig. 5A. Moreover, ATF4 mRNA levels were substantially elevated in the cold exposure group (Fig. 5B). Treatment with 4-PBA effectively reduced the levels of p-PERK, p-eIF2α, and ATF4 in the ovaries of these rats.
Figure 5: Effects of cold exposure on the PERK/eIF2α/ATF4 pathway in rat ovaries. (A) The protein levels of p-PERK, p-eIF2α, and ATF4. (B) ATF4 mRNA expression. Compared with the control group, *p < 0.05, **p < 0.01; compared with the cold exposure group, #p < 0.05, ##p < 0.01. n = 3.
Recent studies have increasingly confirmed the adverse effects of low temperatures on female reproductive functions. Understanding the molecular pathways by which low temperatures induce reproductive harm is crucial for developing preventative and therapeutic strategies against the detrimental effects of hypothermia on female reproductive health. The estrous cycle serves as a critical indicator of female reproductive health, reflecting the dynamic state of ovarian activity and hormone levels [26,27]. In this study, observations from the cold exposure group showed significantly prolonged and irregular estrous cycles, indicating the detrimental effects of sustained intermittent cold exposure on ovarian function. An in-depth analysis was conducted to assess the levels of key reproductive hormones essential for ovarian follicle development. There was a notable decrease in serum concentrations of E2 and P levels following exposure to cold. This hormonal imbalance suggests significant ovarian disruption. Typically, estrogen, primarily secreted by ovarian follicles, sees a reduction in production due to follicular dysplasia. This decrease prompts the pituitary to increase FSH secretion through a negative feedback mechanism, further destabilizing hormonal balance [28]. Cold exposure was found to reduce the prevalence of healthy, normally developing follicles across various stages and increase the incidence of follicular atresia, highlighting the potential of cold stress to impair ovarian functionality. Follicular atresia, characterized by abnormal development and eventual regression of ovarian follicles, critically affects the synthesis of essential reproductive hormones and disrupts normal ovulatory processes, thereby impacting overall reproductive capabilities [29]. Additionally, GCs, integral to the structure of mammalian ovarian follicles, play pivotal roles in the growth and differentiation necessary for healthy follicular development. Notably, the apoptosis of these GCs is a key process contributing to the progression of follicular atresia, influencing the fate of the follicles and the reproductive health of the organism [14–16].
The ER is primarily responsible for protein synthesis, folding, and transport. Under various physiopathological conditions, an accumulation of unfolded and improperly folded proteins within the ER triggers ERS [17]. ERS initiates the UPR, a sophisticated adaptive system designed to maintain protein homeostasis within the cell [17]. This response is orchestrated through three primary regulatory pathways: PERK, inositol-requiring enzyme 1α (IRE1α), and activating transcription factor 6 (ATF6). Under normal conditions, these sensors are bound to the ER-resident protein, GRP78, maintaining them in an inactive state. During ERS, GRP78 detaches from these sensors to bind to misfolded or unfolded proteins, thereby activating the signaling cascades of IRE1α, PERK, and ATF6 aimed at mitigating the burden of these abnormal proteins [30,31]. However, if ERS persists or intensifies, the UPR may transition from a protective to a lethal role by promoting apoptotic processes [18]. Among these pathways, the PERK/eIF2α/ATF4 route is particularly noted for its role in propagating ERS-induced apoptosis. The release of PERK from GRP78 leads to its oligomerization or autophosphorylation, which enhances the phosphorylation of eIF2α. This modification reduces overall protein synthesis, thereby decreasing the protein load within the ER. In cases where ERS becomes unmanageable, phosphorylated eIF2α activates ATF4, which leads to the transcription of apoptotic genes such as CHOP [32]. CHOP is a key trigger and specific transcription factor for ERS-induced apoptosis, capable of regulating various anti-apoptotic and pro-apoptotic genes, including disrupting the balance between anti-apoptotic and pro-apoptotic factors in the Bcl-2 family to trigger apoptosis [33,34]. In instances of prolonged or irreversible ERS, the Bcl-2 protein family is involved in the crosstalk between the ER and mitochondria, playing a crucial role in the initiation of apoptosis [35,36].
Contemporary research indicates that ERS, a critical regulator within the follicular microenvironment, adversely affects follicular health when excessively activated under pathological conditions and significantly contributes to the development of various ovarian pathologies by inducing apoptosis [12]. For example, chronic androgen overload can lead to abnormal apoptosis of GCs by activating ERS, which interferes with normal follicular development and ultimately leads to the occurrence of polycystic ovary syndrome (PCOS) [37]. The application of ERS inhibitors may improve the estrous cycle disorders and reduce follicular atresia associated with PCOS [13]. Similarly, in cases of premature ovarian failure, ERS is critically involved in the aberrant development of follicles, potentially leading to follicular atresia and resultant ovarian dysfunction by inducing apoptosis in GCs [14].
Our observations indicated a significant elevation in GRP78 levels within ovarian tissues following cold exposure. GRP78 acts as a crucial ER-resident chaperone protein that is markedly upregulated upon UPR activation, playing a pivotal role in the initiation phase of ERS signaling and serving as an indicator of ERS [38]. In our experimental model subjected to cold, there was a notable increase in the phosphorylation of PERK and eIF2α, alongside elevated ATF4 protein transcription and mRNA expression, indicative of the activation of the PERK/eIF2α/ATF4 signaling pathway. The activation of the PERK/eIF2α/ATF4 pathway, demonstrated in both in vitro and in vivo contexts, can induce apoptosis in GCs, thereby adversely affecting reproductive functionality [39–42]. Following cold exposure, there was an increase in CHOP transcription within the ovaries, signifying the initiation of ERS-induced apoptotic processes. Further analysis revealed that apoptosis levels in GCs increased significantly after chronic intermittent exposure to cold, accompanied by a decrease in the Bcl-2 and an increase in the Bax. The regulatory interplay between these pro-apoptotic and anti-apoptotic factors, encoded by the Bcl-2 gene family, is crucial in determining the fate of ovarian germ and somatic cells, influencing whether follicles undergo atresia or progress to full maturation and eventual ovulation [43,44]. Elevated expressions of pro-apoptotic proteins BH3-interacting domain death agonist (Bid) and Bax were specifically noted in the GCs of atretic porcine follicles compared to healthy ones [45]. Moreover, activation of caspase-3, a key executor within the caspase family, was detected following cold exposure, emphasizing the induction of apoptosis within rat ovaries. Additionally, the expression of caspase-12, localized to the ER and specifically activated under ERS-induced apoptotic conditions [46], was confirmed. This activation initiates a specific apoptotic cascade involving caspase-12, caspase-9, and caspase-3, occurring independently of cytochrome c, and leading to apoptosis mediated by ERS [47]. The activation of caspase-12 in response to cold confirms that such exposure intricately links to ER-specific stress induction leading to apoptosis.
Our studies have highlighted the critical role of ERS in inducing apoptosis in ovarian cells after exposure to cold environments, with significant mitigation observed upon the administration of 4-PBA. It is well-established that chaperone proteins, essential for cellular homeostasis, operate by binding to unfolded or misfolded protein chains, thus preventing their improper aggregation and assisting in proper folding [48]. This function has spurred the development of chemical chaperones, synthetic analogs of chaperonins, which are increasingly recognized for their potential in treating pathologies associated with ERS. Among these, 4-PBA stabilizes protein folding and prevents aberrant protein aggregation, thereby alleviating ERS, improving the function of the ER, and decreasing apoptosis triggered by the UPR [49]. Extensive research has demonstrated that 4-PBA not only alleviates the adverse effects of ERS on ovarian cells but also significantly improves ovarian health by restoring normal cellular functions and reducing apoptotic activity [22,23].
While recent research has predominantly focused on the deleterious effects of heat stress on ovarian functionality, as evidenced by References [50,51], our study delves into the relatively unexplored area of cold-induced ovarian dysfunction. Previous findings suggest that local exposure to cold water in women is associated with an increased risk of gynecological diseases [52]. Therefore, we chose ice water immersion to simulate cold exposure. We present novel evidence suggesting that cold conditions contribute to ovarian impairment through ERS-mediated apoptosis. This study appears to be the first exploration of how cold exposure disrupts ovarian function via this specific apoptotic pathway.
In conclusion, our findings reveal that exposure to cold triggers ERS and activates the PERK pathway, subsequently enhancing apoptosis levels, as illustrated in Fig. 6. Mitigating ERS proves to be an effective strategy for alleviating ovarian apoptosis and enhancing ovarian health. The link between cold temperatures and ovarian dysfunction appears to involve ERS and apoptotic processes, particularly within GCs. These insights could offer novel strategies for protecting ovarian health against the detrimental effects of cold environments. This research also serves as a reminder that since cold exposure can lead to ovarian damage, prolonged exposure to the cold should be avoided.
Figure 6: A schematic mechanism by which cold exposure-induced ERS-induced apoptosis damages ovarian function.
This investigation acknowledges certain limitations. The exploration of mechanisms underlying cold-induced ovarian dysfunction has been confined to animal models, which necessitates further examination at the cellular level. Regarding the inhibition of ERS, 4-PBA was selected due to its recognized efficacy. However, the dual role of 4-PBA as both an ERS inhibitor and a histone deacetylase inhibitor introduces uncertainties regarding the effects specifically attributable to its latter function. In future research, our direction will focus on identifying more specific inhibitors of ERS, or selectively targeting key factors involved in ERS to further investigate the mechanisms by which ERS impairs ovarian function upon cold exposure.
Acknowledgement: None.
Funding Statement: This study was supported by the National Natural Science Foundation of China (Nos. 82174426, 82104740), the Natural Science Foundation of Hebei Province of China (No. H2021423020) and the Innovation Funding Program for doctoral students in Hebei Province (No. CXZZBS2022098).
Author Contributions: The authors confirm their contribution to the paper as follows: study conception and design: Xiumei Cheng, Di Wang and Yanqing Ren. Data collection: Xiaodan Song and Yuqing Wang. Analysis and interpretation of results: Xiaodan Song, Xinhua Li, Xiaoqian Di, Sisi Xue and Tianyuan Lv. Draft manuscript preparation: Xiaodan Song. Reviewed and edited the manuscript: Xiumei Cheng and Xiaoyun Zhang. All authors reviewed the results and approved the final version of the manuscript.
Availability of Data and Materials: The datasets generated and/or analyzed during the current study are available from the corresponding author on reasonable request.
Ethics Approval: The experimental protocol received approval from the Ethics Committee of Hebei University of Chinese Medicine (DWLL 2021025). All animals were treated humanely.
Conflicts of Interest: The authors declare that the research was conducted in the absence of any commercial or financial relationships that could be construed as a potential conflict of interest.
References
1. Gasparrini A, Guo Y, Hashizume M, Lavigne E, Zanobetti A, Schwartz J, et al. Mortality risk attributable to high and low ambient temperature: a multicountry observational study. Lancet. 2015;386(9991):369–75. doi:10.1016/S0140-6736(14)62114-0. [Google Scholar] [PubMed] [CrossRef]
2. Zhao Q, Guo Y, Ye T, Gasparrini A, Tong S, Overcenco A, et al. Global, regional, and national burden of mortality associated with non-optimal ambient temperatures from 2000 to 2019: a three-stage modelling study. Lancet Planet Health. 2021;5(7):e415–25. doi:10.1016/S2542-5196(21)00081-4. [Google Scholar] [PubMed] [CrossRef]
3. Hyrkäs-Palmu H, Jaakkola MS, Mäkikyrö EMS, Jaakkola JJK. Subtypes of asthma and cold weather-related respiratory symptoms. Int J Environ Res Public Health. 2022;19(14):8790. doi:10.3390/ijerph19148790. [Google Scholar] [PubMed] [CrossRef]
4. Phung D, Thai PK, Guo Y, Morawska L, Rutherford S, Chu C. Ambient temperature and risk of cardiovascular hospitalization: an updated systematic review and meta-analysis. Sci Total Environ. 2016;550:1084–102. doi:10.1016/j.scitotenv.2016.01.154. [Google Scholar] [PubMed] [CrossRef]
5. Nabenishi H, Yamazaki A. Impaired reproduction in Japanese black cattle under cold environmental conditions. Reprod Domest Anim. 2017;52(3):371–5. doi:10.1111/rda.12917. [Google Scholar] [PubMed] [CrossRef]
6. Messing K, Saurel-Cubizolles MJ, Bourgine M, Kaminski M. Menstrual-cycle characteristics and work conditions of workers in poultry slaughterhouses and canneries. Scand J Work Environ Health. 1992;18(5):302–9. doi:10.5271/sjweh.1572. [Google Scholar] [PubMed] [CrossRef]
7. Retana-Márquez S, Juárez-Rojas L, Ávila-Quintero A, Rojas-Maya S, Perera G, Casillas F, et al. Neuroendocrine disruption is associated to infertility in chronically stressed female rats. Reprod Biol. 2020;20(4):474–83. doi:10.1016/j.repbio.2020.07.011. [Google Scholar] [PubMed] [CrossRef]
8. Belay T, Woart A, Graffeo V. Effect of cold water-induced stress on immune response, pathology and fertility in mice during Chlamydia muridarum genital infection. Pathog Dis. 2017;75(5):ftx045. doi:10.1093/femspd/ftx045. [Google Scholar] [PubMed] [CrossRef]
9. Wang D, Cheng X, Fang H, Ren Y, Li X, Ren W, et al. Effects of modified Wenjing decoction on microcirculation in reproductive organs in rats with symptom patterns of cold coagulation and blood stasis. J Tradit Chin Med. 2020;40(2):212–23. doi:10.19852/j.cnki.jtcm.2020.02.005. [Google Scholar] [CrossRef]
10. Wang D, Cheng X, Fang H, Ren Y, Li X, Ren W, et al. Effect of cold stress on ovarian & uterine microcirculation in rats and the role of endothelin system. Reprod Biol Endocrinol. 2020;18(1):29. doi:10.1186/s12958-020-00584-1. [Google Scholar] [PubMed] [CrossRef]
11. Fan X, Wang J, Ma Y, Chai D, Han S, Xiao C, et al. Activation of P2X7 receptor mediates the abnormal ovulation induced by chronic restraint stress and chronic cold stress. Biology. 2024;13(8):620. doi:10.3390/biology13080620. [Google Scholar] [PubMed] [CrossRef]
12. Harada M, Takahashi N, Azhary JM, Kunitomi C, Fujii T, Osuga Y. Endoplasmic reticulum stress: a key regulator of the follicular microenvironment in the ovary. Mol Hum Reprod. 2021;27(1):gaaa088. doi:10.1093/molehr/gaaa088. [Google Scholar] [PubMed] [CrossRef]
13. Azhary JMK, Harada M, Kunitomi C, Kusamoto A, Takahashi N, Nose E, et al. Androgens increase accumulation of advanced glycation end products in granulosa cells by activating ERS in PCOS. Endocrinology. 2020;161(2):bqaa015. doi:10.1210/endocr/bqaa015. [Google Scholar] [PubMed] [CrossRef]
14. Tang X, Dong H, Fang Z, Li J, Yang Q, Yao T, et al. Ubiquitin-like modifier 1 ligating enzyme 1 relieves cisplatin-induced premature ovarian failure by reducing endoplasmic reticulum stress in granulosa cells. Reprod Biol Endocrinol. 2022;20(1):84. doi:10.1186/s12958-022-00956-9. [Google Scholar] [PubMed] [CrossRef]
15. Liu W, Chen Q, Liu Z, Weng Z, Nguyen TN, Feng J, et al. Zihuai recipe alleviates cyclophosphamide-induced diminished ovarian reserve via suppressing PI3K/AKT-mediated apoptosis. J Ethnopharmacol. 2021;277:113789. doi:10.1016/j.jep.2021.113789. [Google Scholar] [PubMed] [CrossRef]
16. Zhang Y, Weng Y, Wang D, Wang R, Wang L, Zhou J, et al. Curcumin in combination with aerobic exercise improves follicular dysfunction via inhibition of the hyperandrogen-induced IRE1α/XBP1 endoplasmic reticulum stress pathway in PCOS-like rats. Oxid Med Cell Longev. 2021;2021(1):7382900. doi:10.1155/2021/7382900. [Google Scholar] [PubMed] [CrossRef]
17. Marciniak SJ, Chambers JE, Ron D. Pharmacological targeting of endoplasmic reticulum stress in disease. Nat Rev Drug Discov. 2022;21(2):115–40. doi:10.1038/s41573-021-00320-3. [Google Scholar] [PubMed] [CrossRef]
18. Sims SG, Cisney RN, Lipscomb MM, Meares GP. The role of endoplasmic reticulum stress in astrocytes. Glia. 2022;70(1):5–19. doi:10.1002/glia.24082. [Google Scholar] [PubMed] [CrossRef]
19. Guo J, Hu H, Chen Z, Xu J, Nie J, Lu J, et al. Cold exposure induces intestinal barrier damage and endoplasmic reticulum stress in the colon via the SIRT1/Nrf2 signaling pathway. Front Physiol. 2022;13:822348. doi:10.3389/fphys.2022.822348. [Google Scholar] [PubMed] [CrossRef]
20. Teng T, Zheng Y, Zhang M, Sun G, Li Z, Shi B, et al. Chronic cold stress promotes inflammation and ER stress via inhibiting GLP-1R signaling, and exacerbates the risk of ferroptosis in the liver and pancreas. Environ Pollut. 2024;360(2):124647. doi:10.1016/j.envpol.2024.124647. [Google Scholar] [PubMed] [CrossRef]
21. Zhou X, Zou L, Deng H, Zhou Y, Wu Y, Ouyang X, et al. Protective effects and mechanisms of inhibiting endoplasmic reticulum stress on cold seawater immersion combined with hemorrhagic shock. J Inflamm Res. 2024;17:4923–40. doi:10.2147/JIR.S469622. [Google Scholar] [PubMed] [CrossRef]
22. Wu Y, Ma C, Zhao H, Zhou Y, Chen Z, Wang L. Alleviation of endoplasmic reticulum stress protects against cisplatin-induced ovarian damage. Reprod Biol Endocrinol. 2018;16(1):85. doi:10.1186/s12958-018-0404-4. [Google Scholar] [PubMed] [CrossRef]
23. Cong J, Zhang Y, Yang X, Wang Y, He H, Wang M. Anti-polycystic ovary syndrome effect of electroacupuncture: IMD inhibits ER stress-mediated apoptosis and autophagy in granulosa cells. Biochem Biophys Res Commun. 2022;634:159–67. doi:10.1016/j.bbrc.2022.10.030. [Google Scholar] [PubMed] [CrossRef]
24. Cheng X, Du H, Li D. Establishment of syndrome model of blood stasis due to cold accumulation in rats. J Basic Chin Med. 2005;8:604–5 (In Chinese). [Google Scholar]
25. Zhou Q, He M, Jin Q, Gao S, Yang Z, Zhu P, et al. Mechanism of action of Taohong Siwu decoction in the alleviation of primary dysmenorrhea. Front Med. 2024;11:1343179. doi:10.3389/fmed.2024.1343179. [Google Scholar] [PubMed] [CrossRef]
26. Yin Y, Li H, Qin Y, Chen T, Zhang Z, Lu G, et al. Moxibustion mitigates mitochondrial dysfunction and NLRP3 inflammatory activation in cyclophosphamide-induced premature ovarian insufficiency rats. Life Sci. 2023;314:121283. doi:10.1016/j.lfs.2022.121283. [Google Scholar] [PubMed] [CrossRef]
27. Yu Z, Wang F, Han J, Lu R, Li Q, Cai L, et al. Opposite effects of high- and low-dose di-(2-ethylhexyl) phthalate (DEHP) exposure on puberty onset, oestrous cycle regularity and hypothalamic kisspeptin expression in female rats. Reprod Fertil Dev. 2020;32(6):610–8. doi:10.1071/RD19024. [Google Scholar] [PubMed] [CrossRef]
28. Chen Y, Sun Y, Zhao A, Cai X, Yu A, Xu Q, et al. Arsenic exposure diminishes ovarian follicular reserve and induces abnormal steroidogenesis by DNA methylation. Ecotoxicol Environ Saf. 2022;241(Pt 3):113816. doi:10.1016/j.ecoenv.2022.113816. [Google Scholar] [PubMed] [CrossRef]
29. Yang F, Chen Y, Liu Q, Dai S, Zeng S. Dynamics and regulations of BimEL Ser65 and Thr112 phosphorylation in porcine granulosa cells during follicular atresia. Cells. 2020;9(2):402. doi:10.3390/cells9020402. [Google Scholar] [PubMed] [CrossRef]
30. Hetz C, Zhang K, Kaufman RJ. Mechanisms, regulation and functions of the unfolded protein response. Nat Rev Mol Cell Biol. 2020;21(8):421–38. doi:10.1038/s41580-020-0250-z. [Google Scholar] [PubMed] [CrossRef]
31. Shreya S, Grosset CF, Jain BP. Unfolded protein response signaling in liver disorders: a 2023 updated review. Int J Mol Sci. 2023;24(18):14066. doi:10.3390/ijms241814066. [Google Scholar] [PubMed] [CrossRef]
32. da Silva DC, Valentão P, Andrade PB, Pereira DM. Endoplasmic reticulum stress signaling in cancer and neurodegenerative disorders: tools and strategies to understand its complexity. Pharmacol Res. 2020;155(1):104702. doi:10.1016/j.phrs.2020.104702. [Google Scholar] [PubMed] [CrossRef]
33. Hu H, Tian M, Ding C, Yu S. The C/EBP homologous protein (CHOP) transcription factor functions in endoplasmic reticulum stress-induced apoptosis and microbial infection. Front Immunol. 2019;9:3083. doi:10.3389/fimmu.2018.03083. [Google Scholar] [PubMed] [CrossRef]
34. Liu H, Tian Z, Guo Y, Liu X, Ma Y, Du X, et al. Microcystin-leucine arginine exposure contributes to apoptosis and follicular atresia in mice ovaries by endoplasmic reticulum stress-upregulated Ddit3. Sci Total Environ. 2021;756:144070. doi:10.1016/j.scitotenv.2020.144070. [Google Scholar] [PubMed] [CrossRef]
35. Rodriguez D, Rojas-Rivera D, Hetz C. Integrating stress signals at the endoplasmic reticulum: the BCL-2 protein family rheostat. Biochim Biophys Acta. 2011;1813(4):564–74. doi:10.1016/j.bbamcr.2010.11.012. [Google Scholar] [PubMed] [CrossRef]
36. Hetz C. The unfolded protein response: controlling cell fate decisions under ERS and beyond. Nat Rev Mol Cell Biol. 2012;13(2):89–102. doi:10.1038/nrm3270. [Google Scholar] [PubMed] [CrossRef]
37. Wang C, Zhang Y. Endoplasmic reticulum stress: a new research direction for polycystic ovary syndrome? DNA Cell Biol. 2022;41(4):356–67. doi:10.1089/dna.2021.1050. [Google Scholar] [PubMed] [CrossRef]
38. Schönthal AH. Endoplasmic reticulum stress: its role in disease and novel prospects for therapy. Scientifica. 2012;2012(4–6):857516–26. doi:10.6064/2012/857516. [Google Scholar] [PubMed] [CrossRef]
39. Pan X, Liu Y, Liu L, Pang B, Sun Z, Guan S, et al. Bushen Jieyu Tiaochong formula reduces apoptosis of granulosa cells via the PERK-ATF4-CHOP signaling pathway in a rat model of polycystic ovary syndrome with chronic stress. J Ethnopharmacol. 2022;292:114923. doi:10.1016/j.jep.2021.114923. [Google Scholar] [PubMed] [CrossRef]
40. Li X, Lin Y, Yao J, Pan B, Zhan X, Chen Z, et al. Protegrin-1 inhibits porcine ovarian granulosa cell apoptosis from H2O2-induced oxidative stress via the PERK/eIF2α/CHOP signaling pathway in vitro. Theriogenology. 2022;179(Suppl 4):117–27. doi:10.1016/j.theriogenology.2021.11.022. [Google Scholar] [PubMed] [CrossRef]
41. Luo Y, Guo Q, Zhang L, Zhuan Q, Meng L, Fu X, et al. Dihydroartemisinin exposure impairs porcine ovarian granulosa cells by activating PERK-eIF2α-ATF4 through endoplasmic reticulum stress. Toxicol Appl Pharmacol. 2020;403:115159. doi:10.1016/j.taap.2020.115159. [Google Scholar] [PubMed] [CrossRef]
42. Wang W, Guan J, Feng Y, Liu S, Zhao Y, Xu Y, et al. Polystyrene microplastics induced ovarian toxicity in juvenile rats associated with oxidative stress and activation of the PERK-eIF2α-ATF4-CHOP signaling pathway. Toxics. 2023;11(3):225. doi:10.3390/toxics11030225. [Google Scholar] [PubMed] [CrossRef]
43. Zhang G, Wan Y, Zhang Y, Lan S, Jia R, Wang Z, et al. Expression of mitochondria-associated genes (PPARGC1A, NRF-1, BCL-2 and BAX) in follicular development and atresia of goat ovaries. Reprod Domest Anim. 2015;50(3):465–73. doi:10.1111/rda.12514. [Google Scholar] [PubMed] [CrossRef]
44. Rucker EB 3rd, Dierisseau P, Wagner KU, Garrett L, Wynshaw-Boris A, Flaws JA, et al. Bcl-x and Bax regulate mouse primordial germ cell survival and apoptosis during embryogenesis. Mol Endocrinol. 2000;14(7):1038–52. doi:10.1210/mend.14.7.0465. [Google Scholar] [PubMed] [CrossRef]
45. Sai T, Goto Y, Yoshioka R, Maeda A, Matsuda F, Sugimoto M, et al. Bid and Bax are involved in granulosa cell apoptosis during follicular atresia in porcine ovaries. J Reprod Dev. 2011;57(3):421–7. doi:10.1262/jrd.11-007H. [Google Scholar] [PubMed] [CrossRef]
46. Nakagawa T, Zhu H, Morishima N, Li E, Xu J, Yankner BA, et al. Caspase-12 mediates endoplasmic-reticulum-specific apoptosis and cytotoxicity by amyloid-β. Nature. 2000;403(6765):98–103. doi:10.1038/47513. [Google Scholar] [PubMed] [CrossRef]
47. Liu YN, Mu YD, Wang H, Zhang M, Shi YW, Mi G, et al. Endoplasmic reticulum stress pathway mediates T-2 toxin-induced chondrocyte apoptosis. Toxicology. 2000;464:152989. doi:10.1016/j.tox.2021.152989. [Google Scholar] [PubMed] [CrossRef]
48. Jeon JH, Im S, Kim HS, Lee D, Jeong K, Ku JM, et al. Chemical chaperones to inhibit endoplasmic reticulum stress: implications in diseases. Drug Des Devel Ther. 2022;16:4385–97. doi:10.2147/DDDT.S393816. [Google Scholar] [PubMed] [CrossRef]
49. Kolb PS, Ayaub EA, Zhou W, Yum V, Dickhout JG, Ask K. The therapeutic effects of 4-phenylbutyric acid in maintaining proteostasis. Int J Biochem Cell Biol. 2015;61(1):45–52. doi:10.1016/j.biocel.2015.01.015. [Google Scholar] [PubMed] [CrossRef]
50. Xiong Y, Yin Q, Jin E, Chen H, He S. Selenium attenuates chronic heat stress-induced apoptosis via the inhibition of endoplasmic reticulum stress in mouse granulosa cells. Molecules. 2020;25(3):557. doi:10.3390/molecules25030557. [Google Scholar] [PubMed] [CrossRef]
51. Xiong Y, Jin E, Yin Q, Che C, He S. Boron attenuates heat stress-induced apoptosis by inhibiting endoplasmic reticulum stress in mouse granulosa cells. Biol Trace Elem Res. 2021;199(2):611–21. doi:10.1007/s12011-020-02180-1. [Google Scholar] [PubMed] [CrossRef]
52. Du H, Chai L, Ha L, Wu G, Sha M, Jin J. An initial exploration of the pathological mechanism of cold coagulation and blood stasis syndrome in menstrual disorders. J Tradit Chin Med. 1993;34(7):428–30 (In Chinese). doi:10.13288/j.11-2166/r.1993.07.025. [Google Scholar] [CrossRef]
Cite This Article
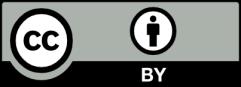
This work is licensed under a Creative Commons Attribution 4.0 International License , which permits unrestricted use, distribution, and reproduction in any medium, provided the original work is properly cited.