Open Access
REVIEW
The diverse functions and therapeutic implications of cancer-associated fibroblasts in colorectal cancer
1 Department of Pathology, Key Laboratory of Disease Proteomics of Zhejiang Province, School of Medicine, Zhejiang University, Hangzhou, 310058, China
2 Department of Pathology, Sir Run Run Shaw Hospital, Zhejiang University School of Medicine, Hangzhou, 310018, China
* Corresponding Author: HONG DENG. Email:
(This article belongs to the Special Issue: Extracellular Matrix in Development and Disease)
BIOCELL 2024, 48(11), 1569-1578. https://doi.org/10.32604/biocell.2024.053983
Received 02 March 2024; Accepted 21 June 2024; Issue published 07 November 2024
Abstract
In the development of colorectal cancer (CRC), cancer-associated fibroblasts (CAFs) play a pivotal role in establishing tumor-permissive extracellular matrix structures, angiogenesis, and modulating the immune status of the tumor microenvironment (TME), thereby influencing tumor metastasis and resistance to radiotherapy and chemotherapy. The pleiotropic effects of CAFs in the TME may be attributed to the heterogeneous origin and high plasticity of their population. Given the specificity of CAFs, they provide a variety of potential target molecules for future CRC treatment, which may play an indispensable role in CRC therapeutic strategies. This review summarizes the origin of CAFs and their roles in the CRC tumor microenvironment, including the interaction between exosomes and CAFs in CRC. Additionally, we discuss potential therapeutic strategies targeting CAFs.Keywords
Abbreviations
5-FU | 5-Fluorouracil |
ACTA2 | Actin alpha 2 |
AOM | Azoxymethane |
BMP4 | Bone morphogenetic protein 4 |
BMP5 | Bone morphogenetic protein 5 |
C1QC | Complement C1q C chain |
CAC | Colitis-associated cancer |
CAF | Cancer-associated fibroblast |
CAFs-exo | Cancer-associated fibroblast-derived exosome |
CCL2 | C-C motif chemokine ligand 2 |
CELF2 | CUGBP elav-like family member 2 |
CRKL | CRK like proto-oncogene: adaptor protein |
CXCL1 | C-X-C motif chemokine ligand 1 |
CRC | Colorectal cancer |
CTSH | Cathepsin H |
DSS | Dextran sodium sulfate |
ECM | Extracellular matrix |
EMT | Epithelial-to-mesenchymal transition |
FAP | Fibroblast activation protein |
FBXW7 | F-box and WD repeat domain-containing 7 |
FXR | Farnesoid X receptor |
HGF | Hepatocyte growth factor |
IAF | Inflammatory associated fibroblasts |
ICB | Immune checkpoint blockade |
ICRG | Immune- and CAF-related gene |
IL-1 | Interleukin-1 |
KLF5 | Krüppel like factor 5 |
KRAS | Kirsten rats arcomaviral oncogene homolog |
L-OHP | Oxaliplatin |
Lepr | Leptin receptor |
lncRNA | Long non-coding ribonucleic acid |
lnc-FAL1 | Focally amplified lncRNA on chromosome 1 |
LOX | Lysyl oxidases |
MCAM | Melanoma cell adhesion molecule |
MDSC | Myeloid-derived suppressor cell |
MERFISH | Multiplexed error-robust fluorescence in situ hybridization |
MFAP | Microfibrillar-associated protein |
MOAP1 | Modulator of apoptosis 1 |
MMP | Metalloproteinase |
MSC | Mesenchymal stem cell |
MYL9 | Myosin light chain 9 |
NF-κB | Nuclear factor kappa B |
NK cell | Natural killer cell |
OXA | Oxaliplatin |
PDGFA | Platelet derived growth factor subunit A |
PDGFRA | Platelet derived growth factor receptor A |
POSTN | Periostin |
PTEN | Phosphatase and tensin homolog |
RNA | Ribonucleic acid |
ROS | Reactive oxygen species |
TAGLN | Transgelin |
TAZ | Transcriptional co-activator with PDZ-binding motif |
TFCP2 | Transcription factor CP2 |
TGF-β | Transforming growth factor-β |
TLR | Toll-like receptor |
TME | Tumor microenvironment |
TNF | Tumour necrosis factor |
Treg | Regulatory T cell |
TRIM3 | Tripartite motif containing 3 |
VEGF | Vascular endothelial growth factor |
WNT5B | Wnt family member 5B |
WWOX | WW domain-containing oxidoreductase |
YAP | Yes-associated protein |
yCRC | Young-onset colorectal cancer |
Colorectal cancer (CRC) is one of the most common cancers globally [1], often accompanied by desmoplasia during its advancement. This pathological process is marked by the proliferation and accumulation of fibrous tissue, often predictive of unfavorable disease outcomes and therapeutic response [2–4]. The desmoplastic reaction consists mainly of activated fibroblasts, also called cancer-associated fibroblasts (CAFs). In the complex and dynamic milieu of tumor microenvironment (TME), CAFs occupy a pivotal position [5]. These specialized stromal cells, originating from a heterogeneous population with high plasticity, play a multifaceted role in tumor progression. Since Rudolf L.K. Virchow’s initial observations of CAF, the function and role of fibroblasts have been extensively investigated, particularly in the context of cancer. In CRC, CAFs are not merely passive bystanders, rather, they actively contribute to the establishment of tumor-permissive extracellular matrix structures, angiogenesis, and modulation of the immune status within the TME. The significance of CAFs in CRC is underscored by their involvement in key processes such as tumor metastasis and resistance to radiotherapy and chemotherapy. This intricate interplay between CAFs and cancer cells within the TME represents a novel and promising target for therapeutic intervention. The pleiotropic effects of CAFs are attributed to their diverse origins, including the activation of quiescent fibroblasts and other stromal cell populations [6]. The resulting heterogeneity in CAFs is reflected in their ability to secrete a wide range of factors that influence tumor behavior. In this review, we delve into the origins of CAFs and their diverse functions in the CRC TME. We examine the complex interactions between CAFs and tumor cells, including the role of exosomes in mediating these interactions. Furthermore, we discuss the therapeutic implications of targeting CAFs in CRC, highlighting their potential as novel therapeutic targets. Here we specifically propose the mechanisms underlying the role of diet and microbiota in promoting CRC progression through their interactions with CAFs. Understanding the complex roles of CAFs in CRC progression holds the key to developing more effective therapeutic strategies against this deadly disease.
Fibroblasts occupy a fundamental position among the cellular constituents of connective tissue, essential for maintaining the structural scaffold and physiological functions of these tissues. In 1858, the German pathologist Rudolf L.K. Virchow first reported spindle cells in connective tissue. These cells are embedded in the fibrous matrix and are responsible for the synthesis and secretion of extracellular matrix (ECM). In rectal juvenile polyps, Römer captured the morphology of fibroblastic cells, which are spindle-shaped or flat star-shaped with protrusions, considering that these mesenchymal components have great proliferation potential [7]. Fibroblasts in tumor stroma are collectively known as CAFs. Current researchers have identified certain sources of CAF, as depicted in Fig. 1. These originate from the activation of quiescent fibroblasts and stellate cells, the recruitment and differentiation of bone marrow-derived mesenchymal stem cells (MSCs), and furthermore, the transdifferentiation process of epithelial cells, endothelial cells, pericytes, and adipocytes [6,8]. Different opinions have been expressed about the origins of CAFs. The origin of CAF is still controversial since there are no specific markers to them. The consensus among experts suggests that the principal source of CAFs lies in their indigenous precursors, though it is noteworthy that a minor fraction of CAFs traces their origins to alternative ancestral lineages [9]. Among all the origins, fibroblasts are the primary source of CAF in CRC. Within the context of gastrointestinal cancer, native fibroblasts that inhabit the vicinity of tumor cells have been identified as undergoing activation mediated by signaling cues emanating from the tumor cells. This activation process contributes significantly to the emergence of a specific subset of CAFs [10]. Some special sources have been found in CRC. In the colitis-associated cancer (CAC) mouse model, intestinal smooth muscle cells undergo transformation into fibroblasts through the activation of YAP/TAZ, initiating the CAC process, and this transformation process is age-dependent [11]. Furthermore, using lineage-tracing techniques, studies have found that leptin receptor (Lepr)-lineage intestinal stromal cells proliferate and differentiate into melanoma cell adhesion molecule (MCAM)+ CAFs during the development of CRC. These CAFs form a tumor-promoting immune microenvironment by interacting with the nuclear-factor-κB-interleukin 34/C-C Motif Chemokine Ligand 8 (NF-κB-IL34/CCL8) signaling pathway that facilitates the chemotactic migration of macrophages [10].
Figure 1: The diverse origins of CAF and the classification. The sources of CAF include the activation of quiescent fibroblasts and stellate cells, the recruitment and differentiation of bone marrow-derived mesenchymal stem cells as well as the transdifferentiation of epithelial cells, endothelial cells, pericytes, and adipocytes. CAF itself also proliferates, which promotes the aggregation of CAF. These different sources of CAFs can be classified by single-cell sequencing methods. CAF-A is linked to extracellular matrix (ECM) remodeling, expressing MMP2, DCN, and COL1A2. While CAF-B expresses markers that are characteristic of activated myofibroblasts, including ACTA2, TAGLN, and PDGFA. CAF, cancer-associated fibroblast; EMT, epithelial-to-mesenchymal transition; EndMT, endothelial-to-mesenchymal transition; SMC, smooth muscle cell; MMP, matrix metalloproteinase; DCN, decorin; COL1A2, collagen type I alpha 2; ACTA2, actin alpha 2; TAGLN, transgelin; PDGFA, platelet-derived growth factor subunit A.
Different sources of CAF have different effects on tumors because they retain some of the function of the tissue of origin. On the other side, CAFs originating from diverse tissues exhibit a series of unique molecular signatures, which significantly differentiate their molecular expression profiles from those of CAFs of other origins. These distinctive molecular expression patterns are crucial for elucidating their functional roles and underlying mechanisms of CAFs in tumor progression, metastasis, and other pathological processes. In addition, the different molecular expressions of CAFs also suggest their diverse functions. Utilizing single-cell RNA sequencing, Li et al. classified CAFs in CRC into two distinct types: CAF-A and CAF-B. CAF-B expresses markers of activated myofibroblasts, such as actin alpha 2 (ACTA2), transgelin (TAGLN), and platelet-derived growth factor subunit A (PDGFA), affecting TME and cell invasion. CAF-A is linked to ECM remodeling, which expressed metalloproteinase 2 (MMP2), decorin (DCN), and Collagen Type I Alpha 2 (COL1A2), potentially as an intermediate or independent CAF subtype, enabling tumor invasion and metastasis [12]. The functional heterogeneity of CAF is related to the difference in its molecular expression, and its origin is an important factor determining the molecular expression of CAF. Therefore, the functional heterogeneity of CAF may be related to the diversity of its sources.
CAF occupies a pivotal position in many key aspects of tumorigenesis (Fig. 2). The diverse effects of CAFs on cancer may stem from their heterogeneity and plasticity, manifesting in the contextual impact of carcinogenesis. The progression of CRC is a complex and progressive process, stemming from the synergistic effect of multiple carcinogenic factors [13]. It is crucial to obtain more comprehension of its underlying biological mechanisms. In the pathogenetic process of CRC, there exists a complex and multidirectional interactive pattern between CAFs and tumor cells. This relationship is not one-sided but rather a dynamic process involving mutual influences between the two parties. CAFs possess the ability to release a diverse array of bioactive molecules, including growth factors, chemokines, and extracellular matrix proteins, which can directly or indirectly stimulate tumor cell proliferation and survival, thereby promoting the malignant progression of tumors [14]. Furthermore, CAFs possess a remarkable ability to modify the tumor microenvironment. Serving as the “breeding ground” for tumor proliferation and dissemination, those environment exerts a decisive influence on the biological behavior of tumor cells. By virtue of their ability to secrete various enzymes and cytokines, CAFs are able to reshape the structure of the TME, promote the formation of blood vessels and lymphatic vessels, and simultaneously suppress the activity of immune cells, thus creating a more favorable environment for tumor cell growth and dissemination [15].
Figure 2: The function of CAF in driving the progression of CRC resides in its ability to influence the biological activities of CRC cells, blood vessels, immune cells, and the ECM. Specifically, CAFs promote tumor proliferation, angiogenesis, immune suppression, and ECM remodeling, thereby fostering the growth, invasion, and immune evasion of CRC. Furthermore, CAFs are a contributing factor to drug resistance, posing a significant challenge in the clinical management of CRC. This multifaceted role of CAFs underscores their significance in the complex TME. CRC, colorectal cancer cell; CAF, cancer-associated fibroblast; ECM, extracellular matrix; CCL2, C-C motif chemokine ligand 2; CXCL1, C-X-C motif chemokine ligand 1; CTSH, cathepsin H; HGF, hepatocyte growth factor; lnc, long non-coding RNA; miR, microRNA; POSTNi4, periostin isoform 4; TFCP2, transcription factor CP2; TGF-β, transforming growth factor-β; TNF, tumour necrosis factor; VEGF, vascular endothelial growth factor; WNT, Wnt family member.
In recent years, the scientific community has gained a deeper understanding of the role played by fibroblasts in tumor formation and development. Specifically, people are beginning to recognize that the protumorigenic state of fibroblasts can emerge even prior to the malignant alteration of tumor cells [16]. CAFs may form due to damage to the colonic mucosa, oxidative stress, or chronic inflammation [17–19], these changes may precede mutations in epithelial cells. When mutations eventually occur in epithelial cells, these pre-existing CAFs may already be poised to participate in the tumor transformation process, significantly influencing the occurrence and progression of CRC. In the azoxymethane/dextran sodium sulfate (AOM/DSS)-induced colitis-associated cancer model, collagen type VI alpha 1 (Col6a1)+ CAFs can promote tumor-associated angiogenesis and exert oncogenic functions through their innate immune activation by tumour necrosis factor receptor 1 (TNFR1) or IL (interleukin)-1 receptor signaling pathways [20]. Cadinu et al. used multiplexed error-robust fluorescence in situ hybridization (MERFISH) technology to delineate four different subtypes of inflammatory-associated fibroblasts (IAF) in colitis, which are closely related to the spatial organization of the intestinal tract. Among them, IAF1 is associated with early changes in normal lamina propria fibroblasts, while IAF4 located in the top crypt is closely related to extracellular matrix (ECM) remodeling and various immune activation processes [21,22]. These activated fibroblasts in inflammatory bowel disease can promote tumorigenesis [22]. The function of CAF is heterogeneous. Just as the function of CAF in different regions of the same tumor is different, the function of CAF in different development stages of the same tumor is also different. CAFs exhibit stage-specific differential function. For example, in the early stage of CRC (T1CRC), CAFs can upregulate the level of CD44 in tumor cells through direct intercellular communication, thereby promoting matrix remodeling. On the other hand, Cathepsin H is significantly upregulated in CAFs, and directly induces matrix remodeling. While the sustained upregulation of Cathepsin H (CTSH) is specifically observed in T1CRC [23].
CAFs serve as a pivotal perpetrators in the malignant progression. CAFs can secrete related proteins to reshape the extracellular matrix by the balance of metalloproteinases (MMPs) and their inhibitors, alongside other enzymes such as lysyl oxidases (LOX) and play its function by interacting with tumor cells and other stromal cells [24,25]. During the progression of tumorigenesis, CAFs emerge as the key regulators in the unbalanced collagen turnover, ultimately contributing to desmoplasia—a condition characterized by excessive deposition of collagen in the TME [13,24,26]. In CRC, tumors exhibiting a high stromal content have been associated with a dismal prognosis for patients, suggesting a poor clinical outcome [4]. The pattern of desmoplastic reaction (DR) correlates with CRC recurrence [2,27] and liver metastasis [3]. In the context of TME, high expressions of angiogenic factors and inflammatory cytokines, as well as hypoxia, are all contributory factors that significantly enhance tumor angiogenesis [28,29]. In CRC, the absence of p53 significantly triggers the generation of reactive oxygen species (ROS), thereby potently inducing vascular endothelial growth factor (VEGF) production in fibroblasts, ultimately contributing to tumor progression [30]. Kirsten rats arcomaviral oncogene homolog (KRAS) activates the transcription factor CP2 (TFCP2) which upregulates the expression of pro adipogenic factors bone morphogenetic protein 4 (BMP4) and Wnt family member 5B (WNT5B), triggering the transformation of CAFs into lipid-rich CAFs. These lipid-rich CAFs are capable of further producing VEGFR, thereby promoting angiogenesis and tumor progression [31]. KRAS is capable of modulating cellular response to hepatocyte growth factor (HGF) through the HGF-C-MET axis, thereby affecting invasive capabilities [32]. However, one research indicates that despite the crucial role of KRAS mutation in CRC, CRC cells maintain the ability to regulate tumor-promoting characteristics of fibroblasts even in the absence of KRAS expression, suggesting a potential mechanism for resistance to KRAS inhibition [33].
It is noteworthy that CAFs engage in interactions with a majority of cells within TME, encompassing diverse types of immune cells. CAFs can regulate the immune system of the TME by secreting a variety of proteins, cytokines, chemokines, etc., thus promoting the progression of CRC [34–36]. CAFs with different phenotypes have different mechanisms in regulating the immune microenvironment. For example, CAFs expressing myosin light chain 9 (MYL9) exhibit regulatory functions in modulating the release of cytokines and chemokines, including C-X-C motif chemokine ligand 1 (CXCL1), C-C Motif Chemokine Ligand 2 (CCL2), IL-10, and transforming growth factor-β1 (TGF-β1). This modulation triggers the polarization from macrophages M0 to macrophages M2, enhancing the recruitment of macrophages M2 and hindering the activation of dendritic cells [37]. While microfibrillar-associated protein (MFAP5)+ fibroblasts release complement C3, which interacts with myeloid cells, specifically complement C1q C chain (C1QC)+ macrophages, activating the complement system. In turn, myeloid cells can enhance the invasive phenotype of MFAP5+ fibroblasts [38]. In the context of cancer biology, the interaction between CAFs and immune cells generates a positive feedback loop that intensifies, thus fostering the malignant transformation and progression of tumors. Furthermore, research has established that algorithmic models, which capitalize on immune- and CAF-related genes (ICRGs), exhibit remarkable predictive value in forecasting both the prognosis and the efficacy of immunotherapy for patients diagnosed with colorectal cancer [39,40]. Under physiological conditions, the immune system possesses the capability to identify and eliminate abnormal tumor cells. In scenarios where the immune system is impaired or weakened, malignant cells gain the capacity to circumvent the immune system’s surveillance and clearance mechanisms, culminating in tumor genesis. Extensive research has revealed that CAFs play a pivotal role in fostering immunosuppression within the TME and enabling immune evasion in CRC. Specifically, CAFs hinder the infiltration and functional activity of critical immune cells, including CD8+ T-cells, CD4+ T-cells, and natural killer (NK) cells. Furthermore, they can concomitantly promote the activation and recruitment of immunosuppressive cells, exemplified by M2 macrophages, myeloid-derived suppressor cells (MDSCs), and regulatory T-cells (Tregs), thereby augmenting the immune evasion capabilities of tumor cells [41].
One significant pathway tumors employ to achieve immune evasion is through the overexpression of immune checkpoint (IC) molecules. This overexpression attenuates the killing effect of T cells against tumor cells. The essence of immunotherapy targeting immune checkpoints lies in the liberation of T cells, restoring their normal activation level, and enhancing their capability to attack tumor cells [42]. CAF can promote immunotherapy resistance in CRC by mediating T cells. CAFs may suppress CD8+ T cell infiltration through TGFβ signaling, thereby executing immune checkpoint blockade (ICB) [43]. CAFs also play a role in mediating the development of resistance to traditional chemotherapeutic drugs. The production of ECM proteins and matrix remodeling by CAFs, through MMPs, leads to an increase in matrix stiffness, and intertumoral pressure, thereby hindering the penetration of chemotherapeutics and targeted therapies [44]. CAF can also establish intercellular communication with CRC cells via exosomes, leading to tumor drug resistance (as will be further discussed later).
Additionally, one research focusing on young-onset CRC(yCRC) revealed that Actinomyces residing in CAFs promote inflammation and suppress anti-tumor responses by inducing the activation of Toll-like receptor 2/nuclear factor kB (TLR2/NF-kB) and Toll-like receptor 4/nuclear factor kB (TLR4/NF-kB) pathways, while reduces the infiltration of CD8+ T lymphocytes in the TME [45]. This suggests that the progression of CRC can occur via modulation of the gut microbiota existing in CAF, yet these mechanisms require further investigation.
CAF-Derived Exosomes in CRC Progression
In 1981, Trams and his colleagues named the small vesicles with specific membrane structures discovered in the supernatant of cultured sheep red blood cells as “exosomes” [46]. These exosomes are round or elliptical membranous vesicles with an average diameter of 100 nanometers [47]. They are rich in various components such as lipids, proteins or peptides, mRNA, microRNA (miRNA), and long non-coding RNA (lncRNA) [47–49]. Extant investigations have revealed the pivotal significance of exosomes in intercellular communication and disease progression [50]. The exosomes secreted by CAF harbor numerous factors that possess the ability to regulate signaling pathways, pivotal in the advancement of cancer. For example, CAF-derived exosomes (CAFs-exo) miR-20a-5p enhances the proliferation and migration capabilities by targeting phosphatase and tensin homolog (PTEN) to induce the expression of EMT markers, which is closely associated with the occurrence of lymph node metastasis in advanced stages of the tumor [51]. CAFs-exo miR-522-3p promotes the growth and metastasis of CRC by downregulating bone morphogenetic protein 5 (BMP5) [52]. The exosomal miR-625-3p derived from CAFs-xxo may promote the development of chemotherapy resistance in CRC by inhibiting the CUGBP elav-like family member 2/WW domain-containing oxidoreductase (CELF2/WWOX) pathway [53]. CAFs elevate the level of miR-92a-3p in CRC cells via exosomes, thereby activating the Wnt/β-catenin pathway and inhibiting mitochondrial apoptosis by directly suppressing F-box and WD repeat domain-containing 7 (FBXW7) and modulator of apoptosis 1 (MOAP1). This process contributes significantly to the enhancement of cellular stemness, EMT, metastasis, and 5-fluorouracil/oxaliplatin (5-FU/L-OHP) resistance in CRC [54].
In a similar fashion to miRNA, lncRNA secreted by CAF via exosomes has exhibited properties that contribute to tumor progression and promotion. By modulating the miR-34b-5p/CRK Like proto-oncogene (miR-34b-5p/CRKL) axis, LINC00355 derived from CAFs-exo promotes epithelial-to-mesenchymal transition (EMT) and chemotherapy resistance in CRC [55]. CAFs-exo LINC00355 facilitates EMT and resistance to chemotherapy with 5-FU, Oxaliplatin (OXA), and cisplatin via modulation of the miR-34b-5p/CRKL axis [55]. The combination of platinum-based drugs with other chemotherapy drugs (such as 5-FU and leucovorin calcium) is one of the standard basic chemotherapy regimens for colorectal cancer [56]. Studies have found that CAF upregulates the expression of IL11 and periostin isoform 4 (POSTNi4) through direct activation of the TGF-β pathway, leading to the development of resistance to platinum-based drugs in CRC [57]. CAFs-exo also has the ability to induce resistance to platinum-based drugs in CRC, indicating that they may have an emerging role in drug resistance during the treatment of CRC. CAFs-exo lnc-FAL1 promotes the development of oxaliplatin resistance in CRC by facilitating tripartite motif containing 3 (TRIM3)-dependent Beclin1 polyubiquitination and degradation, which consequently inhibits oxaliplatin-induced autophagic cell death [58]. Besides, CAFs-exo miR-93-5p induces the proliferation of SW480 cells, thus resisting the apoptosis induced by radiotherapy [59]. These findings not only reveal the significant role of CAFs-exo in the progression of CRC but also provide new potential targets for the treatment.
During the progression of CRC, CAFs play a pivotal role by virtue of their unique abilities to regulate tumor cell proliferation, invasion, and metastasis, while also influencing cellular immune responses and chemotherapy resistance. Given the complexity of the TME and the multifaceted support provided by CAFs for tumor growth, they emerge as highly promising targets for cancer therapy [60]. Therapeutic strategies targeting CAFs are anticipated to offer novel perspectives and approaches for cancer intervention and treatment. The potential therapeutic approaches based on CAFs have been extensively investigated, and their core concepts can be summarized in two major directions (Fig. 3). Firstly, the therapeutic strategy targeting CAFs themselves primarily focuses on achieving therapeutic goals by eliminating specific CAF subpopulations. FAP+ CAF is currently the most commonly used target [61]. Other targets include platelet-derived growth factor receptor alpha (PDGFRA), and CD142 [62,63]. Secondly, researchers have also concentrated on regulating the interaction between tumor cells and CAFs, aiming to attenuate their synergistic effects on cancer development, particularly targeting the positive feedback mechanisms that promote cancer progression [64]. This interaction involves the activation of various tumor-related pathways mediated by cytokines and growth factors. By intervening in the intercellular communication between tumor cells and CAFs, CAFs can be deactivated, inducing their transition to non-tumor-promoting subtypes, or directly inhibiting the tumor-promoting effects of CAFs on tumor cells [65,66]. Additionally, some other strategy involves modulating immune cells within the ECM or the physical properties of the ECM to influence the function of CAFs, thereby providing new perspectives and methods for treatment [43,44,67,68]. These CAF-based treatments are primarily carried out through pharmacological interventions, and the progress of these drugs and their clinical studies has been comprehensively outlined in previous reviews [16,69].
Figure 3: Potential therapeutic strategies for CRC with CAF. The potential therapeutic approaches based on CAFs can be summarized in two major directions. Firstly, the therapeutic strategy targeting CAFs themselves focuses on eliminating specific CAF subpopulations including FAP+, PDGFRA+, and CD142+. Secondly, researchers have also concentrated on regulating the interaction between tumor cells and CAFs, mediated by cytokines and growth factors. Additionally, some other strategy indicates modulating immune cells within the ECM or the physical properties of the ECM. Furthermore, ketogenic diets and low-fat diets have the potential to extend the survival duration of patients with CRC. Targeting microbiota also presents a potential approach for achieving therapeutic efficacy in CRC. CRC, colorectal cancer cell; CAF, cancer-associated fibroblast; ECM, extracellular matrix; FAP, fibroblast activation protein; PDGFA, platelet-derived growth factor subunit A.
Furthermore, several metabolic-based studies have indicated the potential of ketogenic diets and low-fat diets to extend the survival duration of patients with CRC [70,71]. A ketogenic diet can increase ketone body production in the TME of MC38 CRC mice, thereby inhibiting Krüppel factor 5 (KLF5)-dependent CXCL12 expression in CAFs. By reducing the level of CXCL12, NK cells and CD4+ T cells are enriched in the TME, while immunosuppressive Treg cells and MDSCs are depleted. This reconstruction of the immune tumor microenvironment and reactivation of immune surveillance can enhance the anti-cancer effect of PD-1 blockade in murine CRC [70]. Following the intake of a high-fat diet, the metabolic production of conjugated bile acids (BAs) prompts an increase in the number of bacteria with bile salt hydrolase activity in the colon, thereby disrupting the normal metabolic process of BAs. This alteration results in a significant rise in the levels of deconjugated and secondary BA within the colon. These elevated BA, via the farnesoid X receptor (FXR), excessively activate the WNT signaling pathway in colonic MSC, inducing CAF-like properties of MSC, ultimately exerting tumorigenesis [71]. Additionally, given the association between the occurrence of CRC and the intestinal microbiota residing in CAFs, targeting these microbiotas through therapeutic interventions presents a potential approach for achieving therapeutic efficacy in CRC [45]. In yCRC, a reduced tumor microbial diversity was observed, with Actinomyces serving as a key microbiota that primarily resides in CAFs. Notably, Actinomyces coexists with other tumor-promoting microbiota, including Bacteroidia, Gammaproteobacteria, and Pseudomonas. On the other hand, Actinomyces has been found to facilitate intestinal inflammation and suppress antitumor immunity, thereby contributing to tumor progression. Consequently, microbial therapy emerges as a promising non-invasive therapeutic target.
In the intricate landscape of CRC progression, CAFs have emerged as crucial players in tumor development, metastasis, and resistance to therapeutic interventions. The pleiotropic effects of CAFs, stemming from their heterogeneous origin and high plasticity, are now being recognized as potential targets for novel therapeutic strategies [35,72]. This review has provided a comprehensive overview of the origin of CAFs, their multifaceted roles in the CRC tumor microenvironment (TME), and the interplay between CAFs and exosomes.
The understanding of CAFs’ role in CRC has evolved from mere stromal components to active modulators of tumor growth and metastasis. CAFs not only contribute to the establishment of tumor-permissive extracellular matrix structures and angiogenesis but also modulate the immune status of the TME. These activities facilitate tumor cell invasion, migration, and evasion from immune surveillance, ultimately influencing tumor progression and therapeutic resistance. Exosomes, as intercellular communication vehicles, have been shown to play a significant role in the crosstalk between CAFs and tumor cells. These nano-sized vesicles carry a cargo of proteins, lipids, and nucleic acids, which can be transferred to recipient cells, modulating their behavior. The role of exosomes in CAF-mediated tumor progression and metastasis remains an area of active investigation. Given the specificity and complexity of CAFs in CRC, targeting CAFs represents a promising therapeutic approach [73]. Potential strategies include the inhibition of CAF activation, disruption of CAF-tumor cell crosstalk, and modulation of CAF-derived signaling pathways. However, the translation of these preclinical findings into clinical practice faces several challenges, including the identification of specific CAF markers, the development of target-specific therapeutics, and the assessment of their safety and efficacy in clinical trials [74]. Future research should focus on elucidating the molecular mechanisms underlying CAF heterogeneity and plasticity, as well as their role in tumor progression and therapeutic resistance. This will not only provide insights into the biology of CAFs but also identify potential therapeutic targets. Furthermore, the development of novel CAF-specific biomarkers will enable the selection of appropriate therapeutic strategies.
In conclusion, CAFs represent a promising therapeutic target in CRC. The understanding of their role in tumor progression and the development of CAF-specific therapeutics could significantly impact the management of CRC patients. However, further research is needed to address the challenges faced in translating these findings into clinical practice.
Acknowledgement: The author thanks all members of Deng Lab for their help. Thanks for the academic exchange platform provided by Zhejiang University.
Funding Statement: This study was supported by grants from the National Natural Science Foundation of China (No. 30870971), Major Program of NSFC (No. 81090420), and National Natural Science Foundation of Zhejiang (LY17H160017, LY12H16027).
Author Contributions: The authors confirm their contribution to the paper as follows: study conception and design: Zeyin Lai, Hong Deng; draft manuscript preparation: Zeyin Lai; review and editing: Zeyin Lai, Hangyuan Zhao, Hong Deng; visualization: Zeyin Lai, Hangyuan Zhao; supervision: Hong Deng. All authors reviewed the results and approved the final version of the manuscript.
Availability of Data and Materials: Data sharing is not applicable to this article as no datasets were generated or analyzed during the current study.
Ethics Approval: Not applicable.
Conflicts of Interest: The authors declare that they have no conflicts of interest to report regarding the present study.
References
1. Siegel RL, Giaquinto AN, Jemal A. Cancer statistics, 2024. CA Cancer J Clin. 2024;74(1):12–49. doi:10.3322/caac.v74.1. [Google Scholar] [CrossRef]
2. Ueno H, Kanemitsu Y, Sekine S, Ishiguro M, Ito E, Hashiguchi Y, et al. Desmoplastic pattern at the tumor front defines poor-prognosis subtypes of colorectal cancer. Am J Surg Pathol. 2017;41(11):1506–12. doi:10.1097/PAS.0000000000000946. [Google Scholar] [PubMed] [CrossRef]
3. Ao T, Kajiwara Y, Yonemura K, Shinto E, Mochizuki S, Okamoto K, et al. Prognostic significance of histological categorization of desmoplastic reaction in colorectal liver metastases. Virchows Arch. 2019;475(3):341–8. doi:10.1007/s00428-019-02580-2. [Google Scholar] [PubMed] [CrossRef]
4. van Pelt GW, Sandberg TP, Morreau H, Gelderblom H, van Krieken J, Tollenaar R, et al. The tumour-stroma ratio in colon cancer: the biological role and its prognostic impact. Histopathology. 2018;73(2):197–206. doi:10.1111/his.2018.73.issue-2. [Google Scholar] [CrossRef]
5. Liao Z, Chua D, Tan NS. Reactive oxygen species: a volatile driver of field cancerization and metastasis. Mol Cancer. 2019;18(1):65. doi:10.1186/s12943-019-0961-y. [Google Scholar] [PubMed] [CrossRef]
6. Plikus MV, Wang X, Sinha S, Forte E, Thompson SM, Herzog EL, et al. Fibroblasts: origins, definitions, and functions in health and disease. Cell. 2021;184(15):3852–72. doi:10.1016/j.cell.2021.06.024. [Google Scholar] [PubMed] [CrossRef]
7. Römer H, Cotte C, Essenfeld-Yahr E. Behaviour of the rectal juvenile polyp in vitro. Gut. 1971;12(3):194–6. doi:10.1136/gut.12.3.194. [Google Scholar] [PubMed] [CrossRef]
8. Chen X, Song E. Turning foes to friends: targeting cancer-associated fibroblasts. Nat Rev Drug Discov. 2019;18(2):99–115. doi:10.1038/s41573-018-0004-1. [Google Scholar] [PubMed] [CrossRef]
9. Sahai E, Astsaturov I, Cukierman E, DeNardo DG, Egeblad M, Evans RM, et al. A framework for advancing our understanding of cancer-associated fibroblasts. Nat Rev Cancer. 2020;20(3):174–86. doi:10.1038/s41568-019-0238-1. [Google Scholar] [PubMed] [CrossRef]
10. Kobayashi H, Gieniec KA, Lannagan TRM, Wang T, Asai N, Mizutani Y, et al. The origin and contribution of cancer-associated fibroblasts in colorectal carcinogenesis. Gastroenterology. 2022;162(3):890–906. doi:10.1053/j.gastro.2021.11.037. [Google Scholar] [PubMed] [CrossRef]
11. Liu Y, Ji Y, Jiang R, Fang C, Shi G, Cheng L, et al. Reduced smooth muscle-fibroblasts transformation potentially decreases intestinal wound healing and colitis-associated cancer in ageing mice. Signal Transduct Target Ther. 2023;8(1):294. doi:10.1038/s41392-023-01554-w. [Google Scholar] [PubMed] [CrossRef]
12. Li H, Courtois ET, Sengupta D, Tan Y, Chen KH, Goh JJL, et al. Reference component analysis of single-cell transcriptomes elucidates cellular heterogeneity in human colorectal tumors. Nat Genet. 2017;49(5):708–18. doi:10.1038/ng.3818. [Google Scholar] [PubMed] [CrossRef]
13. Kalluri R. The biology and function of fibroblasts in cancer. Nat Rev Cancer. 2016;16(9):582–98. doi:10.1038/nrc.2016.73. [Google Scholar] [PubMed] [CrossRef]
14. Hexun Z, Miyake T, Maekawa T, Mori H, Yasukawa D, Ohno M, et al. High abundance of Lachnospiraceae in the human gut microbiome is related to high immunoscores in advanced colorectal cancer. Cancer Immunol Immunother. 2023;72(2):315–26. doi:10.1007/s00262-022-03256-8. [Google Scholar] [PubMed] [CrossRef]
15. Nissen NI, Karsdal M, Willumsen N. Collagens and cancer associated fibroblasts in the reactive stroma and its relation to cancer biology. J Exp Clin Cancer Res. 2019;38(1):115. doi:10.1186/s13046-019-1110-6. [Google Scholar] [PubMed] [CrossRef]
16. Abudukelimu S, de Miranda N, Hawinkels L. Fibroblasts in orchestrating colorectal tumorigenesis and progression. Cell Mol Gastroenterol Hepatol. 2024;17(5):821–6. doi:10.1016/j.jcmgh.2024.01.013. [Google Scholar] [PubMed] [CrossRef]
17. Kinchen J, Chen HH, Parikh K, Antanaviciute A, Jagielowicz M, Fawkner-Corbett D, et al. Structural remodeling of the human colonic mesenchyme in inflammatory bowel disease. Cell. 2018;175(2):372–86.e17. doi:10.1016/j.cell.2018.08.067. [Google Scholar] [PubMed] [CrossRef]
18. Henderson NC, Rieder F, Wynn TA. Fibrosis: from mechanisms to medicines. Nature. 2020;587(7835):555–66. doi:10.1038/s41586-020-2938-9. [Google Scholar] [PubMed] [CrossRef]
19. Zhong X, He X, Wang Y, Hu Z, Huang H, Zhao S, et al. Warburg effect in colorectal cancer: the emerging roles in tumor microenvironment and therapeutic implications. J Hematol Oncol. 2022;15(1):160. doi:10.1186/s13045-022-01358-5. [Google Scholar] [PubMed] [CrossRef]
20. Chalkidi N, Melissari MT, Henriques A, Stavropoulou A, Kollias G, Koliaraki V. Activation and functions of Col6a1+ fibroblasts in colitis-associated cancer. Int J Mol Sci. 2023;25(1):148. doi:10.3390/ijms25010148. [Google Scholar] [PubMed] [CrossRef]
21. Cadinu P, Sivanathan KN, Misra A, Xu RJ, Mangani D, Yang E, et al. Charting the cellular biogeography in colitis reveals fibroblast trajectories and coordinated spatial remodeling. Cell. 2024;187(8):2010–28.e30. doi:10.1016/j.cell.2024.03.013. [Google Scholar] [PubMed] [CrossRef]
22. Biffi G, Tuveson DA. Diversity and biology of cancer-associated fibroblasts. Physiol Rev. 2021;101(1):147–76. doi:10.1152/physrev.00048.2019. [Google Scholar] [PubMed] [CrossRef]
23. Dang H, Harryvan TJ, Liao CY, Danen EHJ, Spalburg V, Kielbasa SM, et al. Cancer-associated fibroblasts are key determinants of cancer cell invasion in the earliest stage of colorectal cancer. Cell Mol Gastroenterol Hepatol. 2023;16(1):107–31. doi:10.1016/j.jcmgh.2023.04.004. [Google Scholar] [PubMed] [CrossRef]
24. Pankova D, Chen Y, Terajima M, Schliekelman MJ, Baird BN, Fahrenholtz M, et al. Cancer-associated fibroblasts induce a collagen cross-link switch in tumor stroma. Mol Cancer Res. 2016;14(3):287–95. doi:10.1158/1541-7786.MCR-15-0307. [Google Scholar] [PubMed] [CrossRef]
25. Mochizuki S, Ao T, Sugiura T, Yonemura K, Shiraishi T, Kajiwara Y, et al. Expression and function of a disintegrin and metalloproteinases in cancer-associated fibroblasts of colorectal cancer. Digestion. 2020;101(1):18–24. doi:10.1159/000504087. [Google Scholar] [PubMed] [CrossRef]
26. Bhattacharjee S, Hamberger F, Ravichandra A, Miller M, Nair A, Affo S, et al. Tumor restriction by type I collagen opposes tumor-promoting effects of cancer-associated fibroblasts. J Clin Invest. 2021;131(11):e146987. doi:10.1172/JCI146987. [Google Scholar] [PubMed] [CrossRef]
27. Ueno H, Kanemitsu Y, Sekine S, Ishiguro M, Ito E, Hashiguchi Y, et al. A multicenter study of the prognostic value of desmoplastic reaction categorization in stage II colorectal cancer. Am J Surg Pathol. 2019;43(8):1015–22. doi:10.1097/PAS.0000000000001272. [Google Scholar] [PubMed] [CrossRef]
28. Shaw P, Dwivedi SKD, Bhattacharya R, Mukherjee P, Rao G. VEGF signaling: role in angiogenesis and beyond. Biochim Biophys Acta Rev Cancer. 2024;1879(2):189079. doi:10.1016/j.bbcan.2024.189079. [Google Scholar] [PubMed] [CrossRef]
29. Lugano R, Ramachandran M, Dimberg A. Tumor angiogenesis: causes, consequences, challenges and opportunities. Cell Mol Life Sci. 2020;77(9):1745–70. doi:10.1007/s00018-019-03351-7. [Google Scholar] [PubMed] [CrossRef]
30. Hayashi Y, Tsujii M, Kodama T, Akasaka T, Kondo J, Hikita H, et al. p53 functional deficiency in human colon cancer cells promotes fibroblast-mediated angiogenesis and tumor growth. Carcinogenesis. 2016;37(10):972–84. doi:10.1093/carcin/bgw085. [Google Scholar] [PubMed] [CrossRef]
31. Hsu WH, LaBella KA, Lin Y, Xu P, Lee R, Hsieh CE, et al. Oncogenic KRAS drives lipofibrogenesis to promote angiogenesis and colon cancer progression. Cancer Discov. 2023;13(12):2652–73. doi:10.1158/2159-8290.CD-22-1467. [Google Scholar] [PubMed] [CrossRef]
32. Dias Carvalho P, Martins F, Mendonça S, Ribeiro A, Machado AL, Carvalho J, et al. Mutant KRAS modulates colorectal cancer cells invasive response to fibroblast-secreted factors through the HGF/C-MET axis. Int J Cancer. 2022;151(10):1810–23. doi:10.1002/ijc.v151.10. [Google Scholar] [CrossRef]
33. Dias Carvalho P, Mendonça S, Martins F, Oliveira MJ, Velho S. Modulation of fibroblast phenotype by colorectal cancer cell-secreted factors is mostly independent of oncogenic KRAS. Cells. 2022;11(16):2490. doi:10.3390/cells11162490. [Google Scholar] [PubMed] [CrossRef]
34. Papait A, Romoli J, Stefani FR, Chiodelli P, Montresor MC, Agoni L, et al. Fight the cancer, hit the CAF! Cancers. 2022;14(15):3570. doi:10.3390/cancers14153570. [Google Scholar] [PubMed] [CrossRef]
35. Mao X, Xu J, Wang W, Liang C, Hua J, Liu J, et al. Crosstalk between cancer-associated fibroblasts and immune cells in the tumor microenvironment: new findings and future perspectives. Mol Cancer. 2021;20(1):131. doi:10.1186/s12943-021-01428-1. [Google Scholar] [PubMed] [CrossRef]
36. Arpinati L, Scherz-Shouval R. From gatekeepers to providers: regulation of immune functions by cancer-associated fibroblasts. Trends Cancer. 2023;9(5):421–43. doi:10.1016/j.trecan.2023.01.007. [Google Scholar] [PubMed] [CrossRef]
37. Deng S, Cheng D, Wang J, Gu J, Xue Y, Jiang Z, et al. MYL9 expressed in cancer-associated fibroblasts regulate the immune microenvironment of colorectal cancer and promotes tumor progression in an autocrine manner. J Exp Clin Cancer Res. 2023;42(1):294. doi:10.1186/s13046-023-02863-2. [Google Scholar] [PubMed] [CrossRef]
38. Peng Z, Ren Z, Tong Z, Zhu Y, Zhu Y, Hu K. Interactions between MFAP5 + fibroblasts and tumor-infiltrating myeloid cells shape the malignant microenvironment of colorectal cancer. J Transl Med. 2023;21(1):405. doi:10.1186/s12967-023-04281-6. [Google Scholar] [PubMed] [CrossRef]
39. Wei J, Ge X, Qian Y, Jiang K, Chen X, Lu W, et al. Development and verification of a combined immune- and cancer-associated fibroblast related prognostic signature for colon adenocarcinoma. Front Immunol. 2024;15:1291938. doi:10.3389/fimmu.2024.1291938. [Google Scholar] [PubMed] [CrossRef]
40. Zheng L, Zhang J, Ye Y, Shi Z, Huang Y, Zhang M, et al. Construction of a novel cancer-associated fibroblast-related signature to predict clinical outcome and immune response in colon adenocarcinoma. Aging. 2023;15(18):9521–43. doi:10.18632/aging.v15i18. [Google Scholar] [CrossRef]
41. Chen Y, Liang Z, Lai M. Targeting the devil: strategies against cancer-associated fibroblasts in colorectal cancer. Transl Res. 2024;270:81–93. doi:10.1016/j.trsl.2024.04.003. [Google Scholar] [PubMed] [CrossRef]
42. Picard E, Verschoor CP, Ma GW, Pawelec G. Relationships between immune landscapes, genetic subtypes and responses to immunotherapy in colorectal cancer. Front Immunol. 2020;11:369. doi:10.3389/fimmu.2020.00369. [Google Scholar] [PubMed] [CrossRef]
43. Zhang D, Ni QQ, Liang QY, He LL, Qiu BW, Zhang LJ, et al. ASCL2 induces an immune excluded microenvironment by activating cancer-associated fibroblasts in microsatellite stable colorectal cancer. Oncogene. 2023;42(38):2841–53. doi:10.1038/s41388-023-02806-3. [Google Scholar] [PubMed] [CrossRef]
44. Provenzano PP, Cuevas C, Chang AE, Goel VK, Von Hoff DD, Hingorani SR. Enzymatic targeting of the stroma ablates physical barriers to treatment of pancreatic ductal adenocarcinoma. Cancer Cell. 2012;21(3):418–29. doi:10.1016/j.ccr.2012.01.007. [Google Scholar] [PubMed] [CrossRef]
45. Xu Z, Lv Z, Chen F, Zhang Y, Xu Z, Huo J, et al. Dysbiosis of human tumor microbiome and aberrant residence of Actinomyces in tumor-associated fibroblasts in young-onset colorectal cancer. Front Immunol. 2022;13:1008975. doi:10.3389/fimmu.2022.1008975. [Google Scholar] [PubMed] [CrossRef]
46. Trams EG, Lauter CJ, Salem Jr.N, Heine U. Exfoliation of membrane ecto-enzymes in the form of micro-vesicles. Biochim Biophys Acta. 1981;645(1):63–70. doi:10.1016/0005-2736(81)90512-5. [Google Scholar] [PubMed] [CrossRef]
47. Kalluri R, LeBleu VS. The biology, function, and biomedical applications of exosomes. Science. 2020;367(6478):3700. [Google Scholar]
48. Sun Z, Yang S, Zhou Q, Wang G, Song J, Li Z, et al. Emerging role of exosome-derived long non-coding RNAs in tumor microenvironment. Mol Cancer. 2018;17(1):82. doi:10.1186/s12943-018-0831-z. [Google Scholar] [PubMed] [CrossRef]
49. Li C, Zhou T, Chen J, Li R, Chen H, Luo S, et al. The role of exosomal miRNAs in cancer. J Transl Med. 2022;20(1):6. doi:10.1186/s12967-021-03215-4. [Google Scholar] [PubMed] [CrossRef]
50. Zhang L, Yu D. Exosomes in cancer development, metastasis, and immunity. Biochim Biophys Acta Rev Cancer. 2019;1871(2):455–68. doi:10.1016/j.bbcan.2019.04.004. [Google Scholar] [PubMed] [CrossRef]
51. Ghofrani-Shahpar M, Pakravan K, Razmara E, Amooie F, Mahmoudian M, Heshmati M, et al. Cancer-associated fibroblasts drive colorectal cancer cell progression through exosomal miR-20a-5p-mediated targeting of PTEN and stimulating interleukin-6 production. BMC Cancer. 2024;24(1):400. doi:10.1186/s12885-024-12190-0. [Google Scholar] [PubMed] [CrossRef]
52. Zhang J, Pan Y, Jin L, Yang H, Cao P. Exosomal-miR-522-3p derived from cancer-associated fibroblasts accelerates tumor metastasis and angiogenesis via repression bone morphogenetic protein 5 in colorectal cancer. J Gastroenterol Hepatol. 2024;39(1):107–20. doi:10.1111/jgh.v39.1. [Google Scholar] [CrossRef]
53. Zhang Y, Yin C, Wei C, Xia S, Qiao Z, Zhang XW, et al. Exosomal miR-625-3p secreted by cancer-associated fibroblasts in colorectal cancer promotes EMT and chemotherapeutic resistance by blocking the CELF2/WWOX pathway. Pharmacol Res. 2022;186:106534. doi:10.1016/j.phrs.2022.106534. [Google Scholar] [PubMed] [CrossRef]
54. Hu JL, Wang W, Lan XL, Zeng ZC, Liang YS, Yan YR, et al. CAFs secreted exosomes promote metastasis and chemotherapy resistance by enhancing cell stemness and epithelial-mesenchymal transition in colorectal cancer. Mol Cancer. 2019;18(1):91. doi:10.1186/s12943-019-1019-x. [Google Scholar] [PubMed] [CrossRef]
55. Hu JH, Tang HN, Wang YH. Cancer-associated fibroblast exosome LINC00355 promotes epithelial-mesenchymal transition and chemoresistance in colorectal cancer through the miR-34b-5p/CRKL axis. Cancer Gene Ther. 2024;31(2):259–72. doi:10.1038/s41417-023-00700-4. [Google Scholar] [PubMed] [CrossRef]
56. Yang L, Yang J, Kleppe A, Danielsen HE, Kerr DJ. Personalizing adjuvant therapy for patients with colorectal cancer. Nat Rev Clin Oncol. 2024;21(1):67–79. doi:10.1038/s41571-023-00834-2. [Google Scholar] [PubMed] [CrossRef]
57. Linares J, Sallent-Aragay A, Badia-Ramentol J, Recort-Bascuas A, Méndez A, Manero-Rupérez N, et al. Long-term platinum-based drug accumulation in cancer-associated fibroblasts promotes colorectal cancer progression and resistance to therapy. Nat Commun. 2023;14(1):746. doi:10.1038/s41467-023-36334-1. [Google Scholar] [PubMed] [CrossRef]
58. Zhu S, Mao J, Zhang X, Wang P, Zhou Y, Tong J, et al. CAF-derived exosomal lncRNA FAL1 promotes chemoresistance to oxaliplatin by regulating autophagy in colorectal cancer. Dig Liver Dis. 2024;56(2):330–42. doi:10.1016/j.dld.2023.06.010. [Google Scholar] [PubMed] [CrossRef]
59. Chen X, Liu J, Zhang Q, Liu B, Cheng Y, Zhang Y, et al. Exosome-mediated transfer of miR-93-5p from cancer-associated fibroblasts confer radioresistance in colorectal cancer cells by downregulating FOXA1 and upregulating TGFB3. J Exp Clin Cancer Res. 2020;39(1):65. doi:10.1186/s13046-019-1507-2. [Google Scholar] [PubMed] [CrossRef]
60. Wu C, Gu J, Gu H, Zhang X, Zhang X, Ji R. The recent advances of cancer associated fibroblasts in cancer progression and therapy. Front Oncol. 2022;12:1008843. doi:10.3389/fonc.2022.1008843. [Google Scholar] [PubMed] [CrossRef]
61. Duperret EK, Trautz A, Ammons D, Perales-Puchalt A, Wise MC, Yan J, et al. Alteration of the tumor stroma using a consensus dna vaccine targeting fibroblast activation protein (FAP) synergizes with antitumor vaccine therapy in mice. Clin Cancer Res. 2018;24(5):1190–201. doi:10.1158/1078-0432.CCR-17-2033. [Google Scholar] [PubMed] [CrossRef]
62. Peters NA, Constantinides A, Ubink I, van Kuik J, Bloemendal HJ, van Dodewaard JM, et al. Consensus molecular subtype 4 (CMS4)-targeted therapy in primary colon cancer: a proof-of-concept study. Front Oncol. 2022;12:969855. doi:10.3389/fonc.2022.969855. [Google Scholar] [PubMed] [CrossRef]
63. Soós A, Kelemen A, Orosz A, Szvicsek Z, Tölgyes T, Dede K, et al. High CD142 level marks tumor-promoting fibroblasts with targeting potential in colorectal cancer. Int J Mol Sci. 2023;24(14):11585. doi:10.3390/ijms241411585. [Google Scholar] [PubMed] [CrossRef]
64. Ji Q, Zhou L, Sui H, Yang L, Wu X, Song Q, et al. Primary tumors release ITGBL1-rich extracellular vesicles to promote distal metastatic tumor growth through fibroblast-niche formation. Nat Commun. 2020;11(1):1211. doi:10.1038/s41467-020-14869-x. [Google Scholar] [PubMed] [CrossRef]
65. Greenburg G, Hay ED. Epithelia suspended in collagen gels can lose polarity and express characteristics of migrating mesenchymal cells. J Cell Biol. 1982;95(1):333–9. doi:10.1083/jcb.95.1.333. [Google Scholar] [PubMed] [CrossRef]
66. Yan L, Zheng J, Wang Q, Hao H. Role of cancer-associated fibroblasts in colorectal cancer and their potential as therapeutic targets. Biochem Biophys Res Commun. 2023;681:127–35. doi:10.1016/j.bbrc.2023.09.065. [Google Scholar] [PubMed] [CrossRef]
67. Naktubtim C, Payuhakrit W, Uttarawichien T, Hassametto A, Suwannalert P. YAP, a novel target regulates F-actin rearrangement-associated CAFs transformation and promotes colorectal cancer cell progression. Biomed Pharmacother. 2022;155:113757. doi:10.1016/j.biopha.2022.113757. [Google Scholar] [PubMed] [CrossRef]
68. Barbazan J, Pérez-González C, Gómez-González M, Dedenon M, Richon S, Latorre E, et al. Cancer-associated fibroblasts actively compress cancer cells and modulate mechanotransduction. Nat Commun. 2023;14(1):6966. doi:10.1038/s41467-023-42382-4. [Google Scholar] [PubMed] [CrossRef]
69. Raudenska M, Balvan J, Hanelova K, Bugajova M, Masarik M. Cancer-associated fibroblasts: mediators of head and neck tumor microenvironment remodeling. Biochim Biophys Acta Rev Cancer. 2023;1878(5):188940. doi:10.1016/j.bbcan.2023.188940. [Google Scholar] [PubMed] [CrossRef]
70. Wei R, Zhou Y, Li C, Rychahou P, Zhang S, Titlow WB, et al. Ketogenesis attenuates KLF5-dependent production of CXCL12 to overcome the immunosuppressive tumor microenvironment in colorectal cancer. Cancer Res. 2022;82(8):1575–88. doi:10.1158/0008-5472.CAN-21-2778. [Google Scholar] [PubMed] [CrossRef]
71. Kim TY, Kim S, Kim Y, Lee YS, Lee S, Lee SH, et al. A high-fat diet activates the BAs-FXR axis and triggers cancer-associated fibroblast properties in the colon. Cell Mol Gastroenterol Hepatol. 2022;13(4):1141–59. doi:10.1016/j.jcmgh.2021.12.015. [Google Scholar] [PubMed] [CrossRef]
72. Chhabra Y, Weeraratna AT. Fibroblasts in cancer: unity in heterogeneity. Cell. 2023;186(8):1580–609. doi:10.1016/j.cell.2023.03.016. [Google Scholar] [PubMed] [CrossRef]
73. Kanzaki R, Pietras K. Heterogeneity of cancer-associated fibroblasts: opportunities for precision medicine. Cancer Sci. 2020;111(8):2708–17. doi:10.1111/cas.v111.8. [Google Scholar] [CrossRef]
74. Henrich LM, Greimelmaier K, Wessolly M, Klopp NA, Mairinger E, Krause Y, et al. The impact of cancer-associated fibroblasts on the biology and progression of colorectal carcinomas. Genes. 2024;15(2):209. doi:10.3390/genes15020209. [Google Scholar] [PubMed] [CrossRef]
Cite This Article
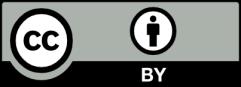
This work is licensed under a Creative Commons Attribution 4.0 International License , which permits unrestricted use, distribution, and reproduction in any medium, provided the original work is properly cited.