Open Access
REVIEW
Impact of nanoparticles on immune cells and their potential applications in cancer immunotherapy
1 Leibniz Institute of Photonic Technology, Albert-Einstein-Straße 9, Jena, 07745, Germany
2 Serah Zines LLP, Sreeragam, Naduvannor P.O. Kozhikkode, Kerala, 673614, India
3 Department of Chemistry, Newman College, Thodupuzha, Kerala, 685585, India
4 Department of Chemistry, Madanapalle Institute of Technology & Science (MITS), Madanapalle, Chittoor, Andhra Pradesh, 517325, India
5 Department of Life Sciences, CHRIST University, Bengaluru, Karnataka, 560029, India
* Corresponding Author: MANU M. JOSEPH. Email:
(This article belongs to the Special Issue: Genetic Biomarkers of Cancer: Insights into Molecular and Cellular Mechanisms)
BIOCELL 2024, 48(11), 1579-1602. https://doi.org/10.32604/biocell.2024.054879
Received 10 June 2024; Accepted 03 September 2024; Issue published 07 November 2024
Abstract
Nanoparticles represent a heterogeneous collection of materials, whether natural or synthetic, with dimensions aligning in the nanoscale. Because of their intense manifestation with the immune system, they can be harvested for numerous bio-medical and biotechnological advancements mainly in cancer treatment. This review article aims to scrutinize various types of nanoparticles that interact differently with immune cells like macrophages, dendritic cells, T lymphocytes, and natural killer (NK) cells. It also underscores the importance of knowing how nanoparticles influence immune cell functions, such as the production of cytokines and the presentation of antigens which are crucial for effective cancer immunotherapy. Hence overviews of bio-molecular mechanisms are provided. Nanoparticles can improve antigen presentation, boost T-cell responses, and overcome the immunosuppressive tumor environment. The regulatory mechanisms, signaling pathways, and nanoparticle characteristics are also presented for a comprehensive understanding. We review the nanotechnology platform options and challenges in nanoparticles-based immunotherapy, from an immunotherapy perspective including precise targeting, immune modulation, and potential toxicity, as well as personalized approaches based on individual patient and tumor characteristics. The development of emerging multifunctional nanoparticles and theranostic nanoparticles will provide new solutions for the precision and efficiency of cancer therapies in next-generation practice.Keywords
Nanoparticles represent a collection of materials either natural or artificial with the dimensions aligning in the nanoscale. They strongly interrelate with the immune system in multiple ways by impacting the behavior of immune cells and presenting the potential for diverse medical applications, particularly in the overall management of cancer. This review article aims to examine how various nanoparticles, including liposomes and metallic ones, affect immune cells such as macrophages, dendritic cells, T lymphocytes, and natural killer (NK) cells. Here we also aim to showcase the importance of understanding how nanoparticles influence immune cell functions like modulating cytokine production, antigen presentation, infiltration of immune cells into cancerous tissues, and much more. In the case of cancer immunotherapy, nanoparticles play a very crucial role by improving antigen presentation, boosting T-cell responses, and overcoming the immunosuppressive tumor environment. In this line, it is equally essential to explore regulatory mechanisms, signaling pathways, and nanoparticle characteristics such as size, shape, and surface charge for a comprehensive understanding [1,2].
Brief overview of cancer immunotherapy
Cancer immunotherapy was presented as a tactical tool to make use of the defense mechanism of the body to overcome the resistance offered by the invading cells. Hence it represents a groundbreaking shift from conventional treatments, connecting the body’s immune system to selectively detect and eliminate cancer cells with greater precision and accuracy compared to chemotherapy and radiation. Checkpoint inhibitors including Programmed Cell Death Protein 1 (PD-1) and Cytotoxic T-Lymphocyte Associated Protein 4 (CTLA-4) disrupt molecular checkpoints, empowering the immune system to mount a stronger anti-tumor response [3]. Adoptive cell transfer involves enhancing T cells externally before reintroduction, intensifying immune responses. Therapeutic vaccines excite the immune system to identify and combat cancer cells using tumour-specific antigens or immune-boosting agents for sustained effectiveness. This comprehensive approach signifies a fundamental shift in cancer treatment [4]. Despite notable successes, challenges persist, including resistance development and immune-related adverse effects. Achieving a delicate immune response modulation balance is crucial. Ongoing research, clinical trials, exploration of combination therapies, quest for new biomarkers, and new targets aim to enhance and expand the applications of cancer immunotherapy. This evolving landscape hence holds promise for more effective, targeted, and personalized interventions, marking a new era in cancer management [5].
Cancer immunotherapy uses the inherent power of the body’s immune system in fighting cancer [6]. The prevalent approaches for this type of treatment include immune checkpoint inhibitors, CAR T-cell therapy, cancer vaccines, and monoclonal antibodies. Checkpoint inhibitors target proteins PD-1 and CTLA-4, such as pembrolizumab and nivolumab respectively can alter the immune system response and are utilized to enhance the immune activation to fight cancer. CAR T-cell therapy involves introducing epitope-specific T-cells in a patient, which attack cancer cells more precisely [7]. Mechanistically, cancer vaccines work by training the immune system to identify and destroy the cells of a cancer; monoclonal antibodies bind to targets on cancer, marking the cells for immune destruction. Clinical advancements in this area are focused on increasing the effectiveness by reducing side effects and treating a larger number of cancers with complex etiology. Combination therapies, in which multiple immunotherapies are used or combined with traditional treatments, have been very effective. Personalizing immunotherapy based on a patient’s tumor genetics and immune profile holds immense promise. Also, nano-enabled approaches deliver precisely to the tumor, enhancing precision and reducing toxicity tremendously. New strategies in fighting immunotherapy resistance include shifting the balance of the tumor microenvironment to make tumours more susceptible to immune attack. This includes strategies against specific immune cells or cytokines involved in favoring immune suppression. Oncolytic virus therapy is the use of weakened viruses to kill cancer cells and stimulate an immune response [8,9]. Research is also oriented toward the development of bispecific T-cell engagers that bring T-cells closer to cancer cells for strong and effective immune attacks. Artificial intelligence and machine learning can be integrated in predicting responses by patients, identifying new targets, and failed optimization of clinical trials [10].
The key predictors of response to cancer immunotherapy include biomarkers, genetic and molecular factors, and emerging indicators that as a whole help in explaining and evaluating the patient’s responses. This will aid personalized therapy opportunities in most cases [11,12]. Probably the most important biomarkers are the levels of PD-L1 expression, which give an idea about response prediction to PD-1/PD-L1 inhibitors, and tumor mutational burden (TMB), where higher TMB generally goes with better outcomes due to increased neoantigen presentation [13]. Equally important are microsatellite instability (MSI) and mismatch repair deficiency (dMMR). High MSI/dMMR tumors exhibited better responses to checkpoint inhibitors. Genetic factors also include gene-specific mutations, such as those in Epidermal Growth Factor Receptor (EGFR), Kirsten Rat Sarcoma Viral Oncogene Homolog (KRAS), and v-Raf murine sarcoma viral oncogene homolog B (BRAF), or oncogenic viruses like Human Papillomavirus (HPV) and Epstein-Barr Virus (EBV), which modulate the efficacy of immunotherapy. The constitution of this tumor microenvironment; density and type of infiltrating immune cells, including TILs, macrophages, and regulatory T cells are also important. Some of the upcoming predictors include the gut microbiome, which is being shown to have a role in modulating the responses to immunotherapy and novel biomarkers like circulating tumor DNA together with specific gene expression profiles [14]. Advanced methodologies, concerning comprehensive genomic profiling and single-cell RNA sequencing, have been expanding this space of predictors’ identification and validation. These predictors, at a later stage, will help guide personalized immunotherapy approaches to finally improve patient outcomes, especially in complex pathological abnormalities including rare types of cancer and autoimmune diseases [15].
Approaches to enhance the effectiveness of immunotherapy
Refining immunotherapy involves employing diverse strategies beyond conventional approaches. Combination therapies, which blend various agents with standard treatments, seek to enhance anti-cancer responses by utilizing complementary mechanisms and addressing resistance issues. Precision medicine tailors immunotherapies to individual patients by considering their genetic, molecular, and immune system characteristics, aided by biomarker identification [16]. Several key strategies must be employed to overcome the suppressive tumor microenvironment. Mainly, it demands targeting inhibitory factors and normalizing tumor blood vessels to enhance immune cell infiltration. It is a good approach to explore the potential of the gut microbiome through interventions such as faecal microbiota transplantation or probiotics to enhance systemic immune responses and improve immunotherapy outcomes. Technological improvements, especially artificial intelligence (AI), are transforming cancer treatment by analyzing massive datasets using machine learning algorithms to unearth complicated biological interactions and identify new immunotherapy targets. Next-generation immunotherapies, including oncolytic viruses and genetically modified immune cells, are broadening the arsenal against cancer by optimizing immune cells’ specificity and potency through genetic engineering, enhancing cancer cell recognition and elimination (Fig. 1). It is to be noted that much ongoing research aims to thoroughly comprehend the dynamic interplay between the immune system and cancer, leading to strategies that bolster immunotherapy effectiveness, surmount challenges, enhance patient responses, and establish immunotherapy as a fundamental pillar of cancer treatment [17,18].
Figure 1: Schematic representation of various approaches used in cancer immunotherapy; that can affect the patient’s immune system. Some of the methods include enhancing the inbuilt immune response towards cancer, and another method initiates a new immune system against the disease, others use cancer-fighting immune elements in the body, created with BioRender.com, accessed on 21 July 2024.
Nanoparticles as potential tools in cancer immunotherapy
Nanoparticles offer promising prospects in cancer immunotherapy by exploiting unique features to improve treatment effectiveness. Their diverse characteristics, including size, surface charge, and compatibility with the body, enable precise delivery of therapeutic substances to cancerous cells, reducing overall side effects. Notably, nanoparticles can effectively encapsulate, transport and precisely deliver immunomodulatory compounds like cytokines or immune checkpoint inhibitors directly to tumor sites. This can modulate the overall response of tumor microenvironment and activate immune cells. Additionally, nanoparticles can serve as adjuvants, stimulating immune responses by engaging with immune cells, particularly those involved in presenting antigens [19,20]. This function is essential in the development of cancer vaccines, where nanoparticles aid in presenting tumor-specific antigens, fostering a potent and targeted anti-cancer immune response [21]. Nanoparticles offer solutions to multiple challenges in cancer immunotherapy. Engineered nanoparticles can modify regulatory T cells and suppressor cells in the tumor microenvironment, creating a conducive setting for immune cells to target cancer. Overcoming drug resistance is possible by co-delivering multiple agents through nanoparticles with distinct mechanisms. Additionally, certain nanoparticles aid in the real-time monitoring of treatment responses, crucial for assessing immunotherapy effectiveness and tailoring strategies based on patient responses [22,23]. Combining nanoparticles with imaging provides insights into immune response dynamics, aiding informed treatment decisions. Despite great promise, challenges like potential toxicity require thorough investigation. Ongoing research aims to refine nanoparticle formulations, optimize properties, and understand interactions within the biological environment, positioning nanoparticles to transform cancer immunotherapy with more effective and targeted strategies (Fig. 2).
Figure 2: Schematic representation of modifications in nanoparticles for effective cancer immunotherapy.
Tuning the physical properties of nanomaterials for enhanced cancer immunotherapy
The size of the particle, synthetically tuned shape, surface charge tuning, chemical and mechanical strength, and affinity towards water are just a few examples of physical features of nanoparticles that can alter immune cell functions and mutate immune responses in both healthy and diseased states. Thus, the distinct physicochemical properties of nanoparticles can be tuned for immuno-bioengineering applications to accomplish targeted immunological effects. The physiochemical parameters of the synthesized nanoparticles can meticulously change how protein adsorption, immune scavenging property, biodistribution, immune cell targeting, and toxicity. We describe the problems in nanomaterial-based immune engineering that need to be solved to enable clinical translation and emphasize the breakthroughs in nanoengineering that have made it possible to tune the physical properties of nanoparticles for applications in cancer immune engineering.
Certain immune cell subtypes, including dendritic cells, macrophages, T cells, natural killer cells, and B cells, have their immune signaling pathways and effects or functions transformed by physical parameters. For instance, the way that nanoparticles interact with immune cells and trigger an immunological response can be affected by their size, shape, and surface charge. Researchers can create nanoparticles that boost immune cell function and increase their capacity to target and kill cancer cells by carefully manipulating these physical characteristics. This degree of control opens the door for future novel cancer immunotherapies and is essential to the success of immuno-engineering. The physiochemical properties such as toxicity, bio-distribution, pharmacokinetics, and targeted immune cell targeting are influenced by changes in their physical characteristics. Engineering nanoparticle properties, directly or indirectly facilitate the activation of antitumor immune signaling pathways and effects or functions in distinct immune cell subtypes. The physical engineering of nanomaterials provides an unprecedented way to implement efficient cancer immunotherapy. For example, modifying the size and shape of gold nanoparticles can enhance their uptake by dendritic cells, leading to improved antigen presentation and T-cell activation [20]. Additionally, surface modifications with antibodies targeting specific immune cell receptors can direct the nanomaterials to interact with desired immune cells, further enhancing their therapeutic potential in cancer immunotherapy.
Nanoparticles and Immune Cells
Exploring the dynamic interplay between nanoparticles and immune cells unlocks many innovative applications in biomedicine. Nanoparticles interact intricately with different components of the immune system either passively or actively. This interaction has far-reaching implications, influencing immune cell behavior and responses. Understanding these complex biomolecular interactions is of much importance because it can have greater impacts, particularly in the context of cancer immunotherapy. From liposomes to polymeric and metallic nanoparticles, each variant presents unique opportunities for modulating immune cell activity. This study investigates the mechanisms by which nanoparticles engage with immune cells, providing a foundation for harnessing their potential in advancing medical interventions [24].
Nanoparticles commonly used in cancer immunotherapy
Nanoparticles come in a wide range of sizes, shapes, surface charges, and functionalization, all of which eventually affect their interactions with immune cells like macrophages, dendritic cells, T lymphocytes, and NK cells (Fig. 3). Size here becomes a very critical factor. Usually, smaller nanoparticles (1–100 nm) may have better cellular uptake and deeper tissue penetration [25]. In contrast, larger-sized nanoparticles may get taken up more efficiently by phagocytic cells like macrophages [26]. Equally important is the shape, as spherical nanoparticles normally have homogeneous distribution and cellular uptake, while rod-shaped or filamentous particles may avoid phagocytosis and provide a longer circulation time. The surface charge affects the interaction of a nanoparticle with the cell membrane. Generally, positively charged nanoparticles exhibit higher uptake resulting from electrostatic attraction to the cell membranes, which are negatively charged, but it tends to cause higher cytotoxicity. Surface functionalization of the nanoparticle with specific ligands or coatings, like Polyethylene Glycol (PEG), antibodies, or peptides, enhances targeting and decreases immunogenicity at a high level, providing improved biocompatibility and efficacy. Lastly, immune cells can interact with nanoparticles in the following ways: macrophages-good at phagocytosing and processing nanoparticles, thus leading to inflammatory responses; dendritic cells-as an Antigen-Presenting Cell (APC), nanoparticles can be uptaken up to increase immune activation; functionalized nanoparticles targeting or activating T lymphocytes to alter immune responses; and finally, NK cells may be influenced by nanoparticles to enhance their cytotoxic activity against tumors [27].
Figure 3: Schematic illustration of the diverse types of nanoparticles, highlighting various possibilities for modifications, including different shapes, surface functionalization, and other potential alterations, created with BioRender.com.
Nanoparticles can be designed in such a way that they should transport antigens directly to APCs, which, therefore, will make the quality of antigen presentation more effective and accurate. Selective orientation like this facilitates strong T-cell activation, which, in turn, paves the way for a more ground-breaking and precise response that destroys tumor cells more effectively. Besides, nanoparticles are established to regulate the production of cytokines, the messenger molecules that lead immune responses to be activated or terminated. For example, nanoparticles are engineered to deliver genes or small molecules that trigger the production of pro-inflammatory cytokines, which in turn increases the ability of the immune system to distinguish and then get rid of cancer cells [28]. Besides that, the nanoparticles can also deliver the agents that will counteract cancer cells’ immunosuppressive effect. For instance, the nanoparticles may be carrying drugs that prevent regulatory T cells (Tregs) or myeloid-derived suppressive cells (MDSCs), both of which are known to act in anti-tumor immunity as suppressors are the known culprits. Additionally, nanoparticles may be used to bring targeted immune checkpoint inhibitors to the tumor, which results in T cell activation and, hence, T cell killing cancer cells. Nanoparticles, capable of mediating the immune response on different levels like antigen presentation, cytokine production, and tumor immunosuppression stand as viable candidates in cancer immunotherapy, thus promising the development of innovative, entirely efficient, and personalized therapy options [29].
Various nanoparticles, including liposomes, polymeric nanoparticles, and metallic nanoparticles, significantly contribute to cancer immunotherapy, each offering distinct advantages [30]. Liposomes, as lipid-based carriers, excel in targeted drug delivery, influencing the tumor microenvironment precisely. Their biocompatibility and adjustable surface properties enable localized immune cell activation. Polymeric nanoparticles have many features including the ease of customizability. Possible applications in cancer therapy by enabling targeted delivery of immunomodulators that enhance cancer radiotherapy efficacy through oxygen metabolism reprogramming and antigens that could serve as therapeutic payloads (Fig. 4a) [23]. Nevertheless, immune checkpoint blockade (ICB) therapy against PD-1/PD-L1 using monoclonal antibodies offers limited benefits in glioblastoma (GBM), likely partly because of blood-brain barrier (BBB) and immune privileges. A recent study established a novel ICB strategy in which BBB-permeable ferritin nanocages were equipped with immunomodulatory payloads such as PD-L1 inhibitory peptides providing effective tumor targeting and BBB penetration, proving their potential in brain tumor growth suppression. In vitro, PD-L1-binding peptides (PD-L1pep) ferritin nanocages disrupted PD-1/PD-L1 interactions, restoring T-cell activity [31]. Metallic nanoparticles, like gold or silver, leverage unique physicochemical properties, allowing precise attachment of immunomodulators for targeted immune cell activation (Fig. 4b). Their plasmonic features can be utilized for localized hyperthermia, expanding their potential in cancer treatment. While promising, ongoing research focuses on optimizing formulations and addressing safety concerns, highlighting their pivotal role in shaping advanced and targeted cancer immunotherapies [32,33].
Figure 4: Overview of advanced biocompatible nanomaterials for cancer immunotherapy. (a) Schematic illustration of a nano-scale sensitizer incorporating metal and phenolic components to enhance cancer radiotherapy efficacy and immunogenic response through oxygen metabolism reprogramming, adapted with permission [23]. Copyright © 2022, Wiley Publishers. (b) Nanocages blocking immune checkpoints penetrate the blood-brain barrier and hinder the growth of brain tumors, adapted with permission [31]. Copyright © 2024, American Chemical Society. (c) Illustration of the mechanism of action of hybrid nanovesicles formed by the fusion of liposomes and tumour-derived nanovesicles with immunogenic properties for cancer immunochemotherapy, adapted with permission [36]. Copyright © 2021, American Chemical Society.
Liposomes are one of the most widely used nanomedicine, especially in cancer. They are largely employed in cancer immunotherapy, providing unique capabilities that advance therapeutic approaches significantly. Liposomes are composed of amphiphilic lipid molecules. They serve as effective carriers for several immunomodulatory compounds and therapeutic agents. Their versatility stems from their biocompatibility and the ability to encapsulate both hydrophilic and hydrophobic substances with good cargo loading efficiency, enabling precise drug delivery and reducing unintended effects, but the stability factor needs to be further considered [34]. In cancer immunotherapy, by encapsulating immunomodulators like cytokines or checkpoint inhibitors and chemotherapeutic drugs, liposomes ensure targeted delivery to the tumor microenvironment. This focused delivery approach enhances the concentration of therapeutic agents further intensifying their impact on immune cell activation and promoting strong anti-cancer immune responses [35]. Recently (DOX@LINV), a DOX-loaded hybrid nanovesicle by combining artificial liposomes (LIPs) through tumour-derived nanovesicles (TNVs) was fabricated for effective immunochemotherapy (Fig. 4c). DOX@LINV, featuring homologous targeting, triggers immunogenic cell death, enhances tumor immunogenicity, and activates dendritic cells, fostering an antigen-specific T-cell immune response. Moreover, it exhibits specific antitumor effects in murine melanoma, lung cancer, and breast cancer by improving the tumor microenvironment. In [36], Liposomes can encapsulate tumour-associated antigens, aiding their delivery to antigen-presenting cells. This can stimulate a specific and localized immune response against cancer, forming the basis for therapeutic cancer vaccines. Liposomes can be easily subjected to surface modifications which will in turn allow incorporation of multiple antigens, optimizing the immune response and potentially expanding the range of targeted malignancies. It is to be noted that several liposomal nanoformulations with drugs like doxorubicin, demonstrate enhanced anti-tumour efficacy with minimized systemic toxicity. Liposomal formulations of immunomodulators like interleukin-2 or interferon-alpha are also explored for boosting immune responses against cancer. While promising, ongoing research focuses on refining liposome formulations for stability, enhanced drug-loading, and addressing challenges like rapid clearance. The adaptability of liposomes underscores their pivotal role in advancing targeted and effective cancer treatments [37,38].
Lipid nanoparticles (LNPs) have emerged as promising carriers for cancer vaccines and in-situ CAR-T constructs in cancer immunotherapy due to their unique properties and versatility. In general, LNPs are usually a solid or semi-solid lipid core covered by a layer of emulsifiers or surfactants that make up an ultra-robust platform for the delivery of therapeutic agents [39]. In the case of cancer vaccines, LNPs can be formulated into nucleic acid delivery vehicles that protect encapsulated nucleic acids, proteins, or peptides during cell internalization and processing. Treated LNPs can deliver various therapeutic agents into APCs such as dendritic cells, which process and then present the antigens at their cell surface to induce T cells and start a strong immune response against cancer cells. This property of LNPs provides an enhancement in stability and bioavailability for the encapsulated material, translating to substantially improved efficacy in cancer vaccines by engendering long-lasting T-cell immunity and specific targeting of antigens of tumors. In the case of in-situ CAR-T constructs, LNPs play a very central role in the delivery of genetic material that engineers T cells within the body of patients. In contrast, traditional CAR-T cell therapy presents logistic complications and is time-consuming. LNPs can make the process easier by directly delivering mRNA or DNA that expresses the chimeric antigen receptor into T cells in vivo. This methodology takes advantage of LNPs’ natural potential for shielding and delivering nucleic acids across cellular membranes, thus allowing for efficient in vivo transfection of the patient’s T cells. In this manner, in-situ CAR-T cell generation can significantly reduce the complexity and cost of CAR-T cell therapy, make it much more accessible, and probably expand its use to many more patients and cancer types [40,41].
Besides, LNPs can target tissues or even cells in a very specific manner; hence, increasing the precision of cancer immunotherapy. Targeting ligands could be bound onto the surface of LNPs, which would thus be able to locate into tumor cells or specific subpopulations of immune cells and deliver the therapeutic agents there, enhancing the local concentration at the target site while minimizing off-target effects. Targeted delivery enhances the effect of treatment and reduces the risk of adverse reactions. LNPs can certainly be a game-changer in cancer immunotherapy by providing an efficient vehicle for both cancer vaccines and in-situ CAR-T constructs. Having the potential to protect and deliver nucleic acids into targeted cells, and meanwhile being able to easy the production of therapeutic T cells, makes them stand out with the ability to tighten up efficacy, accessibility, and safety for cancer treatments [42,43].
Polymeric nanoparticles are pivotal in cancer immunotherapy, offering unique attributes that significantly contribute to therapeutic advancements. With customizable structures and versatile compositions, they efficiently carry various immunomodulatory compounds and therapeutic agents. The enhanced biocompatible nature with tissue-specific biodegradability along with adjustable surface properties, and capacity to encapsulate diverse cargo make them one of the most attractive nanoparticles for targeted drug delivery [44]. In cancer immunotherapy, polymeric nanoparticles deliver immunomodulators like cytokines or immune checkpoint inhibitors along with standard chemotherapeutics to the tumor microenvironment. This targeted delivery minimizes side effects while maximizing therapeutic impact on immune cell activation, fostering strong anti-cancer responses. Their tuneable size and surface properties optimize vaccine formulations, improving efficacy in inducing immune memory against cancer cells. Examples include poly (lactic-co-glycolic acid) (PLGA) and chitosan-based nanoparticles [45]. PLGA encapsulates immunomodulators with sustained release and enhanced therapeutic effects [46]. Chitosan nanoparticles, due to biocompatibility, are explored for mucosal delivery of cancer vaccines [47]. In an interesting work, metabolic glycoengineering was employed to label dendritic cells DCs for creating nanoscale artificial antigen-presenting cells (aAPCs) in cancer immunotherapy. The azido-labeled DC membrane is applied to polymeric nanoparticles loaded with imiquimod, and anti-CD3ε antibodies are then modified through click chemistry (Fig. 5a). These nanoscale aAPCs demonstrate improved distribution in lymph nodes, stimulating T cells and resident antigen-presenting cells. Vaccination with these nanoscale aAPCs significantly inhibits tumor inoculation and growth, with additional enhancement achieved by combining with PD1 therapy [48]. Ongoing research aims to optimize polymeric nanoparticles for stability, improved drug-loading, and addressing challenges like biocompatibility and immunogenicity. The adaptability and versatility of polymeric nanoparticles underscore their critical role in advancing targeted and effective cancer treatments.
Figure 5: Innovative nanotechnology approaches in cancer immunotherapy. (a) Schematic illustration of enhancing cancer immunotherapy through the creation of nanoscale artificial antigen-presenting cells built upon polymeric nanoparticles via metabolic labelling of dendritic cells, adapted with permission [48]. Copyright © 2021, American Chemical Society. (b) Cancer immunotherapy utilizing gold/platinum metal nanoparticles derived from microcapsules of yeast in a synergistic approach of chemo dynamic and photothermal actions, adapted with permission [53]. Copyright © 2023, American Chemical Society. (c) Illustration of the study which concludes that the innate immune function of human B lymphocytes remains unaffected by polymer-coated gold nanospheres, adapted with permission [54]. Copyright © 2019, American Chemical Society.
Metallic nanoparticles, each with distinct properties, are widely used in biomedicine. Surface modification enables precise immune cell targeting, enhancing anti-cancer response [49,50]. Metallic nanoparticles can be easily subjected to surface modifications to design them as targeted drug delivery vehicles. The unique plasmonic properties of metals can have implications for both diagnostics and therapy, enabling the application in both imaging and photothermal therapy [51]. Extensively studied for cancer immunotherapy, gold nanoparticles, especially when functionalized, can act as carriers for immunomodulators or antigens, facilitating precise delivery to immune cells. Moreover, engineered gold nanoparticles can interact with specific immune system components; further influencing immune responses against cancer [52]. In a recent study, gold and platinum nanoparticles were synthesized within yeast microcapsules to improve antitumor treatment alongside chemodynamic therapy (CDT) and photothermal therapy (PTT). The resulting nanoscale particles (Bre-YAP) effectively mediated CDT and PTT, distributed well in tumors and lymph nodes, and, upon laser irradiation, transformed the microenvironments of tumors and draining lymph nodes (Fig. 5b). This led to substantial inhibition or eradication of primary tumors, contributing to an enhanced overall survival in mice [53]. Interesting research exposes human B lymphocytes to different types of gold NPs with varied surface coatings and shapes. Polymer-coated gold NPs show minimal interaction with B lymphocytes, while uncoated ones exhibit a functional impairment on activated B lymphocytes (Fig. 5c). Moreover, none of the NPs affect cell viability or immune activation markers [54]. While the evident potential of metallic nanoparticles in cancer immunotherapy is acknowledged, ongoing research is concentrated on optimizing formulations, comprehending their interactions in the intricate tumor microenvironment, and addressing concerns about biocompatibility and long-term safety. The versatile properties of metallic nanoparticles position them as valuable tools, transforming the landscape of cancer immunotherapy with targeted and effective treatments.
Interaction between nanoparticles and immune cells
Nanoparticles possess distinctive properties that dictate their interaction with various immune system components, crucial for modulating immune responses therapeutically [55]. An essential factor is their engagement with macrophages, key immune players responsible for phagocytosis. Depending on the physical and chemical properties of nanoparticles such as size and surface charge, they may either be engulfed by macrophages or evade the immune system. Nanoparticles can be surface engineered to enhance T cell responses selectively against cancer cells. NK cells, on the other hand can also be influenced by nanoparticles, promoting targeted cytotoxicity against cancer cells. This interaction is integral for developing strategies utilizing the innate immune system for therapeutic purposes (Fig. 6). For instance, liposomes loaded with immunomodulatory agents can influence macrophage polarization, skewing the immune response towards an anti-tumor phenotype [56,57]. Nanoparticle surface modifications are vital in cellular interactions, especially with primary immune cells. A hydrophilic PEG layer on polysaccharide-based nanoparticles prevents undesired aggregation and decreases non-specific cell uptake (Fig. 7a). Conversely, a low molecular weight dextran layer enhances nanoparticle uptake by antigen-presenting cells, triggering increased surface maturation markers and proinflammatory cytokine production [58]. A recent study shows that the size of nanoparticle carriers plays a crucial role in influencing intra-lymph node transport and cellular interactions (Fig. 7b). The biological fate of administered nanoparticles is influenced by follicular dendritic cell networks. Smaller nanoparticles (5–15 nm) clear within 48 h, while larger ones (50–100 nm) persist for over 5 weeks, exhibiting enhanced antigen delivery. Polymeric nanoparticles for dendritic cell-mediated antigen delivery elicit pote7nt immune responses in cancer vaccine studies. Metallic nanoparticles regulate T-cell activation and cytokine production. Manipulating nanoparticle-immune cell interaction is crucial for tailored immunotherapy [59].
Figure 6: The schematic illustration depicts how various types of nanoparticles uniquely interact with immune cells, including macrophages, dendritic cells, T lymphocytes, and natural killer cells.
Figure 7: Impact of nanoparticle modifications on immune responses. (a) Schematic illustration of the effects of PEG and dextran surface modification on polysaccharide-based nanoparticles and its impact on immune cell binding and stimulatory properties, adapted with permission [58]. Copyright © 2017, American Chemical Society. (b) Effect of nanoparticle size on antigen retention and presentation in lymph node follicles, impacting humoral immunity, adapted with permission [59]. Copyright © 2019, American Chemical Society. (c) Illustration of the study which concludes that the innate immune function of human B lymphocytes remains unaffected by polymer-coated gold nanospheres, adapted with permission [68]. Copyright © 2021, American Chemical Society.
Macrophages and dendritic cells
In cancer immunotherapy, macrophages and dendritic cells play crucial roles, directing immune responses against invading cancer cells [60]. Immunotherapy aims to boost macrophages’ tumour-fighting abilities and the antigen-presenting capacity of dendritic cells. Both cell types are vital for successful immunotherapy, and ongoing research refines strategies utilizing their unique functions. Hence it is important to understand the mechanism of action of both these cell types in order to specifically design a precision medicine and tailoring targeted immunotherapies for better-quality patient outcomes [61,62]. Nanoparticles with diverse characteristics may interact differently with macrophages, potentially evading phagocytosis, and altering interactions through surface charge and modifications like polyethylene glycol. Dendritic cells interact with nanoparticles designed for antigen delivery, influencing antigen presentation and subsequent immune activation. The choice of nanoparticle material and formulation impacts antigen uptake and processing, influencing the immune response’s strength and specificity. Liposomes carrying antigens enhance dendritic cell activation, fostering robust immune responses, especially in cancer vaccines [63]. Tuneable polymeric nanoparticles proficiently deliver antigens to dendritic cells, triggering potent immune reactions. Precisely fabricated nanoparticles interact with both macrophages and dendritic cells for effective immunotherapy. In cancer treatment, nanoparticles re-orient tumor-supportive macrophages towards an anti-tumor state, enhancing anti-cancer immune responses. Immunomodulatory nanoparticle-loaded agents redirect macrophages from pro-tumour behaviour, fostering an environment conducive to anti-cancer immunity. Tailoring nanoparticle properties for precise target recognition, uptake, and immune modulation is crucial for advancing precision medicine and personalized immunotherapy [64].
T lymphocytes and NK cells are integral to immunotherapy, with T lymphocytes, vital for adaptive immunity, recognizing and targeting specific antigens, including those expressed by cancer cells. Immunotherapeutic approaches focus on enhancing T cell responses, either by direct activation or modification outside the body, reinforcing their anti-cancer capabilities upon re-introduction. NK cells, innate immune system components, specialize in identifying and eliminating abnormal cells, like cancer cells. Immunotherapy aims to harness and amplify NK cell activity, improving their ability to target and destroy malignant cells through engineered NK cells or therapeutic agents stimulating their anti-cancer responses. Understanding and manipulating T lymphocytes and NK cells are crucial for developing effective immunotherapies [65,66]. Ongoing research seeks to optimize strategies enhancing the specificity, potency, and persistence of these immune cells, promising more successful and personalized immunotherapeutic interventions against various diseases, particularly cancer. The interplay between nanoparticles and T lymphocytes is intricate, influencing multiple aspects of T cell biology in immunotherapy. Engineered nanoparticles carrying immunomodulatory agents or antigens can enhance T-cell responses against cancer cells [67]. Lymph nodes, vital for immune responses, are targeted for efficient delivery of immunomodulators in cancer treatment (Fig. 7c). Using pH/redox dual-sensitive micelles (PLH-PEG), an interesting study achieved an 8.12-fold increase in lymph node accumulation 48 h post-injection. This approach activated Cytotoxic T Lymphocytes (CTLs) and NK cells, showing a 93.76% tumor inhibition rate and mitigating the immunosuppressive microenvironment. This highlights the potential of lymph node delivery for cancer immunotherapy [68]. NK cells are pivotal targets for immunotherapeutic interventions due to their role in identifying and eliminating abnormal cells. The interaction between nanoparticles and NK cells is crucial for harnessing their cytotoxic activity against cancer. Engineered nanoparticles can enhance NK cell function, delivering immunomodulatory agents or directly interacting with NK cell receptors to potentiate killing cancer cells. Liposomes or polymeric nanoparticles encapsulating immunomodulatory compounds interact with T lymphocytes and NK cells, offering controlled release to influence immune responses. Surface-modified nanoparticles with ligands targeting T cell or NK cell receptors enhance therapeutic impact [68,69]. Understanding nanoparticle interactions is pivotal for advancing immunotherapies, with ongoing research focusing on innovative strategies to optimize specificity, potency, and persistence of immune responses against cancer and other diseases, leveraging nanoparticles as adaptable carriers for immunomodulatory agents in personalized treatments.
Influence of Nanoparticles on Immune Cell Activity
Nanoparticles actively influence the behaviour of immune cells upon introduction into the body. Meticulously designed nanoparticles, including liposomes, polymeric, and metallic ones, interact with immune cells like macrophages, dendritic cells, T lymphocytes, and NK cells. These interactions affect immune responses, changing cytokine production, antigen presentation, and the growth, differentiation, and movement of immune cells. Especially in cancer, nanoparticles notably enhance immune cell activity by promoting their penetration into tumor tissues. More importantly, in the case of the immune system, nanoparticles can interact with macrophages, dendritic cells, and T cells, modulating the immune responses. Many of the nanoparticles can function as immunomodulators and the interaction can be fine-tuned by precisely engineering surface modifications and ligands on their surface. This aspect was widely employed in immunotherapy. After being administrated, nanoparticles often have contact with macrophages first. Macrophages will recognize these particles to internalize them and attempt to digest them [70]. Depending on their size, shape, and surface chemistry, the nanoparticles will either be appropriately processed by macrophages or, through activation, release cytokines and other signalling molecules that help in the modulation of immune responses. Nanoparticles can also be engineered to target dendritic cells directly with antigens, harnessing their ability to activate T cells more effectively [71]. This interaction can become especially useful in vaccine development when nanoparticles act due to carrier performance for antigens, thus enhancing delivery and presentation to the immune system. Nanoparticles can directly influence the activation of T cells through antigen delivery and indirectly due to modulation of the activity of dendritic cells and macrophages. B cells can produce antibodies against antigens complexed to nanoparticles, and therefore a humoral immune response takes place. Those cytokines released from macrophages and dendritic cells in response to nanoparticles might further be involved in the activation of NK cells and hence take part in innate immunity. In general, the interactions of nanoparticles with immune cells denote multifaceted complexity, offering great therapeutic and diagnostic applications [72]. Scientists can develop advanced nanomedicine strategies related to targeted drug delivery, vaccine development, and immune modulation by understanding and manipulating these interactions.
Modulation of immune cell activation
Nanoparticles play crucial role in the field of cancer immunotherapy by enhancing immune responses selectively against specific tumor subtypes. Mainly they modulate immune cell activation by influencing cytokine production, antigen presentation, and proliferation of macrophages, dendritic cells, T lymphocytes, and NK cells [73]. Liposomal nano formulations loaded with various immunomodulatory agents shift pro-tumor macrophages towards an anti-tumor phenotype, creating a favorable environment for anti-cancer responses. Polymeric nanoparticles, on the other hand proficiently deliver antigens to dendritic cells, forming the basis for potent cancer vaccines. Surface modifications of nanoparticles or nanoparticles encapsulated with immunomodulatory and anticancer agents activate NK cells, showcasing nanoparticles’ role in refining immune responses. Nanoparticle properties like size, shape, and surface charge intricately influence their interaction with immune cells and the delivery of therapeutic agents. Surface modifications, using ligands or antibodies, further refine interactions, guiding nanoparticles to specific immune cell populations. Examples demonstrate nanoparticles, like gold and polymeric variants, modulating T cell activation and cytokine production [74]. This dynamic process holds profound implications for immunotherapy, showcasing the adaptability of nanoparticles in shaping immune responses.
Cytokines, signalling molecules produced by immune cells are major players in the immune system to regulate immune activity. Although they are produced in very trace volumes upon specific antigenic response, in immunotherapy, intentionally varying cytokine profiles of crucial immune cells is crucial. In this line of engagement, activation of macrophages in an attempt to generate pro-inflammatory cytokines like interleukin-12 (IL-12) will definitely bolster the anti-tumor response. On the other hand, inducing anti-inflammatory cytokines such as interleukin-10 (IL-10) will help to curb excessive inflammation to control auto immune disorders [75,76]. Dendritic cells can also be modulated to produce cytokines that foster T-cell activation, a vital aspect in crafting effective cancer vaccines. T lymphocytes release cytokines to synchronize immune responses, inducing the strength and specificity of adaptive immunity. Adjusting cytokine production by NK cells is not only relevant in immunotherapy but also in managing autoimmune diseases, where symptom relief entails dampening pro-inflammatory cytokines. Immunotherapy utilizes diverse approaches like immunomodulatory agents, cytokine therapies, or engineered nanoparticles for precise cytokine delivery to finely tune immune responses [77]. These approaches seek to balance effective disease control while avoiding immune-related adverse effects. Overall, cytokine modulation stands as a versatile and sophisticated method in immunotherapy, offering valuable tools to tailor immune responses for therapeutic benefits across a range of diseases.
Antigen presentation stands as a pivotal immune process wherein APCs exhibit pathogen or abnormal cell fragments, signalling threats to other immune cells, notably T lymphocytes. Tailoring immune responses via antigen presentation holds paramount importance in both immunotherapy and disease management [78]. It encompasses strategically adjusting antigen presentation to either bolster or dampen immune responses for therapeutic ends. Dendritic cells, specialized APCs, assume a central role in this mechanism, taking, processing, and presenting antigens to initiate immune responses. Heightening antigen presentation through nanoparticle engineering for antigen delivery to dendritic cells emerges as crucial for effective vaccines and immunotherapies, particularly in cancer treatment [79]. This strategic modulation is also applied in autoimmune diseases to suppress immune responses. Strategies inhibiting or altering antigen presentation can mitigate autoreactive T cell activation, reducing inflammation in autoimmune disorders. Antigen presentation modulation is pivotal in developing targeted and personalized immunotherapies, and ongoing research aims to optimize these strategies for more effective treatments and vaccines across various diseases.
Impact on immune cell proliferation and differentiation
Nanoparticles can impact across various immune cell populations, including T lymphocytes, B cells, APCs, and NK cells. In T lymphocytes, engineered nanoparticles carrying antigens or immunomodulatory agents can enhance proliferation and guide the differentiation of these cells into specific subsets, tailoring immune responses [80]. Correspondingly, many immune modulatory nanoparticles stimulate the proliferation and differentiation of B cells thereby influencing antibody production and the humoral immune response. It is to be noted that APCs, especially dendritic cells, are mainly modulated by nanoparticles. This is very crucial for initiating adaptive immune responses which can further check the proliferation of invading tumor cells. Even NK cells, vital for innate cytotoxic activity, experience nanoparticle-mediated effects, enhancing their potential against diseased cells. Therefore, nanoparticles are known to affect both innate and adaptive immune responses. In the case of T cell activation, nanoparticles exhibit the ability to enhance immune responses by delivering antigens or immunomodulatory agents. Their controlled release ensures nuanced modulation, crucial for tailored therapeutic applications [81]. Simultaneously, nanoparticles influence dendritic cell maturation, vital for initiating adaptive immune responses [82]. The strategic manipulation of dendritic cell maturation by nanoparticles shows promise in optimizing immunotherapeutic efficacy. Metallic nanoparticles, with unique properties, can be tailored to promote dendritic cell maturation, contributing to orchestrated adaptive immune responses. Precise targeting is enabled through surface modifications with ligands, directing nanoparticles to specific immune cell populations and enhancing their modulatory effects.
Regulation of immune cell migration and infiltration into tumor tissues
Engineered nanoparticles have a profound impact on immune cell movement toward tumor sites, particularly enhancing T lymphocytes’ migratory capabilities, crucial in anti-tumor responses. Nanoparticles, delivering therapeutic payloads, optimize T cell homing to tumor tissues, concentrating immune cells for improved cancer cell recognition and elimination. They also regulate immune cell infiltration into tumors, a critical factor in immunotherapy success. Nanoparticles interact with the tumor microenvironment, strategically influencing signaling pathways for efficient immune cell penetration. Liposomal nanoparticles with chemokines enhance T cell migration, polymeric nanoparticles release factors promoting immune cell infiltration, and metallic nanoparticles, tailored for the tumor microenvironment, affect immune cell behavior. Nanoparticle properties crucially dictate interactions with immune cells, impacting migratory processes. Precise targeting through surface modifications enables specific immune cell interaction, optimizing the effect on migration and infiltration. Modulating these processes strategically holds promise for personalized therapies, especially in cancer.
Improving nanoparticle penetration into tumors is a vital focus in cancer immunotherapy, addressing challenges posed by the complex tumor microenvironment. Engineered nanoparticles are designed to overcome obstacles such as abnormal blood vessels and dense matrices. Surface modifications, especially in polymeric nanoparticles, enhance interactions with tumor cells and the extracellular matrix, enabling deeper penetration [83]. Responsive polymeric nanoparticles adapt to the tumor environment, releasing therapeutic payloads effectively. The size of nanoparticles, particularly small ones, aids efficient navigation through tumor vasculature. Surface modifications with ligands or antibodies enable targeted interactions with cancer cells, improving penetration. Strategies include liposomal nanoparticles with modified surfaces and polymeric nanoparticles responsive to tumor conditions [84]. Metallic nanoparticles, with unique properties, can overcome barriers, enhancing distribution within tumors. Enhancing tumor penetration is crucial for delivering therapies effectively and nurturing interactions with immune cells, which can further eliminate the tumors. Various mechanism and precise topological modifications can improve nanoparticle penetration to boost immunotherapy. In summary, refining engineered nanoparticles to enhance tumor penetration in immunotherapy is a strategic solution to tackle challenges in the tumor microenvironment, offering better delivery of therapeutic payloads and overall efficacy in cancer treatment.
Improved targeting of cancer cells
Improving cancer cell targeting with nanoparticles is crucial for advancing immunotherapy, ensuring precise delivery of therapeutic agents to malignant tissues. Nanoparticles offer versatility in tailoring targeting mechanisms, with surface modifications enabling selective recognition and binding to cancer cells [85]. Immunoliposomes, for example, functionalized with antibodies, ensure highly specific interactions with cancer-specific antigens [86]. Responsive polymeric nanoparticles enhance targeting by adapting to the unique cancer cell microenvironment, responding to factors like acidic pH. This controlled release minimizes off-target effects, optimizing treatment precision. Metallic nanoparticles, leveraging distinct properties, can be engineered for enhanced cancer cell targeting, responding to external stimuli within the tumor microenvironment. The size of nanoparticles is critical; small ones exploit leaky tumor vasculature, facilitating accumulation due to the enhanced permeability and retention effect. Strategies include liposomal nanoparticles with antibody functionalization and polymeric nanoparticles responsive to tumor pH. Metallic nanoparticles, modified with peptides targeting cancer-associated proteins, showcase precision in recognizing and binding to cancer cells. Advancements in cancer cell targeting contribute not only to therapeutic payload delivery but also facilitate interactions with immune cells in the tumor microenvironment. This optimization of immunotherapies promotes targeted and personalized cancer treatment [87].
Nanoparticles in Cancer Immunotherapy
Nanoparticles play a pivotal role in immunotherapy, acting as efficient drug carriers by encapsulating therapeutic agents for controlled release at specific body sites, enhancing efficacy and reducing systemic side effects. Their molecular interactions with immune cells, facilitated by engineered surface properties, promote uptake and modulate immune responses. Size and shape influence nanoparticle accumulation in lymph nodes, optimizing antigen presentation and immune reactions. Physicochemical features like surface charge affect interactions, influencing cellular uptake and immune cell activation. Nanoparticles also impact the tumor microenvironment, overcoming immunosuppression by delivering agents that inhibit immune checkpoints, activating cytotoxic T cells, and remodelling the extracellular matrix for enhanced immune cell penetration (Fig. 8).
Figure 8: Scheme illustrating the potential of modified nanoparticles for immunotherapy could depict the mechanistic effects on various immune cells, including macrophages, dendritic cells, NK cells, and T cells, created with biorender.com.
Augmentation of antigen presentation and cross-presentation
Antigen presentation is the process of exhibiting foreign or irregular molecules (antigens) on APCs to trigger an orchestrated immune recognition. This enables the immune system to react to pathogens or cancerous cells. Major histocompatibility complex (MHC) molecules, crucial in this mechanism, present antigens to T cells to initiate immune responses. Cross-presentation is a specific form where external antigens are presented on MHC class I (MHC-I) molecules, typically associated with internally produced antigens. Dendritic cells demonstrate proficiency in cross-presentation, linking innate and adaptive immune reactions. This mechanism is essential for eliciting cytotoxic T-cell responses which is necessary for identifying and eradicating foreign antigens and abnormal cells, including cancers [88]. They efficiently deliver antigens to APCs, particularly dendritic cells, safeguarding them from degradation and facilitating targeted delivery. Engineered nanoparticles influence APC interactions through size, surface charge, and modifications, promoting efficient cross-presentation on MHC-I molecules. Additionally, nanoparticles carry adjuvants, intensifying APC activation and immune response [89]. Nanoparticles derived from mung beans demonstrate high efficacy in transporting model antigens and adjuvants, showcasing their promise in cancer immunotherapy. These vaccine nanoparticles efficiently travel to lymph nodes, trigger dendritic cell maturation, boost antigen cross-presentation, and stimulate CTLs (Fig. 9a). Consequently, this results in heightened secretion of cytokines and antibodies, which effectively suppress tumor growth in vivo [90]. Controlled antigen release from nanoparticles sustains presentation, fostering memory T cell development. Nanoparticles, by modulating intracellular antigen trafficking, ensure efficient cross-presentation.
Figure 9: Advanced nanotechnology strategies for enhancing cancer immunotherapy. (a) Schematic illustration of nano-sized vaccines from mung bean extracts for cancer immunotherapy, adapted with permission [90]. Copyright © 2021, American Chemical Society. (b) Nanovesicles engineered from dendritic cell membranes for precise T cell activation, adapted with permission [93]. Copyright © 2022, American Chemical Society. (c) Illustration of tumour-associated macrophage polarization via nanoparticle-loaded Escherichia coli coupled with immunogenic cell death for cancer immunotherapy. adapted with permission [97]. Copyright © 2021, American Chemical Society.
Potentiation of T cell responses
T-cell responses are vital in cancer immunotherapy [91]. Nanoparticles enhance T cell-mediated immune responses by carrying immune-modulating agents. This protects them from premature degradation and ensures targeted delivery to immune cells, including T cells. This targeted delivery enhances T cell activation at the tumor site, overcoming the immunosuppressive cancer microenvironment. Moreover, nanoparticles can be tailored to promote antigen presentation to T cells [92]. By encapsulating tumor antigens or peptides, nanoparticles safeguard these antigens, allowing controlled and sustained release. This controlled release ensures prolonged exposure of T cells to antigens, facilitating their activation for an effective immune response against cancer cells. Dendritic cells and their nanovesicles show promise in directing immune responses, especially for cancer immunotherapy (Fig. 9b). In a study, nanovesicles derived from dendritic cell membranes, engineered through nitrogen cavitation, exhibit similar properties to dendritic cell exosomes. These nanovesicles effectively activate antigen-specific T cells, offering a potential immunotherapy platform [93]. Furthermore, nanoparticles can actively engage with T cells through surface modifications. By incorporating ligands or antibodies on their surfaces, nanoparticles can specifically target and bind to T cells, promoting interactions that enhance T cell activation. These surface modifications can mimic natural signalling mechanisms, providing costimulatory signals to T cells, which are essential for their proper activation and proliferation [94]. The physical properties of nanoparticles, such as size and shape, also influence their interaction with T cells. Nanoparticles of optimal size can efficiently traffic to lymph nodes, where T cell priming often occurs. Moreover, nanoparticles contribute to the persistence of T cell responses. They can be engineered to act as depots, gradually releasing immunomodulatory agents over time [95]. This sustained release ensures a prolonged activation of T cells, promoting durable and effective anti-tumor immune responses.
Overcoming immunosuppressive tumor microenvironment
The tumor microenvironment (TME) is a hurdle for immunotherapy by creating an immune-suppressive background that delays natural anti-tumor responses. Henceforth it is critical to design strategies to overcome such a niche for efficient therapeutic outcomes. Key components of TME like regulatory T cells, myeloid-derived suppressor cells, and immunosuppressive cytokines contribute to this inhibitory nature. Nanoparticles are instrumental in addressing these challenges in cancer immunotherapy. They excel in delivering immunomodulatory agents directly to TME, addressing inhibitory molecules like immune checkpoint proteins and cytokines. Engineered nanoparticles encapsulate drugs targeting these factors, mitigating immunosuppressive signals. This innovative approach promotes a more permissive environment for effective anti-tumour immune responses, presenting a promising strategy to overcome TME-associated obstacles in cancer treatment [96]. Their distinct physical and chemical properties enable enhanced penetration of immune cells into solid tumors, overcoming the dense extracellular matrix that typically hinders infiltration. Nanoparticles enhance immune access and anti-tumor response by disrupting the matrix (Fig. 9c). They redirect tumor-associated macrophages from pro-tumoral to anti-tumoral, altering the TME to support immunity [97]. Nanoparticles aid in activating cytotoxic T cells within the TME by precisely delivering antigens and adjuvants, promoting their activation and proliferation. Additionally, nanoparticles modulate oxidative stress, a strategy to sensitize cancer cells to immune attack by disrupting their evasion mechanisms [98,99].
Regulatory Mechanisms in Nanoparticle-Mediated Immunomodulation
Nanoparticle-driven immunomodulation is a burgeoning field harnessing nanoparticles to finely adjust immune responses for therapeutic ends. Nanoparticles are subjected to modification to either enhance immune reactions, beneficial in vaccines and cancer immunotherapy or regulate the excessive immune responses, remarkably in the cases of autoimmune conditions. These carefully calibrated nanoparticles serve as efficient carriers for targeted drug delivery, reducing off-target toxicities and improving treatment effectiveness. Biocompatibility is a very crucial parameter to ensure the safety of nanoparticle use in medical applications. Exploring the intricate interactions between nanoparticles and the immune cells, including signalling pathways and cytokine production, is essential for understanding their mechanisms (Fig. 10). The applications of nanoparticle-mediated immunomodulation extend across various medical domains, promising more precise and effective therapeutic approaches.
Figure 10: Schematic illustration of the mechanistic action of nanoparticles loaded immune agents for cancer immunotherapy (created with biorender.com).
Signalling pathways involved in immune cell modulation by nanoparticles
Deciphering the molecular mechanism of the involved signalling pathways is crucial to understand how nanoparticles either activate or suppress immune cell functions. Certain nanoparticles engage toll-like receptors (TLRs), to modulate the immune responses [100]. Some nanoparticles exhibit dual functionality wherein they both stimulate and suppress immune responses. The interactions of nanoparticles with the immune system engage key signalling pathways critical for the efficacy of cancer immunotherapy and involve different molecular mechanisms and regulatory networks [101]. The toll-like receptor (TLR) signalling pathway is one major pathway that may be so designed that nanoparticles can activate TLRs on immune cells such as dendritic cells and macrophages. It is due to their binding by TLRs, which subsequently leads to downstream signalling cascades, including Myeloid Differentiation Primary Response 88 (MyD88) and TIR-domain-containing Adapter-inducing Interferon-β (TRIF) adaptors [102]. These eventually lead to activation of the Nuclear factor kappa B (NF-κB) and Interferon Regulatory Factors (IRF) transcription factors, resulting in productive pro-inflammatory cytokine and type I interferon responses that will improve antigen presentation and provoke strong adaptive immune responses. Another important pathway is through the stimulator of interferon genes (STING) pathway. In this case, mostly nanoparticles delivering cytosolic DNA or cyclic dinucleotides could stimulate STING in immune cells [103]. STING brings the phosphorylation and subsequent activation of TANK-Binding Kinase 1 (TBK1) and IRF3, followed by the efflux of interferons and other inflammatory cytokines which in turn guides the maturation and activation of dendritic cells, which are then capable of T-cell priming to mount anti-tumor immunity. Similarly, the Phosphoinositide 3-Kinase (PI3K)/Protein Kinase B (Akt)/mammalian Target of Rapamycin (mTOR) signalling pathways also control immune system responses to nanoparticles [104]. It affects both the survival, proliferation, metabolism, and differentiation of immune cells. Nanoparticles have been reported to modulate the PI3K/Akt/mTOR pathway, including T cells and macrophages. Activating PI3K/Akt signalling can improve the survival and effector functions of T cells, and can promote an M1 anti-tumor phenotype in macrophages. Thus, using nanoparticles to modulate this pathway could enhance anti-tumor immunity, by increasing the activity of immune cells toward tumors. In the same way, nanoparticle interactions can regulate the cytokine responses mediated by Janus Kinase (JAK)/Signal Transducer and Activator of Transcription (STAT) signalling pathway [105]. Understanding these intricate biomolecular interactions and biochemistry of signalling pathways holds promise for therapeutic applications, spanning cancer immunotherapy, vaccine development, and autoimmune disease treatment.
Toll-like receptor (TLR) signalling
TLRs are vital components of the innate immune system that identify molecular patterns associated with pathogens. In the sphere of nanoparticle-mediated immunomodulation, TLR signalling pathways are central in shaping immune cell responses. Nanoparticles, with their distinctive physical and chemical traits, interact with TLRs on immune cell surfaces, initiating signalling events influencing immune cell modulation. Nanoparticles can activate TLRs to trigger the setting off of intracellular cascades. Ligand binding prompts TLRs to recruit adaptor proteins, activating downstream signalling pathways [106].
Pro-inflammatory cytokines are pivotal in TLR pathways influenced by nanoparticles. Nanoparticles activating TLRs prompt immune cells to release cytokines like Interleukin 6 (IL-6), Interleukin-1β (IL-1β), Tumor Necrosis Factor-alpha (TNF-α), and interferons, shaping immune functions. This impacts various immune cell types, including macrophages, dendritic cells, and B cells, crucial for immune coordination. Nanoparticle-TLR interactions boost dendritic cells’ antigen-presenting capacity, aiding immune maturation and effective threat responses. Additionally, nanoparticles modulate adaptive immune responses via TLR signalling, activating and maturing antigen-presenting cells [107]. This coordination is essential for recognizing and responding efficiently to pathogens or abnormal cells. Nanoparticles’ specificity for particular TLRs is influenced by their physical and chemical characteristics, encompassing size, shape, surface charge, and coating composition. These attributes dictate the preference of nanoparticles for specific TLRs, impacting the selectivity and strength of the immune responses triggered by interactions with TLRs. A comprehensive understanding of the intricate mechanisms involved in TLR signalling pathways, modulated by nanoparticles and influencing immune cell behaviour, is crucial for devising targeted immunomodulation strategies [108].
The NF-κB pathway regulates immune responses, inflammation, and cell survival. This family of transcription factors controls genes in multiple processes. It activates in response to stimuli like cytokines and pathogens. Normally inactive, NF-κB is sequestered by proteins like Inhibitor of κB (IκB). Activation, like ligand binding to cell receptors, triggers IκB degradation by the IκB kinase complex. This releases NF-κB, allowing it to move into the nucleus, where it influences gene expression related to immune responses and inflammation. The non-canonical activation mode involves processing p100 to generate the p52-RelB heterodimer, triggered by specific TNF receptor superfamily members. Understanding these NF-κB pathways is crucial for developing targeted interventions in immune-related processes [109]. Aberrant activation of NF-κB pathway contributes to chronic diseases’ pathogenesis, emphasizing its role in maintaining immune balance. Understanding NF-κB’s dynamics forms a foundation for targeted therapies in immune modulation. Research explores its complexities, offering therapeutic potential. Nanoparticles intricately influence the NF-κB pathway. Certain nanoparticles activate it, enhancing cytokine production and immune cell activation, while others act as inhibitors, tempering inflammation. Surface modifications further tailor nanoparticle-NF-κB interactions, crucial in diseases like cancer, infections, and autoimmunity [110]. Ongoing research delves into molecular details, shaping targeted immunotherapies through NF-κB modulation.
The JAK-STAT pathway, vital for immune cell modulation, engages with nanoparticles in a complex study field. This pathway transduces signals from extracellular cytokines, influencing immune responses by regulating gene expression. In nanoparticle-mediated immunomodulation, understanding the JAK-STAT pathway dynamics is crucial [111]. Initiated by cytokine binding to cell surface receptors, this pathway involves JAK activation, receptor phosphorylation, and subsequent STAT activation. Activated STAT proteins form dimers, translocating to the nucleus to influence gene transcription. Deciphering how nanoparticles modulate immune cells through this pathway is intricate, with potential implications for targeted immunotherapies. In the realm of immune cell modulation by nanoparticles, specific nanomaterials exhibit influence on the JAK-STAT pathway. Varied physicochemical properties of nanoparticles enable engagement with cell surface receptors, particularly those integral to the JAK-STAT pathway. This interaction can either activate or inhibit the pathway, depending on diverse factors. Activation enhances immune cell responses, as seen with gold nanoparticles stimulating JAK-STAT, leading to heightened expression of immune-related genes [105]. Conversely, certain nanoparticles act as inhibitors, curtailing downstream signalling and tempering immune reactions. Surface modifications, coatings, and ligands further shape these nuanced interactions, dictating nanoparticle specificity. Understanding these complexities is crucial for tailoring nanoparticles toward desired immunomodulation. Reference [112] exploring the involvement of the JAK-STAT pathway in nanoparticle-mediated immune cell modulation unveils promising prospects for targeted interventions in conditions like cancer, autoimmune disorders, and infectious diseases, contributing to the evolving landscape of precision immunotherapy in nanomedicine.
Influence of nanoparticle properties on immune responses
The impact of nanoparticle properties on immune responses is pivotal and intricate. Nanoparticles, characterized by unique physical and chemical attributes, wield diverse effects on the immune system, shaping both innate and adaptive responses. Parameters like size, shape, surface charge, composition, and coating collectively govern interactions with immune cells, shaping immunological outcomes [27]. Size serves as a fundamental determinant, influencing biodistribution, cellular uptake, and immune cell interactions. Nanoparticle shape is an additional factor steering immunomodulatory effects, with distinct shapes provoking specific immune responses. The geometry of nanoparticles governs cellular internalization, immune cell adhesion, and interactions with biological barriers, ultimately moulding their overall immunogenicity. Surface charge plays a crucial role in shaping nanoparticle interactions with the immune system. Positively charged nanoparticles may experience heightened uptake by immune cells through electrostatic interactions, while negatively charged counterparts could undergo altered interactions with cellular components, impacting their immunomodulatory properties. The surface charge also influences nanoparticle stability and aggregation in biological fluids, affecting systemic circulation and cellular interactions. The composition of nanoparticles, determined by their construction materials, is a key factor in dictating immunological effects [25,113]. Varied materials may elicit different immune responses, ranging from pro-inflammatory to immunosuppressive. For instance, metallic nanoparticles like gold or silver may provoke unique immune reactions compared to commonly used biodegradable polymers or lipids. Surface coating and functionalization significantly contribute to nanoparticle biocompatibility and immunomodulatory effects. Coating materials influence protein adsorption, opsonization, and cellular recognition, shaping the nanoparticle’s fate within the immune system. Nanoparticle properties, notably biocompatibility and biodegradability, are vital in nanomedicine. Biocompatible nanoparticles interact safely with biological systems, reducing the risk of adverse reactions. Surface modifications improve biocompatibility, enabling effective navigation in physiological environments. Biodegradability ensures safety and effectiveness, with controlled degradation minimizing long-term accumulation. Understanding these properties’ interplay with the immune system is crucial for tailoring nanoparticles in drug delivery, vaccines, and immunotherapy [114].
Resistance towards immunotherapy
Resistance to nanoparticle-mediated immunotherapy is one of the biggest hurdles in cancer treatment. Sources of resistance can vary and relate to the tumor microenvironment, genetically mutated cancer cells, or even the hosts themselves, through their immune regulatory mechanisms. Frequently, it is reported that the tumor microenvironment harbours such immunosuppressive features as high levels of Tregs, MDSCs, and immunosuppressive cytokines like Transforming Growth Factor-beta (TGF-β) and IL-10, which hinder the effectiveness of nanoparticle-mediated therapies [115]. It can also physically grow barriers around tumors, such as dense extracellular matrix components, that retarded and hindered the penetration and distribution of nanoparticles, thus maximizing the reduction in therapeutic efficiency. Genetic mutations and heterogeneity in cancer cells also result in resistance and enable tumor cells to circumvent detection and destruction by the immune system [116,117]. Genetic heterogeneity within tumors can be extensive and may result in the downregulation/mutation of antigens targeted by nanoparticles. Such antigenic variation should suggest that immune cells, even upon activation by a nanoparticle, would not actually recognize and kill all cancer cells. In addition, cancer cells themselves have been shown to alter their antigen presentation pathways, reducing the visibility of antigens to the immune system. Furthermore, cancer cells can up-regulate some immune checkpoint molecules, for example, PD-L1, to suppress T-cell activities by binding to the corresponding checkpoint receptors PD-1 on the surface of T cells, thereby counterbalancing the intended immunological activation of nanoparticle-mediated therapies [118]. However, this equilibrium can be disrupted by prolonged exposure to nanoparticles. Immune tolerance or anergy may occur over time as a result of this exposure, and this phenomenon includes the lack of responsiveness of immune cells to therapeutic agents necessary for fighting off diseases accordingly. Moreover, when not properly administered, nanoparticles with their therapeutic potential might cause unintended immune responses [119]. This might translate to chronic inflammation and allergies that increase treatment complications hence compromising patient outcomes in the end.
Optimizing nanoparticle-based therapies
Specific case studies illustrate a variety of nanoparticle-based immunotherapies in which new strategies and modifications make up for different issues. One notable example is the use of mRNA vaccines using LNPs, such as in the case of COVID-19 vaccines developed by Pfizer-BioNTech and Moderna. In this case, these vaccines use LNPs for the delivery of mRNA encoding the spike protein of SARS-CoV-2, inducing an enhanced immune response. The carrier LNPs protect the mRNA from degradation and facilitate its entry into cells and, eventually immune activation. This has resulted in a highly effective and safe approach for mRNA-based immunotherapy against cancer and infectious diseases [28]. Polymeric nanoparticles are also used for cancer immunotherapy. PLGA nanoparticle designs have been created by researchers that can carry tumor antigens and adjuvants to dendritic cells [120]. These nanoparticles can improve antigen presentation and activate effective cytotoxic T-lymphocyte responses against tumors in preclinical models. Here, co-encapsulation of the antigen together with the adjuvant in these nanoparticles facilitates the ability to trigger the immune system to recognize and attack cancer cells. To counter the challenges in the form of stability, effective delivery, and off-target effects, nanoparticles have to be designed with great care and their surface engineered to achieve optimal sizes and charges and kinetics of release.
Gold nanoparticles are largely used in photothermal therapy combined with immunotherapy [121]. These nanoparticles are functionalized with tumor antigens and antibodies against specific types of cancer cells and upon irradiation with near-infrared light, increase the local temperature and subsequently trigger the death of tumor cells, releasing antigens from the tumor; afterwards, they are engulfed by APCs to promote an immune response. In this way, this approach will not only directly kill cancer cells but also turn the tumor into an in-situ vaccine, promoting systemic anti-tumor immunity. Strategies in surmounting like challenges to this approach are the optimization of the size and shape of gold nanoparticles for maximal tumor penetration, which would have the least negative effects on biocompatibility and reduce possible toxicity. In another highly innovative application, researchers have engineered anti-PD-1-coated nanoparticles that target the TME to locally release the checkpoint inhibitor at a maximal concentration for T cell activity against the tumor, while reducing systemic side effects [122]. This may result in the avoidance of immune-related adverse events often associated with checkpoint inhibitors due to off-target immune activation. These case studies emphasize the versatility and potential of nanoparticle-based immunotherapies but also how nanoparticle design or changes in therapeutic strategies could be tailored to address specific challenges for improved clinical outcomes. Along this refinement path, further tuning of the efficacy and safety of nanoparticle-based therapies can continue to offer new hopes for the treatment of cancer and other diseases.
Challenges and Future Directions
The application of nanoparticles in cancer immunotherapy holds immense promise but faces challenges. Precise targeting to tumor sites is critical for minimizing side effects and optimizing efficacy, requiring innovations to ensure selective delivery. Balancing immune modulation without causing unintended reactions is a significant hurdle due to the complex immune system. Resistance mechanisms developed by cancer cells pose a challenge, necessitating continuous innovation to overcome evasion strategies. Concerns about nanoparticle toxicity to normal tissues must be carefully evaluated through comprehensive studies. Despite challenges, the future is optimistic. Advances in nanotechnology, materials science, and immunology may address limitations. Personalized nanoparticle designs based on individual patient and tumor profiles could enhance outcomes. Combining nanoparticle immunotherapies with traditional treatments or emerging therapies holds potential for synergistic effects. Smart nanoparticles capable of monitoring and responding to the dynamic tumor microenvironment could revolutionize cancer immunotherapy.
Knowledge gaps in nanoparticle-based immunotherapy of cancer exist mostly concerning the nanoparticle-immune system complex interactions, the design optimization for targeted delivery, and the evaluation of the long-term safety and efficacy of such nanoplatforms. These are being addressed by research into how different characteristics of nanoparticles like their size, shape, surface charge, and composition which determine the biodistribution, cellular uptake, and immune responses [123]. Advanced imaging and analytical techniques are underway to pursue nanoparticles in vivo and learn about their behaviour and interactions at the cellular and molecular levels. High-throughput screening and computational models will be used to optimize nanoparticle design by predicting formulations that will be most effective against specific types of cancers. The development of multi-functionalized nanoparticles that co-deliver several therapeutic molecules to enhance immune activation and target the tumor microenvironment has taken much effort. This effort could result in a long-term safety and efficacy record by exploring comprehensive preclinical studies and clinical trials for possible toxicity, immunogenicity, and nanoparticle-induced durable anti-tumour responses [124].
Clinical translation pathways and practical recommendations
If the innovative nanoparticle designs developed at the bench side have to find their way into clinical settings, more so in cancer immunotherapy, a holistic and systematic approach is the need of the hour. Several critical steps should be properly followed with a clearer roadmap for that [125]. First, identify and validate target molecules to be interacted with by nanoparticles, ensuring this interaction is specific and effective in preclinical models. This is followed by the synthesis and characterization of nanoparticles that ensure reproducibility, stability, and safety. Next, there needs to be rigorous testing at the preclinical level for pharmacokinetics, biodistribution, toxicity, and efficacy evaluations; most often, this involves an animal model. Contingent upon the preclinical results, the finalization of scalable and reproducible manufacturing processes to good manufacturing practice standards is quite necessary [126]. Dealing with case-by-case regulatory considerations, early and continued contact with agencies like the FDA helps align with requirements on safety and efficacy, often mandating an Investigational New Drug (IND) application. Clinical studies initiate with Phase I trials for safety, progress to Phases II and III for efficacy in larger patient populations, and monitor for adverse effects. Other practical considerations include the integration of nanoparticles into already existing treatment regimens, their co-compatibilities with the current cancer immunotherapies, and robust delivery so that nanoparticle systems do eventually target tumor sites effectively [127,128]. This would also entail an interplay of many disciplines like nanotechnology, oncology, pharmacology, regulatory science, etc. A patient-centred approach is essential to meet potential adverse economic and psychological repercussions. In the end, it is the translation of clinically viable nanoparticle-based therapies that ensure better treatment outcomes, all while safeguarding safety and efficacy which is the ultimate need of the hour in cancer immunotherapy.
Recent updates on nanoparticle-based therapies in the context of cancer immunotherapy give several actionable recommendations for researchers and clinicians. As some nanoparticle immunotherapies are currently facing specific challenges to achieve optimal outcomes, efforts must be dedicated to improving targeting specificity and reducing off-target effects, which are now achievable through state-of-the-art surface modification techniques and the application of biomimetic materials [129]. Nanoparticles made from biodegradable and biocompatible materials needs special attention in this regard. Tumour heterogeneity can be overcome by designing nanoparticles that would be able to target several tumor antigens simultaneously to enhance the effectiveness of immunotherapy [130]. If such a gap between laboratory findings and clinical applications is to be bridged by a clinician, rigorous standardized preclinical testing must be ensured with robust animal models that closely mimic human cancer biology. Clinicians have to remain vigilant about probable immunogenicity and long-term safety and carefully monitor and report possible adverse effects during the trials of nanomedicine in cancer immunotherapy. The selection of patients needs further refinement to identify individuals who are most likely to benefit from nanoparticle-based immunotherapy, possibly using biomarkers and genetic profiling. Further, the impediments that exist toward its wide application are financial and logistical barriers to the advocacy of supportive healthcare policy and access equity for these cutting-edge treatments. Attention to these recommendations will let scientists and clinicians improve the efficacy and safety of nanoparticle-based cancer immunotherapies, hence bringing improved outcomes for patients [131].
Potential side effects and safety concerns
While nanoparticle use in immunotherapy holds promise, caution is warranted due to potential side effects and safety concerns. An important issue is nanoparticle toxicity, especially with metal-based materials like gold or silver, which may accumulate in vital organs over time despite efforts to improve biocompatibility. Systematic toxicity assessments in preclinical and clinical studies are vital to ensure the safety parameters associated with nanoparticle use. Immunotoxicity is also a major concern to be taken care of. Nanoparticles can interact with the immune system, potentially causing unintended responses like excessive activation or suppression, leading to inflammatory reactions or increased infection susceptibility [132]. A detailed study of the immunomodulatory effects of nanoparticles is vital for designing safe immunotherapies. Navigating nanoparticle biodistribution and clearance is challenging, with the risk of off-target effects due to non-target tissue accumulation. Controlled nanoparticle distribution requires surface modifications and coatings to enhance tissue targeting and reduce interactions with healthy organs. Long-term effects and chronic exposure concerns arise due to nanoparticle persistence, emphasizing the need for extended safety assessments. Specific concerns surround liposomes, a common nanoparticle platform, regarding stability and potential immune responses. Iron oxide nanoparticles, used for imaging and therapy, raise worries about long-term safety, emphasizing the importance of addressing biodegradability and organ accumulation in their application [133].
Personalized approaches in nanoparticle-based immunotherapy
A pivotal element involves tailoring nanoparticle design to the molecular and genetic attributes of the patient’s tumor. For instance, engineered nanoparticles can carry antigens derived from the patient’s specific tumor cells, fostering a uniquely personalized immune response. By leveraging the distinct antigenic profile of individual tumors, this approach enhances the precision with which the immune system identifies and targets cancer cells [134]. Guiding personalized nanoparticle-based immunotherapy, genomic profiling analyzes a patient’s tumor genetics to identify specific targets for tailored interventions. Nanoparticles, precision-engineered, deliver therapeutic agents like siRNA or CRISPR-Cas9, targeting tumor-specific genetic vulnerabilities. This customization ensures a focused and efficient treatment approach [135]. Considering individual immune profiles, nanoparticles can be designed to modulate T cells, dendritic cells, and natural killer cells, enhancing their anti-tumor functions. For instance, nanoparticles may boost patient-derived T cells’ activation and expansion, improving their ability to eliminate cancer cells.
Emerging technologies and novel nanoparticle formulations
At the forefront of nanomedicine, new technologies and creative nanoparticle designs offer fresh paths for drug delivery and therapies. A notable advancement involves multifunctional nanoparticles that combine different features into one platform [136]. Theranostic nanoparticles are created nanoparticles that deliver drugs while also containing imaging agents, enabling real-time monitoring of treatment effectiveness and drug distribution. These multifunctional nanoparticles improve precision and accuracy in both diagnosis and treatment, providing a thorough and personalized approach to patient care. In oncology, theranostic nanoparticles epitomize radical application, delivering drugs to cancer cells while also carrying imaging agents for monitoring treatment responses non-invasively. This fruitful amalgamation of dual modalities allows clinicians to evaluate therapeutic effectiveness instantly, facilitating timely adjustments and personalized interventions based on each patient’s unique responses [137]. Nanoparticle gene delivery systems enable precise cellular function modulation, offering targeted gene expression regulation. They offer promise in treating genetic disorders, neurodegenerative diseases, cancers, and enhancing vaccine efficacy. Stimuli-responsive nanoparticles improve drug delivery accuracy, minimizing off-target effects. For instance, pH-sensitive nanoparticles can precisely release drugs in acidic tumor environments, improving drug delivery to cancer cells. Responsive nanoparticles enhance drug delivery precision, reducing side effects and improving therapeutic outcomes [138–140].
Emerging trends in cancer nano immunotherapy
Cancer nano immunotherapy revolutionizes the face of conventional cancer treatment through innovation-focused strategies in immune targeting and elimination of tumors. One such trend is multifunctional nanoparticles capable of antigen, adjuvant, and immunomodulatory drug concomitant delivery to optimize immune responses. These nanoparticles will achieve the repression of a highly immunosuppressive content in the tumor microenvironment through delivery of immune checkpoint inhibitors together with traditional chemotherapy drugs or other immune-activating agents, which enhance T-cell activation and reduce immune evasion in cancer cells. Other recent developments are also oriented towards integrating nanotechnology with personalized medicine. Researchers are working on designing nanoparticles that can be tailored to the genetic and immunological profiles of individual patients, allowing for more precise and effective treatments. Such nanoparticles may carry antigens unique to each patient or be specifically designed to modulate immune pathways that are unique to the patient’s tumor to enhance the outcome of therapy. Another area of active development involves biomimetic nanoparticles coated in cell membranes from cancer cells, immune cells, or platelets. Because of the coating, these can bypass the host immune system but find tumors. One of the interesting approaches using biomimetic nanoparticles is their direct delivery to the site of tumors without systemic toxicity, thus actively increasing the therapeutic agent accumulation by the tumor [141–143].
Nanoparticles capable of delivering genes or reprogramming proteins in vivo could thus offer a way to generate CAR-T cells straightforwardly within the patient and bypass the complex ex vivo manipulations. Improving the treatment process of such therapies reduces costs and broadens applicability to more patients and cancer indications. Another approach is the use of nanoparticles for imaging and monitoring immune response. Nanoparticles could be engineered to bear imaging agents that provide real-time feedback on distribution and activity of immune cells within the tumor microenvironment. That gives the capability to precisely monitor treatment responses and adjust therapy regimens based on real-time data, improving management and outcomes of cancer immunotherapy. Nanoparticles designed to disrupt the blood supply to tumors, function to enhance the delivery of immune cells and therapeutic agents into the tumor site, and facilitate a heightened immune response. The overall integration of nanotechnology into cancer immunotherapy points the way toward more effective, personalized, and acute therapy with reduced toxicity in cancer patients [144]. These emerging trends may greatly improve the outcome for patients and revolutionize the approach toward curing cancer in the light of advancing research.
Limitations of the current manuscript
Although our review of nanoparticles and their interaction with immunity for treating cancer was timely, several limitations are noted. First of all, although the review stipulated that it would present a critical overview of nanoparticle classes and their requirements to interact with immune cells, including macrophages, dendritic cells, T lymphocytes, and NK cells, we failed to provide in-depth information about the vast array of diverse nanoparticles available with specific characteristics. It is highly important to discuss the ways through which nanoparticles can influence immune cell functions, such as cytokine production and antigen presentation; however, there could be little or no regard for variability across different types of cancer and patient populations. This may carry over to the discussion of other regulatory mechanisms, signalling pathways, and nanoparticle characteristics that are important to mention for purposes of understanding but perhaps too general to provide meaningful guidance for both researchers and clinicians. Issues raised regarding nanoparticle-based immunotherapy include accurate cell targeting, modulation of the immune response, and toxicity; however, the review may have fallen short in fully addressing the details of how these challenges are to be overcome in practice or give concrete examples of how some strategies were successful. Further, though there is considerable hope held out in such more tailored approaches based on patient and tumor features from our article, it alone may not provide any clear view or guideline of its implementation toward the application of such strategies in clinical practice. It finally presents the development of emerging multifunctional and theranostic nanoparticles as a very promising route for future cancer therapies but perhaps falls a bit short in giving a clear roadmap on how to translate such innovation from research into the clinics. While our article is useful to provide an overview of the field, the discussions may be more useful for detailed reviews, specific examples, and practical recommendations. This information is critical to properly equip researchers and clinicians with all knowledge necessary for nanoparticle-based cancer immunotherapy.
In summary, the exploration of nanoparticles in immune cell modulation, particularly in cancer immunotherapy, reveals a diverse landscape of possibilities. The review highlights nanoparticles’ multifaceted impact on immune cells, influencing responses from antigen presentation to immune tolerance and evasion. This versatility offers transformative potential for personalized cancer treatment. While optimistic about revolutionizing cancer therapy, challenges remain, requiring in-depth research to understand nanoparticle-immune system interactions. The call for comprehensive investigations at preclinical and clinical levels is crucial for effective clinical translation. The review emphasizes nanoparticles’ remarkable impact on immune cells, signaling their potential to shape the future of cancer immunotherapy. Navigating this frontier requires sustained efforts, collaboration, and a commitment to unraveling the intricate interplay between nanoparticles and the immune system for the global benefit of cancer patients.
Acknowledgement: None.
Funding Statement: The authors received no specific funding for this study.
Author Contributions: The authors confirm their contribution to the paper as follows: study conception and design: Manu M. Joseph; draft manuscript preparation: Jyothi B. Nair, Anu Mary Joseph, Sanoop P.; review and editing: Manu M. Joseph, Jyothi B. Nair; visualization: Manu M. Joseph, Jyothi B. Nair; supervision: Manu M. Joseph. All authors reviewed the results and approved the final version of the manuscript.
Availability of Data and Materials: All data generated or analyzed during this study are included in this published article.
Ethics Approval: Not applicable.
Conflicts of Interest: The authors declare that they have no conflicts of interest to report regarding the present study.
References
1. Liu J, Liu Z, Pang Y, Zhou H. The interaction between nanoparticles and immune system: application in the treatment of inflammatory diseases. J Nanobiotechnology. 2022;20(1):127. doi:10.1186/s12951-022-01343-7. [Google Scholar] [PubMed] [CrossRef]
2. Dobrovolskaia MA, Shurin M, Shvedova AA. Current understanding of interactions between nanoparticles and the immune system. Toxicol Appl Pharmacol. 2016;29:78–89. [Google Scholar]
3. Zhang Y, Zhang Z. The history and advances in cancer immunotherapy: understanding the characteristics of tumour-infiltrating immune cells and their therapeutic implications. Cell Mol Immunol. 2020;17(8):807–21. doi:10.1038/s41423-020-0488-6. [Google Scholar] [PubMed] [CrossRef]
4. Abbott M, Ustoyev Y. Cancer and the immune system: the history and background of immunotherapy. Semin Oncol Nurs. 2019;35(5):150923. doi:10.1016/j.soncn.2019.08.002. [Google Scholar] [PubMed] [CrossRef]
5. Kottschade LA. The future of immunotherapy in the treatment of cancer. Semin Oncol Nurs. 2019;35(5):150934. doi:10.1016/j.soncn.2019.08.013. [Google Scholar] [PubMed] [CrossRef]
6. Corrales L, Matson V, Flood B, Spranger S, Gajewski TF. Innate immune signaling and regulation in cancer immunotherapy. Cell Res. 2017;27(1):96–108. doi:10.1038/cr.2016.149. [Google Scholar] [PubMed] [CrossRef]
7. Sterner RC, Sterner RM. CAR-T cell therapy: current limitations and potential strategies. Blood Cancer J. 2021;11(4):69. doi:10.1038/s41408-021-00459-7. [Google Scholar] [PubMed] [CrossRef]
8. Sanmamed MF, Chen L. A paradigm shift in cancer immunotherapy: from enhancement to normalization. Cell. 2018;175(2):313–26. doi:10.1016/j.cell.2018.09.035. [Google Scholar] [PubMed] [CrossRef]
9. Tian Y, Xie D, Yang L. Engineering strategies to enhance oncolytic viruses in cancer immunotherapy. Signal Transduct Target Ther. 2022;7(1):117. doi:10.1038/s41392-022-00951-x. [Google Scholar] [PubMed] [CrossRef]
10. Bhalla S, Laganà A. Artificial intelligence for precision oncology. Adv Exp Med Biol. 2022;1361:249–68. doi:10.1007/978-3-030-91836-1. [Google Scholar] [CrossRef]
11. Xie N, Shen G, Gao W, Huang Z, Huang C, Fu L. Neoantigens: promising targets for cancer therapy. Signal Transduct Target Ther. 2023;8(1):9. doi:10.1038/s41392-022-01270-x. [Google Scholar] [PubMed] [CrossRef]
12. Kiyotani K, Chan HT, Nakamura Y. Immunopharmacogenomics towards personalized cancer immunotherapy targeting neoantigens. Cancer Sci. 2018;109(3):542–9. doi:10.1111/cas.13498. [Google Scholar] [PubMed] [CrossRef]
13. Jardim DL, Goodman A, de Melo Gagliato D, Kurzrock R. The challenges of tumor mutational burden as an immunotherapy biomarker. Cancer Cell. 2021;39(2):154–73. doi:10.1016/j.ccell.2020.10.001. [Google Scholar] [PubMed] [CrossRef]
14. Lu Y, Yuan X, Wang M, He Z, Li H, Wang J, et al. Gut microbiota influence immunotherapy responses: mechanisms and therapeutic strategies. J Hematol Oncol. 2022;15(1):47. doi:10.1186/s13045-022-01273-9. [Google Scholar] [PubMed] [CrossRef]
15. Shi Y, Lei Y, Liu L, Zhang S, Wang W, Zhao J, et al. Integration of comprehensive genomic profiling, tumor mutational burden, and PD-L1 expression to identify novel biomarkers of immunotherapy in non-small cell lung cancer. Cancer Med. 2021;10(7):2216–31. doi:10.1002/cam4.3649. [Google Scholar] [PubMed] [CrossRef]
16. Baxevanis CN, Perez SA, Papamichail M. Cancer immunotherapy. Crit Rev Clin Lab Sci. 2009;46(4):167–89. doi:10.1080/10408360902937809. [Google Scholar] [PubMed] [CrossRef]
17. López Vázquez M, Du W, Kanaya N, Kitamura Y, Shah K. Next-generation immunotherapies for brain metastatic cancers. Trends Cancer. 2021;7(9):809–22. doi:10.1016/j.trecan.2021.02.003. [Google Scholar] [PubMed] [CrossRef]
18. Hamid O, Ismail R, Puzanov I. Intratumoral immunotherapy-update 2019. Oncologist. 2020;25(3):e423–38. doi:10.1634/theoncologist.2019-0438. [Google Scholar] [PubMed] [CrossRef]
19. Nasirmoghadas P, Mousakhani A, Behzad F, Beheshtkhoo N, Hassanzadeh A, Nikoo M, et al. Nanoparticles in cancer immunotherapies: an innovative strategy. Biotechnol Prog. 2021;37(2):e3070. doi:10.1002/btpr.3070. [Google Scholar] [PubMed] [CrossRef]
20. Saleh T, Shojaosadati SA. Multifunctional nanoparticles for cancer immunotherapy. Hum Vaccin Immunother. 2016;12(7):1863–75. [Google Scholar] [PubMed]
21. Saxena M, van der Burg SH, Melief CJM, Bhardwaj N. Therapeutic cancer vaccines. Nat Rev Cancer. 2021;21(6):360–78. doi:10.1038/s41568-021-00346-0. [Google Scholar] [PubMed] [CrossRef]
22. Han Q, Niu M, Wu Q, Zhong H. Real-time monitoring of nanoparticle-based therapeutics: a review. Curr Drug Metab. 2018;19(2):124–30. doi:10.2174/1389200219666180212113426. [Google Scholar] [PubMed] [CrossRef]
23. Li J, Xie L, Sang W, Li W, Wang G, Yan J, et al. A Metal-phenolic nanosensitizer performs hydrogen sulfide-reprogrammed oxygen metabolism for cancer radiotherapy intensification and immunogenicity. Angew Chem Int Ed Engl. 2022;61(18):e202200830. doi:10.1002/anie.v61.18. [Google Scholar] [CrossRef]
24. Jang H, Kim EH, Chi SG, Kim SH, Yang Y. Nanoparticles targeting innate immune cells in tumor microenvironment. Int J Mol Sci. 2021;22(18):10009. doi:10.3390/ijms221810009. [Google Scholar] [PubMed] [CrossRef]
25. Sujai PT, Joseph MM, Saranya G, Nair JB, Murali VP, Maiti KK. Surface charge modulates the internalization vs. penetration of gold nanoparticles: comprehensive scrutiny on monolayer cancer cells, multicellular spheroids and solid tumors by SERS modality. Nanoscale. 2020;12(13):6971–5. doi:10.1039/D0NR00809E. [Google Scholar] [PubMed] [CrossRef]
26. Kim S, Jang J, Kim H, Cho H, Lee K, Choi IH. The effects of silica nanoparticles in macrophage cells. Immune Netw. 2012;12(6):296–300. doi:10.4110/in.2012.12.6.296. [Google Scholar] [PubMed] [CrossRef]
27. Kononenko V, Narat M, Drobne D. Nanoparticle interaction with the immune system. Arh Hig Rada Toksikol. 2015;66(2):97–108. doi:10.1515/aiht-2015-66-2582. [Google Scholar] [PubMed] [CrossRef]
28. Wang C, Zhang Y, Dong Y. Lipid nanoparticle-mRNA formulations for therapeutic applications. Acc Chem Res. 2021;54(23):4283–93. doi:10.1021/acs.accounts.1c00550. [Google Scholar] [PubMed] [CrossRef]
29. Zhu X, Li C, Lu Y, Liu Y, Wan D, Zhu D, et al. Tumor microenvironment-activated therapeutic peptide-conjugated prodrug nanoparticles for enhanced tumor penetration and local T cell activation in the tumor microenvironment. Acta Biomater. 2021;119:337–48. doi:10.1016/j.actbio.2020.11.008. [Google Scholar] [PubMed] [CrossRef]
30. Guevara ML, Persano F, Persano S. Nano-immunotherapy: overcoming tumour immune evasion. Semin Cancer Biol. 2021;69(4):238–48. doi:10.1016/j.semcancer.2019.11.010. [Google Scholar] [PubMed] [CrossRef]
31. Kim M, Yoon HJ, Le C, Lee M, Park RW, Lee B, et al. Immune checkpoint-blocking nanocages cross the blood-brain barrier and impede brain tumor growth. ACS Biomater Sci Eng. 2024;10(1):575–87. doi:10.1021/acsbiomaterials.3c01200. [Google Scholar] [PubMed] [CrossRef]
32. Gupta J, Safdari HA, Hoque M. Nanoparticle mediated cancer immunotherapy. Semin Cancer Biol. 2021;69(80):307–24. doi:10.1016/j.semcancer.2020.03.015. [Google Scholar] [PubMed] [CrossRef]
33. Shi Y, Lammers T. Combining nanomedicine and immunotherapy. Acc Chem Res. 2019;52(6):1543–54. doi:10.1021/acs.accounts.9b00148. [Google Scholar] [PubMed] [CrossRef]
34. Vitor MT, Bergami-Santos PC, Barbuto JA, de la Torre LG. Cationic liposomes as non-viral vector for RNA delivery in cancer immunotherapy. Recent Pat Drug Deliv Formul. 2013;7(2):99–110. doi:10.2174/18722113113079990010. [Google Scholar] [PubMed] [CrossRef]
35. Sayour EJ, Mendez-Gomez HR, Mitchell DA. Cancer vaccine immunotherapy with RNA-loaded liposomes. Int J Mol Sci. 2018;19(10):2890. doi:10.3390/ijms19102890. [Google Scholar] [PubMed] [CrossRef]
36. Hu M, Zhang J, Kong L, Yu Y, Hu Q, Yang T, et al. Immunogenic hybrid nanovesicles of liposomes and tumor-derived nanovesicles for cancer immunochemotherapy. ACS Nano. 2021;15(2):3123–38. doi:10.1021/acsnano.0c09681. [Google Scholar] [PubMed] [CrossRef]
37. Yuba E. Liposome-based immunity-inducing systems for cancer immunotherapy. Molecular Immunol. 2018;98:8–12. doi:10.1016/j.molimm.2017.11.001. [Google Scholar] [PubMed] [CrossRef]
38. Yoshizaki Y, Yuba E, Sakaguchi N, Koiwai K, Harada A, Kono K. pH-sensitive polymer-modified liposome-based immunity-inducing system: effects of inclusion of cationic lipid and CpG-DNA. Biomaterials. 2017;141:272–83. doi:10.1016/j.biomaterials.2017.07.001. [Google Scholar] [PubMed] [CrossRef]
39. Guevara ML, Persano F, Persano S. Advances in lipid nanoparticles for mRNA-based cancer immunotherapy. Front Chem. 2020;8:589959. doi:10.3389/fchem.2020.589959. [Google Scholar] [PubMed] [CrossRef]
40. Short L, Holt RA, Cullis PR, Evgin L. Direct in vivo CAR T cell engineering. Trends Pharmacol. Sci. 2024;45(5):406–18. doi:10.1016/j.tips.2024.03.004. [Google Scholar] [PubMed] [CrossRef]
41. Zhang H, You X, Wang X, Cui L, Wang Z, Xu F, et al. Delivery of mRNA vaccine with a lipid-like material potentiates antitumor efficacy through Toll-like receptor 4 signalling. Proc Natl Acad Sci U S A. 2021;118(6):e2005191118. doi:10.1073/pnas.2005191118. [Google Scholar] [PubMed] [CrossRef]
42. Chen J, Ye Z, Huang C, Qiu M, Song D, Li Y, et al. Lipid nanoparticle-mediated lymph node-targeting delivery of mRNA cancer vaccine elicits robust CD8+ T cell response. Proc Natl Acad Sci U S A. 2022;119(34):e2207841119. doi:10.1073/pnas.2207841119. [Google Scholar] [PubMed] [CrossRef]
43. Zong Y, Lin Y, We T, Cheng Q. Lipid nanoparticle (LNP) enables mRNA delivery for cancer therapy. Adv Mater. 2023;35(51):e2303261. doi:10.1002/adma.202303261. [Google Scholar] [PubMed] [CrossRef]
44. Wan Z, Zheng R, Moharil P, Liu Y, Chen J, Sun R, et al. Polymeric micelles in cancer immunotherapy. Molecules. 2021;26(5):1220. doi:10.3390/molecules26051220. [Google Scholar] [PubMed] [CrossRef]
45. Lima BV, Oliveira MJ, Barbosa MA, Gonçalves RM, Castro F. Immunomodulatory potential of chitosan-based materials for cancer therapy: a systematic review of in vitro, in vivo and clinical studies. Biomaterials Sci. 2021;9(9):3209–27. doi:10.1039/D0BM01984D. [Google Scholar] [PubMed] [CrossRef]
46. Danhier F, Ansorena E, Silva JM, Coco R, Le Breton A, Préat V. PLGA-based nanoparticles: an overview of biomedical applications. J Control Release. 2012;161(2):505–22. doi:10.1016/j.jconrel.2012.01.043. [Google Scholar] [PubMed] [CrossRef]
47. Mudgal J, Mudgal PP, Kinra M, Raval R. Immunomodulatory role of chitosan-based nanoparticles and oligosaccharides in cyclophosphamide-treated mice. Scand J Immunol. 2019;89(4):e12749. doi:10.1111/sji.2019.89.issue-4. [Google Scholar] [CrossRef]
48. Xiao P, Wang J, Zhao Z, Liu X, Sun X, Wang D, et al. Engineering nanoscale artificial antigen-presenting cells by metabolic dendritic cell labeling to potentiate cancer immunotherapy. Nano Lett. 2021;21(5):2094–103. doi:10.1021/acs.nanolett.0c04783. [Google Scholar] [PubMed] [CrossRef]
49. Zarenezhad E, Kanaan MHG, Abdollah SS, Vakil MK, Marzi M, Mazarzaei A, et al. Metallic nanoparticles: their potential role in breast cancer immunotherapy via trained immunity provocation. Biomedicines. 2023;11(5):1245. doi:10.3390/biomedicines11051245. [Google Scholar] [PubMed] [CrossRef]
50. Mohapatra A, Sathiyamoorthy P, Park IK. Metallic nanoparticle-mediated immune cell regulation and advanced cancer immunotherapy. Pharmaceutics. 2021;13(11):1867. doi:10.3390/pharmaceutics13111867. [Google Scholar] [PubMed] [CrossRef]
51. He JS, Liu SJ, Zhang YR, Chu XD, Lin ZB, Zhao Z, et al. The application of and strategy for gold nanoparticles in cancer immunotherapy. Front Pharmacol. 2021;12:687399. doi:10.3389/fphar.2021.687399. [Google Scholar] [PubMed] [CrossRef]
52. Huang H, Liu R, Yang J, Dai J, Fan S, Pi J, et al. Gold nanoparticles: construction for drug delivery and application in cancer immunotherapy. Pharmaceutics. 2023;15(7):1868. doi:10.3390/pharmaceutics15071868. [Google Scholar] [PubMed] [CrossRef]
53. Zhang R, Qin X, Lu J, Xu H, Zhao S, Li X, et al. Chemodynamic/photothermal synergistic cancer immunotherapy based on yeast microcapsule-derived Au/Pt nanoparticles. ACS Appl Mater Interfaces. 2023;15(20):24134–48. doi:10.1021/acsami.3c02646. [Google Scholar] [PubMed] [CrossRef]
54. Hočevar S, Milošević A, Rodriguez-Lorenzo L, Ackermann-Hirschi L, Mottas I, Petri-Fink A, et al. Polymer-coated gold nanospheres do not impair the innate immune function of human B lymphocytes in vitro. ACS Nano. 2019;13(6):6790–800. doi:10.1021/acsnano.9b01492. [Google Scholar] [PubMed] [CrossRef]
55. Lin MH, Lin CF, Yang SC, Hung CF, Fang JY. The interplay between nanoparticles and neutrophils. J Biomed Nanotechnol. 2018;14(1):66–85. doi:10.1166/jbn.2018.2459. [Google Scholar] [PubMed] [CrossRef]
56. Gonçalves C, Torrado E, Martins T, Pereira P, Pedrosa J, Gama M. Dextrin nanoparticles: studies on the interaction with murine macrophages and blood clearance. Colloids Surf B, Biointerfaces. 2010;75(2):483–9. doi:10.1016/j.colsurfb.2009.09.024. [Google Scholar] [PubMed] [CrossRef]
57. Zolnik BS, González-Fernández A, Sadrieh N, Dobrovolskaia MA. Nanoparticles and the immune system. Endocrinology. 2010;151(2):458–65. doi:10.1210/en.2009-1082. [Google Scholar] [PubMed] [CrossRef]
58. Bamberger D, Hobernik D, Konhäuser M, Bros M, Wich PR. Surface modification of polysaccharide-based nanoparticles with PEG and dextran and the effects on immune cell binding and stimulatory characteristics. Mol Pharm. 2017;14(12):4403–16. doi:10.1021/acs.molpharmaceut.7b00507. [Google Scholar] [PubMed] [CrossRef]
59. Zhang YN, Lazarovits J, Poon W, Ouyang B, Nguyen LNM, Kingston BR, et al. Nanoparticle size influences antigen retention and presentation in lymph node follicles for humoral immunity. Nano Lett. 2019;19(10):7226–35. doi:10.1021/acs.nanolett.9b02834. [Google Scholar] [PubMed] [CrossRef]
60. Weisheit CK, Kurts Engel DR, CDendritic cells and macrophages: sentinels in the kidney. Clin J Am Soc Nephrol. 2015;10(10):1841–51. doi:10.2215/CJN.07100714. [Google Scholar] [PubMed] [CrossRef]
61. Borasch D, Italiani P, Palomba R, Decuzzi P, Duschl A, Fadeel B, et al. Nanoparticles and innate immunity: new perspectives on host defence. Semin Immunol. 2017;34:33–51. doi:10.1016/j.smim.2017.08.013. [Google Scholar] [PubMed] [CrossRef]
62. He Y, Wang M, Li X, Yu T, Gao X. Targeted MIP-3β plasmid nanoparticles induce dendritic cell maturation and inhibit M2 macrophage polarisation to suppress cancer growth. Biomaterials. 2020;249(7378):120046. doi:10.1016/j.biomaterials.2020.120046. [Google Scholar] [PubMed] [CrossRef]
63. Nagy NA, de Haas AM, Geijtenbeek TBH, van Ree R, Tas SW, van Kooyk Y, et al. Therapeutic liposomal vaccines for dendritic cell activation or tolerance. Front Immunol. 2021;12:674048. doi:10.3389/fimmu.2021.674048. [Google Scholar] [PubMed] [CrossRef]
64. Zong S, Li J, Ye Z, Zhang X, Yang L, Chen X, et al. Lachnum polysaccharide suppresses S180 sarcoma by boosting anti-tumor immune responses and skewing tumor-associated macrophages toward M1 phenotype. Int J Biol Macromol. 2020;144:1022–33. doi:10.1016/j.ijbiomac.2019.09.179. [Google Scholar] [PubMed] [CrossRef]
65. Kyrysyuk O, Wucherpfennig KW. Designing cancer immunotherapies that engage T cells and NK cells. Annu Rev Immunol. 2023;41(1):17–38. doi:10.1146/annurev-immunol-101921-044122. [Google Scholar] [PubMed] [CrossRef]
66. Sivori S, Vacca P, Del Zotto G, Munari E, Mingari MC, Moretta L. Human NK cells: surface receptors, inhibitory checkpoints, and translational applications. Cell Mol Immunol. 2019;16(5):430–41. doi:10.1038/s41423-019-0206-4. [Google Scholar] [PubMed] [CrossRef]
67. Malysheva A, Ivask A, Doolette CL, Voelcker NH, Lombi E. Cellular binding, uptake and biotransformation of silver nanoparticles in human T lymphocytes. Nat Nanotechnol. 2021;16(8):926–32. doi:10.1038/s41565-021-00914-3. [Google Scholar] [PubMed] [CrossRef]
68. Jiang D, Gao T, Liang S, Mu W, Fu S, Liu Y, et al. Lymph node delivery strategy enables the activation of cytotoxic T lymphocytes and natural killer cells to augment cancer immunotherapy. ACS Appl Mater Interfaces. 2021;13(19):22213–24. doi:10.1021/acsami.1c03709. [Google Scholar] [PubMed] [CrossRef]
69. Raker VK, Becker C, Landfester K, Steinbrink K. Targeted activation of T cells with IL-2-coupled nanoparticles. Cells. 2020;9(9):2063. doi:10.3390/cells9092063. [Google Scholar] [PubMed] [CrossRef]
70. McCarthy DP, Hunter ZN, Chackerian B, Shea LD, Miller SD. Targeted immunomodulation using antigen-conjugated nanoparticles. Wiley Interdiscip Rev Nanomed Nanobiotechnol. 2014;6(3):298–315. doi:10.1002/wnan.1263. [Google Scholar] [PubMed] [CrossRef]
71. Niu Y, Tang M. In vitro review of nanoparticles attacking macrophages: interaction and cell death. Life Sci. 2022;307:120840. doi:10.1016/j.lfs.2022.120840. [Google Scholar] [PubMed] [CrossRef]
72. Hu Y, Zhang W, Chu X, Wang A, He Z, Si CL, et al. Dendritic cell-targeting polymer nanoparticle-based immunotherapy for cancer: a review. Int J Pharm. 2023;635:122703. doi:10.1016/j.ijpharm.2023.122703. [Google Scholar] [PubMed] [CrossRef]
73. Miao X, Leng XF, Zhang Q. The current state of nanoparticle-induced macrophage polarization and reprogramming research. Int J Mol Sci. 2017;18(2):336. doi:10.3390/ijms18020336. [Google Scholar] [PubMed] [CrossRef]
74. Chen W, Zhang Q, Kaplan BL, Baker GL, Kaminski NE. Induced T cell cytokine production is enhanced by engineered nanoparticles. Nanotoxicol. 2014;1:11–23. [Google Scholar]
75. Napierska D, Thomassen LC, Vanaudenaerde B, Luyts K, Lison D, Martens JA, et al. Cytokine production by co-cultures exposed to monodisperse amorphous silica nanoparticles: the role of size and surface area. Toxicol Lett. 2012;211(2):98–104. doi:10.1016/j.toxlet.2012.03.002. [Google Scholar] [PubMed] [CrossRef]
76. Patidar M, Yadav N, Dalai SK. Interleukin 15: a key cytokine for immunotherapy. Cytokine Growth Factor Rev. 2016;31:49–59. doi:10.1016/j.cytogfr.2016.06.001. [Google Scholar] [PubMed] [CrossRef]
77. Qiu Y, Su M, Liu L, Tang Y, Pan Y, Sun J. Clinical application of cytokines in cancer immunotherapy. Drug Des Devel Ther. 2021;15:2269–87. doi:10.2147/DDDT.S308578. [Google Scholar] [PubMed] [CrossRef]
78. Jhunjhunwala S, Hammer C, Delamarre L. Antigen presentation in cancer: insights into tumour immunogenicity and immune evasion. Nat Rev Cancer. 2021;21(5):298–312. doi:10.1038/s41568-021-00339-z. [Google Scholar] [PubMed] [CrossRef]
79. Reeves E, James E. Antigen processing and immune regulation in the response to tumours. Immunol. 2017;150(1):16–24. doi:10.1111/imm.2017.150.issue-1. [Google Scholar] [CrossRef]
80. Irvine DJ, Dane EL. Enhancing cancer immunotherapy with nanomedicine. Nat Rev Immunol. 2020;20(5):321–34. doi:10.1038/s41577-019-0269-6. [Google Scholar] [PubMed] [CrossRef]
81. Li F, Li F, Urie R, Bealer E, Ruiz RO, Saito E, et al. Membrane-coated nanoparticles for direct recognition by T cells. Biotechnol Bioeng. 2023;120(3):767–77. doi:10.1002/bit.v120.3. [Google Scholar] [CrossRef]
82. Jia J, Zhang Y, Xin Y, Jiang C, Yan B, Zhai S. Interactions between nanoparticles and dendritic cells: from the perspective of cancer immunotherapy. Front Oncol. 2018;8:404. doi:10.3389/fonc.2018.00404. [Google Scholar] [PubMed] [CrossRef]
83. Sun Q, Ojha T, Kiessling F, Lammers T, Shi Y. Enhancing tumor penetration of nanomedicines. Biomacromol. 2017;18(5):1449–59. doi:10.1021/acs.biomac.7b00068. [Google Scholar] [PubMed] [CrossRef]
84. Zhang YR, Lin R, Li HJ, He WL, Du JZ, Wang J. Strategies to improve tumor penetration of nanomedicines through nanoparticle design. Wiley Interdiscip Rev Nanomed Nanobiotechnol. 2019;11(1):e1519. doi:10.1002/wnan.2019.11.issue-1. [Google Scholar] [CrossRef]
85. Wang M, Thanou M. Targeting nanoparticles to cancer. Pharmacol Res. 2010;62(2):90–9. doi:10.1016/j.phrs.2010.03.005. [Google Scholar] [PubMed] [CrossRef]
86. Eloy JO, Petrilli R, Trevizan LNF, Chorilli M. Immunoliposomes: a review on functionalization strategies and targets for drug delivery. Colloids Surf B Biointerfaces. 2017;159:454–67. doi:10.1016/j.colsurfb.2017.07.085. [Google Scholar] [PubMed] [CrossRef]
87. Choi Y, Kim J, Chae J, Hong J, Park J, Jeong E, et al. Surface glycan targeting for cancer nano-immunotherapy. J Control Release. 2022;34:321–36. [Google Scholar]
88. Yang K, Halima A, Chan TA. Antigen presentation in cancer - mechanisms and clinical implications for immunotherapy. Nat Rev Clin Oncol. 2023;20(9):604–23. doi:10.1038/s41571-023-00789-4. [Google Scholar] [PubMed] [CrossRef]
89. Est-Witte SE, Livingston NK, Omotoso MO, Green JJ, Schneck JP. Nanoparticles for generating antigen-specific T cells for immunotherapy. Semin Immunol. 2021;56:101541. doi:10.1016/j.smim.2021.101541. [Google Scholar] [PubMed] [CrossRef]
90. Wang N, Zhang G, Sun H, Tian Y, Hao J, Cui J. Vaccine nanoparticles derived from mung beans for cancer immunotherapy. Chem Mater. 2021;33(11):4057–66. doi:10.1021/acs.chemmater.1c00629. [Google Scholar] [CrossRef]
91. Wang Z, Wu Z, Liu Y, Han W. New development in CAR-T cell therapy. J Hematol Oncol. 2017;10(1):53. doi:10.1186/s13045-017-0423-1. [Google Scholar] [PubMed] [CrossRef]
92. Yao H, Shen N, Ji G, Huang J, Sun J, Wang G, et al. Cisplatin nanoparticles promote intratumoral CD8+ T cell priming via antigen presentation and T cell receptor crosstalk. Nano Lett. 2022;22(8):3328–39. doi:10.1021/acs.nanolett.2c00478. [Google Scholar] [PubMed] [CrossRef]
93. Harvey BT, Fu X, Li L, Neupane KR, Anand N, Kolesar JM, et al. Dendritic cell membrane-derived nanovesicles for targeted T cell activation. ACS Omega. 2022;7(50):46222–33. doi:10.1021/acsomega.2c04420. [Google Scholar] [PubMed] [CrossRef]
94. Alhallak K, Sun J, Wasden K, Guenthner N, O’Neal J, Muz B, et al. Nanoparticle T-cell engagers as a modular platform for cancer immunotherapy. Leukemia. 2021;35(8):2346–57. doi:10.1038/s41375-021-01127-2. [Google Scholar] [PubMed] [CrossRef]
95. Dong N, Liu Z, He H, Lu Y, Qi J, Wu W. “Hook&Loop” multivalent interactions based on disk-shaped nanoparticles strengthen active targeting. J Control Release. 2023;354:279–93. doi:10.1016/j.jconrel.2023.01.022. [Google Scholar] [PubMed] [CrossRef]
96. Zhang W, Gong C, Chen Z, Li M, Li Y, Gao J. Tumor microenvironment-activated cancer cell membrane-liposome hybrid nanoparticle-mediated synergistic metabolic therapy and chemotherapy for non-small cell lung cancer. J Nanobiotechnology. 2021;19(1):339. doi:10.1186/s12951-021-01085-y. [Google Scholar] [PubMed] [CrossRef]
97. Wei B, Pan J, Yuan R, Shao B, Wang Y, Guo X, et al. Polarization of tumor-associated macrophages by nanoparticle-loaded Escherichia coli combined with immunogenic cell death for cancer immunotherapy. Nano Lett. 2021;21(10):4231–40. doi:10.1021/acs.nanolett.1c00209. [Google Scholar] [PubMed] [CrossRef]
98. Dougan M, Dougan SK. Targeting immunotherapy to the tumor microenvironment. J Cell Biochem. 2017;118(10):3049–54. doi:10.1002/jcb.v118.10. [Google Scholar] [CrossRef]
99. Zhao H, Li Y, Shi H, Niu M, Li D, Zhang Z, et al. Prodrug nanoparticles potentiate tumor chemo-immunometabolic therapy by disturbing oxidative stress. J Control Release. 2022;352:909–19. doi:10.1016/j.jconrel.2022.11.011. [Google Scholar] [PubMed] [CrossRef]
100. Zheng R, Ma J. Immunotherapeutic implications of toll-like receptors activation in tumor microenvironment. Pharmaceutics. 2022;14(11):2285. doi:10.3390/pharmaceutics14112285. [Google Scholar] [PubMed] [CrossRef]
101. Poon W, Kingston BR, Ouyang B, Ngo W, Chan WCW. A framework for designing delivery systems. Nature Nanotechnol. 2020;15(10):819–29. doi:10.1038/s41565-020-0759-5. [Google Scholar] [PubMed] [CrossRef]
102. Escors D. Tumour immunogenicity, antigen presentation and immunological barriers in cancer immunotherapy. N J Sci. 2014;2014:734515. [Google Scholar]
103. Reisländer T, Groelly FJ, Tarsounas M. DNA damage and cancer immunotherapy: a STING in the tale. Mol Cell. 2020;80(1):21–8. doi:10.1016/j.molcel.2020.07.026. [Google Scholar] [PubMed] [CrossRef]
104. Alzahrani AS. PI3K/Akt/mTOR inhibitors in cancer: at the bench and bedside. Semin Cancer Biol. 2019;59:125–32. doi:10.1016/j.semcancer.2019.07.009. [Google Scholar] [PubMed] [CrossRef]
105. Waldmann TA, Chen J. Disorders of the JAK/STAT pathway in T cell lymphoma pathogenesis: implications for immunotherapy. Annu Rev Immunol. 2017;35:533–50. doi:10.1146/immunol.2017.35.issue-1. [Google Scholar] [CrossRef]
106. Tran TH, Tran TTP, Truong DH, Nguyen HT, Pham TT, Yong CS, et al. Toll-like receptor-targeted particles: a paradigm to manipulate the tumor microenvironment for cancer immunotherapy. Acta Biomater. 2019;94:82–96. doi:10.1016/j.actbio.2019.05.043. [Google Scholar] [PubMed] [CrossRef]
107. Gliga AR, De Loma J, Di Bucchianico S, Skoglund S, Keshavan S, Odnevall Wallinder I, et al. Silver nanoparticles modulate lipopolysaccharide-triggered toll-like receptor signaling in immune-competent human cell lines. Nanoscale Adv. 2020;2(2):648–58. doi:10.1039/C9NA00721K. [Google Scholar] [PubMed] [CrossRef]
108. Wang X, Pham A, Kang L, Walker SA, Davidovich I, Iannotta D, et al. Effects of adipose-derved biogenic nanoparticle-associated microRNA-451a on toll-like receptor 4-induced cytokines. Pharmaceutics. 2021;14(1):16. doi:10.3390/pharmaceutics14010016. [Google Scholar] [PubMed] [CrossRef]
109. Sun SC. The noncanonical NF-κB pathway. Immunol Rev. 2021;246(1):125–40. [Google Scholar]
110. Uto T, Wang X, Sato K, Haraguchi M, Akagi T, Akashi M, et al. Targeting of antigen to dendritic cells with poly(γ-glutamic acid) nanoparticles induces antigen-specific humoral and cellular immunity. J Immunol. 2007;178(5):2979–86. doi:10.4049/jimmunol.178.5.2979. [Google Scholar] [PubMed] [CrossRef]
111. Wang J, Wang L, Zhao W, Yu N, Cheng M, Su M, et al. The role of apoptosis pathway in the cytotoxicity induced by fresh and aged zinc oxide nanoparticles. Nanoscale Res Lett. 2021;16(1):129. doi:10.1186/s11671-021-03587-y. [Google Scholar] [PubMed] [CrossRef]
112. Siegrist S, Kettiger H, Fasler-Kan E, Huwyler J. Selective stimulation of the JAK/STAT signaling pathway by silica nanoparticles in human endothelial cells. Toxicol in Vitro. 2017;42:308–18. doi:10.1016/j.tiv.2017.05.002. [Google Scholar] [PubMed] [CrossRef]
113. Liu X, Li H, Jin Q, Ji J. Surface tailoring of nanoparticles via mixed-charge monolayers and their biomedical applications. Small. 2014;10(21):4230–42. doi:10.1002/smll.v10.21. [Google Scholar] [CrossRef]
114. Ngobili TA, Daniele MA. Nanoparticles and direct immunosuppression. Exp Biol Med. 2016;241(10):1064–73. doi:10.1177/1535370216650053. [Google Scholar] [PubMed] [CrossRef]
115. Said SS, Ibrahim WN. Cancer resistance to immunotherapy: comprehensive insights with future perspectives. Pharmaceutics. 2023;15(4):1143. doi:10.3390/pharmaceutics15041143. [Google Scholar] [PubMed] [CrossRef]
116. Gillette JS, Wang EJ, Dowd RS, Toms SA. Barriers to overcoming immunotherapy resistance in glioblastoma. Front Med. 2023;10:1175507. doi:10.3389/fmed.2023.1175507. [Google Scholar] [PubMed] [CrossRef]
117. Sharma P, Hu-Lieskovan S, Wargo JA, Ribas A. Primary, adaptive, and acquired resistance to cancer immunotherapy. Cell. 2017;168(4):707–23. doi:10.1016/j.cell.2017.01.017. [Google Scholar] [PubMed] [CrossRef]
118. Mundekkad D, Cho WC. Nanoparticles in clinical translation for cancer therapy. Int J Mol Sci. 2022;23(3):1685. doi:10.3390/ijms23031685. [Google Scholar] [PubMed] [CrossRef]
119. Li H, Yang YG, Sun T. Nanoparticle-based drug delivery systems for induction of tolerance and treatment of autoimmune diseases. Front Bioeng Biotechnol. 2022;10:889291. doi:10.3389/fbioe.2022.889291. [Google Scholar] [PubMed] [CrossRef]
120. Koerner J, Horvath D, Herrmann VL, MacKerracher A, Gander B, Yagita H, et al. PLGA-particle vaccine carrying TLR3/RIG-I ligand Riboxxim synergizes with immune checkpoint blockade for effective anti-cancer immunotherapy. Nat Commun. 2021;12(1):2935. doi:10.1038/s41467-021-23244-3. [Google Scholar] [PubMed] [CrossRef]
121. Kim H, Baek Y, Ha T, Cho D, Lee WJ, Cho Y, et al. Gold nanoparticle-carrying T cells for the combined immuno-photothermal therapy. Small. 2023;19(47):e2301377. doi:10.1002/smll.v19.47. [Google Scholar] [CrossRef]
122. Moon Y, Shim MK, Choi J, Yang S, Kim J, Yun WS, et al. Anti-PD-L1 peptide-conjugated prodrug nanoparticles for targeted cancer immunotherapy combining PD-L1 blockade with immunogenic cell death. Theranostics. 2022;12(5):1999–2014. doi:10.7150/thno.69119. [Google Scholar] [PubMed] [CrossRef]
123. Feng R, Yu F, Xu J, Hu X. Knowledge gaps in immune response and immunotherapy involving nanomaterials: databases and artificial intelligence for material design. Biomaterials. 2021;266:120469. doi:10.1016/j.biomaterials.2020.120469. [Google Scholar] [PubMed] [CrossRef]
124. Johnston H, Brown D, Kermanizadeh A, Gubbins E, Stone V. Investigating the relationship between nanomaterial hazard and physicochemical properties: informing the exploitation of nanomaterials within therapeutic and diagnostic applications. J Control Release. 2012;164(3):307–13. doi:10.1016/j.jconrel.2012.08.018. [Google Scholar] [PubMed] [CrossRef]
125. Fan D, Cao Y, Cao M, Wang Y, Cao Y, Gong T. Nanomedicine in cancer therapy. Signal Transduction Targeted Ther. 2023;8(1):293. doi:10.1038/s41392-023-01536-y. [Google Scholar] [PubMed] [CrossRef]
126. Pricl S. The spicy science of dendrimers in the realm of cancer nanomedicine: a report from the COST Action CA17140 Nano2Clinic. Pharmaceutics. 2023;15(7):2013. doi:10.3390/pharmaceutics15072013. [Google Scholar] [PubMed] [CrossRef]
127. Bobo D, Robinson KJ, Islam J, Thurecht KJ, Corrie SR. Nanoparticle-based medicines: a review of FDA-approved materials and clinical trials to date. Pharm Res. 2016;33(10):2373–87. doi:10.1007/s11095-016-1958-5. [Google Scholar] [PubMed] [CrossRef]
128. Lytton-Jean AK, Kauffman KJ, Kaczmarek JC, Langer R. Cancer nanotherapeutics in clinical trials. Cancer Treat Res. 2015;166:293–322. doi:10.1007/978-3-319-16555-4. [Google Scholar] [CrossRef]
129. Zeng L, Gowda BHJ, Ahmed MG, Abourehab MAS, Chen ZS, Zhang C, et al. Advancements in nanoparticle-based treatment approaches for skin cancer therapy. Mol Cancer. 2023;22(1):10. doi:10.1186/s12943-022-01708-4. [Google Scholar] [PubMed] [CrossRef]
130. Zhang J, Wang S, Zhang D, He X, Wang X, Han H, et al. Nanoparticle-based drug delivery systems to enhance cancer immunotherapy in solid tumors. Front Immunol. 2023;14:1230893. doi:10.3389/fimmu.2023.1230893. [Google Scholar] [PubMed] [CrossRef]
131. Zhou L, Zou M, Xu Y, Lin P, Lei C, Xia X. Nano drug delivery system for tumor immunotherapy: next-generation therapeutics. Fron Oncol. 2022;12:864301. doi:10.3389/fonc.2022.864301. [Google Scholar] [PubMed] [CrossRef]
132. Pallardy MJ, Turbica I, Biola-Vidamment A. Why the immune system should be concerned by nanomaterials? Front Immunol. 2017;8:544. doi:10.3389/fimmu.2017.00544. [Google Scholar] [PubMed] [CrossRef]
133. Singh R, Kumawat M, Gogoi H, Madhyastha H, Lichtfouse E, Daima HK. Engineered nanomaterials for immunomodulation: a review. ACS Appl Bio Mater. 2024;7(2):727–51. doi:10.1021/acsabm.3c00940. [Google Scholar] [PubMed] [CrossRef]
134. Aikins ME, Xu C, Moon JJ. Engineered nanoparticles for cancer vaccination and immunotherapy. Acc Chem Res. 2020;53(10):2094–105. doi:10.1021/acs.accounts.0c00456. [Google Scholar] [PubMed] [CrossRef]
135. Li S, Bennett ZT, Sumer BD, Gao J. Nano-immune-engineering approaches to advance cancer immunotherapy: lessons from ultra-pH-sensitive nanoparticles. Acc Chem Res. 2020;53(11):2546–57. doi:10.1021/acs.accounts.0c00475. [Google Scholar] [PubMed] [CrossRef]
136. Larrañeta E, McCrudden MT, Courtenay AJ, Donnelly RF. Microneedles: a new frontier in nanomedicine delivery. Pharm Res. 2016;33(5):1055–73. doi:10.1007/s11095-016-1885-5. [Google Scholar] [PubMed] [CrossRef]
137. Rawding PA, Bu JY, Wang J, Kim DW, Drelich AJ, Kim Y, et al. Dendrimers for cancer immunotherapy: avidity-based drug delivery vehicles for effective anti-tumor immune response. Wiley Interdiscip Rev Nanomed Nanobiotechnol. 2022;14(2):e1752. doi:10.1002/wnan.v14.2. [Google Scholar] [CrossRef]
138. Yang J, Zhang C. Regulation of cancer-immunity cycle and tumor microenvironment by nanobiomaterials to enhance tumor immunotherapy. Wiley Interdiscip Rev Wiley Interdiscip Rev Nanomed Nanobiotechnol. 2020;12(4):e1612. doi:10.1002/wnan.v12.4. [Google Scholar] [CrossRef]
139. Hirobe S, Okada N, Nakagawa S. Transcutaneous vaccines--current and emerging strategies. Expert Opin Drug Deliv. 2013;10(4):485–98. doi:10.1517/17425247.2013.760542. [Google Scholar] [PubMed] [CrossRef]
140. Bussio JI, Molina-Perea C, González-Aramundiz JV. Lower-sized chitosan nanocapsules for transcutaneous antigen delivery. Nanomater. 2018;8(9):659. doi:10.3390/nano8090659. [Google Scholar] [PubMed] [CrossRef]
141. Kandasamy G, Karuppasamy Y, Krishnan UM. Emerging trends in nano-driven immunotherapy for the treatment of cancer. Vaccines. 2023;11(2):458. doi:10.3390/vaccines11020458. [Google Scholar] [PubMed] [CrossRef]
142. Venkatesan S, Chanda K, Balamurali MM. Recent advancements of aptamers in cancer therapy. ACS Omega. 2023;8(36):32231–43. doi:10.1021/acsomega.3c04345. [Google Scholar] [PubMed] [CrossRef]
143. Gowd V, Ahmad A, Tarique M, Suhail M, Zughaibi TA, Tabrez S, et al. Advancement of cancer immunotherapy using nanoparticles-based nanomedicine. Semin Cancer Biol. 2022;86(4):624–44. doi:10.1016/j.semcancer.2022.03.026. [Google Scholar] [PubMed] [CrossRef]
144. Buabeid MA, Arafa ESA, Murtaza G. Emerging prospects for nanoparticle-enabled cancer immunotherapy. J Immunol Res. 2020;2020:9624532. doi:10.1155/2020/9624532. [Google Scholar] [PubMed] [CrossRef]
Cite This Article
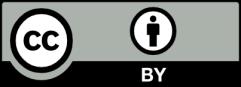
This work is licensed under a Creative Commons Attribution 4.0 International License , which permits unrestricted use, distribution, and reproduction in any medium, provided the original work is properly cited.