Open Access
REVIEW
Neural stem cell-derived exosomes: a cell-free transplant for potential cure of neurological diseases
1 Department of Neurosurgery, Southern Medical University Hospital of Integrated Traditional Chinese and Western Medicine, Southern Medical University, Guangzhou, 510310, China
2 Department of Orthopaedics, Chaozhou Hospital of Traditional Chinese Medicine, Chaozhou, 521011, China
3 Department of Neurosurgery, The Second People’s Hospital of Pingnan, Pingnan, 537300, China
4 Department of Neurology, School of Medicine, Southeast University, Nanjing, 210009, China
5 Section of Neurosurgery, The Aga Khan University, Karachi, 74800, Pakistan
6 Department of Orthopaedics and Traumatology, The University of Hong Kong, Hong Kong, 999077, China
* Corresponding Author: LUKUI CHEN. Email:
# These authors contributed equally to this work and shared first authorship
(This article belongs to the Special Issue: Exosomes in Cell Communication and Disease: From Biomarkers to Therapeutic Targets)
BIOCELL 2024, 48(10), 1405-1418. https://doi.org/10.32604/biocell.2024.053148
Received 25 April 2024; Accepted 08 July 2024; Issue published 02 October 2024
Abstract
Degeneration and death of nerve cells are inevitable with the occurrence and progression of nervous system disorders. Researchers transplanted neural stem cells into relevant areas, trying to solve the difficulty of neural cell loss by differentiating neural stem cells into various nerve cells. In recent years, however, studies have shown that transplanted neural stem cells help neural tissues regenerate and return to normal through paracrine action rather than just replacing cells. Exosomes are essential paracrine mediators, which can participate in cell communication through substance transmission. In this regard, this review mainly discusses the current research progress of neural stem cell-derived exosomes. This paper mainly introduces the self-regulation of neural stem cells (NSCs)-derived exosomes on neural stem cells to clarify the role of NSCs-derived exosomes in the nervous system; the therapeutic effect of NSCs-derived exosomes on various neurological diseases; introduces different studies of NSCs-derived exosomes, showing more in-depth research results of NSCs-derived exosomes. It presents some ideas to provide a reference for subsequent research on neural stem cell-derived exosomes.Keywords
Abbreviations
ABCB1 | ATP-binding cassette transporter B1 |
AD | Alzheimer’s disease |
APP | Amyloid precursor protein |
BBB | Blood brain barrier |
BDNF | Brain-derived neurotrophic factor |
BDNF | Brain-derived neurotrophic factor |
BMEC | Brain microvascular endothelial cells |
CNS | Central nervous system |
CREB | cAMP response element-binding protein |
DG | Dentate gyrus |
ER | Endoplasmic reticulum |
ERK | Extracellular signal-regulated kinase |
ESCs | Embryonic stem cells |
ESCs | Embryonic stem cells |
FTO | Obesity-associated gene |
HAD | Hyaluronic acid |
HDF | Human dermal fibroblasts |
Hes-1 | Hairy and enhancer of split 1 protein |
hNSC | Human neural stem cell |
HUVECs | Human umbilical vein endothelial cells |
IFN-γ | Interferon-γ |
iNPC | Induced neural stem/progenitor cell |
iPSCs | Induced pluripotent stem cells |
JAK/STAT | Janus kinase/Signal Transducer and Activator of Transcription |
MAPK | Mitogen-activated protein kinase |
MMP-9 | Matrix metalloproteinase 9 |
MPTP | Mitochondrial permeability transition pore |
MVE | Multivesicular endosome |
NDNF | Neuro-derived neurotrophic factor |
NF-κB | Nuclear factor-κB |
NRF1 | Nuclear respiratory factor 1 |
NRF2 | Nuclear factor erythroid 2-related factor 2 |
NSCs | Neural stem cells |
OGD | Oxygen-glucose deprivation |
PD | Parkinson’s disease |
PGC1α | Peroxisome proliferator-activated receptor-γ coactivator-1α |
RGD | Arginine-glycine-aspartic acid |
ROS | Reactive oxygen species |
SCI | Spinal cord injury |
SCMECs | Spinal cord microvascular endothelial cells, |
SGZ | Subgranular zone |
SIRT1 | Sirtuin 1 |
SVZ | Subventricular zone |
TBI | Traumatic brain injury |
VEGF-A | Vascular Endothelial Growth Factor A |
Neural stem cells (NSCs) are a kind of cell with self-renewal ability and multiple differentiation potential in the central nervous system (CNS) [1,2]. Such cells can produce transit-amplifying cells or intermediate progenitors through asymmetric cell division [3,4] or two identical daughter cells through symmetrical division [5]. NSCs can differentiate into neurons, astrocytes, and oligodendrocytes. Therefore, neural stem cells play an essential role in the growth and development of the CNS. When the development of the nervous system is complete (neurons, astrocytes, and oligodendrocytes reach the required number), NSC in most areas of the brain stops proliferation, enters terminal differentiation, or acquires a resting state. There are still active neural stem cells in a small number of areas, known as neurogenic regions, including the subgranular zone (SGZ) of the dentate gyrus (DG) in the hippocampus and the subventricular zone (SVZ) in the forebrain [6–8].
Studies have shown that embryonic stem cells (ESCs) and induced pluripotent stem cells (iPSCs), both primitive pluripotent stem cells, can also change into NSCs. NSCs can proliferate and differentiate into nerve-related cells. Many experimental results have demonstrated that transplanting NSCs into the central nervous systems of individuals who are suffering from nervous system illnesses has a therapeutic impact [9–11]. This stem cell-based therapy works primarily through cell replacement or paracrine effects to stop cells from dying and help host cells get the necessary nutrients. However, in further studies, the researchers found that the survival rate of transplanted stem cells was not ideal (most of the cells survived less than four weeks after transplantation) [12,13]. Other studies have shown that a lot of transplanted cells die off [14–16]. Because of this, it is thought that NSCs protect neurons more through nutrient support than through growth [17–19]. Data from radiation and Alzheimer’s disease (AD) studies show that neural stem cells play a neuroprotective and nutritional support mechanism through exosomes to reduce inflammation and maintain the integrity of damaged brain structures. In the case of skull irradiation, several studies backed up this claim. First, non-thymic nude mice exposed to radiation [17,19] and immunoreactive mice [18] were used to prove that Exo therapy worked. Other studies have also shown that Exo transplantation derived from mouse stem cells can provide neurocognitive benefits in a transgenic mouse model of AD [20,21]. Rong et al. also showed that neural stem cell-derived exosomes improved the neuroinflammation and immune microenvironment of spinal cord injury rats to some extent and reduced neuronal apoptosis [22].
Extracellular vesicles include apoptotic bodies, microvesicles, and exosomes [23]. Exosomes are small extracellular vesicles that range in size from 30 to 200 nm and can be released by many different types of cells [24,25]. It includes proteins, lipids, and nucleic acids [26,27], and can participate in cell-to-cell communication through binding to receptor cells. Exosomes are, therefore, essential regulators in physiological and pathological processes, as well as in intercellular signal transduction and target cell function maintenance. In COVID-19, exosomes have also been shown to play a role [28]. Compared with stem cells, the exosomes derived from stem cells have low immunogenicity [29], high biological barrier penetration [30], high circulatory stability (strong stability in blood circulation) [31], low biotoxicity [32], high biocompatibility [33] and no tumorigenicity [34–36]. Low immunogenicity is of particular concern because immune rejection is one of the main problems in nervous system diseases. Immunosuppressants may cause neurotoxicity and speed up the disease’s progression [37,38] (Fig. 1).
Figure 1: Compared with stem cells, the exosomes derived from stem cells have low immunogenicity, high biological barrier penetration, high circulatory stability (strong stability in blood circulation), low biotoxicity, high biocompatibility, and no tumorigenicity (By Figdraw). Abbreviations: BBB, Blood brain barrier.
Numerous studies have confirmed the possible function of exosomes produced from neural stem cells during the past few years. This article will provide a summary and discussion of the research findings, as well as some suggestions for investigating exosomes produced by neural stem cells.
Self-Regulation of Neural Stem Cells by Neural Stem Cell-Derived Exosomes
The division of neural stem cells can be divided into symmetrical and asymmetrical divisions, and the daughter cells produced by these two division methods are very different. However, how to balance the two ways of division in the body and precisely control the two ways of division has not been thoroughly researched. The researchers studied the effects of exosomes derived from neural stem cells on cell proliferation, differentiation, and cell survival (Fig. 2).
Figure 2: Exosomes can promote the self-proliferation of neural stem cells and their differentiation into various types of neural cells, delay cellular senescence and inhibit apoptosis (By Figdraw). Abbreviations: NSC, Neural stem cell; BDNF, Brain-derived neurotrophic factor; ERK, Extracellular signal-regulated kinase; Hes-1, Hairy and enhancer of split 1 protein.
Exosomes promote self-proliferation
Due to the limited number of endogenous neural stem cells in adults, some researchers propose transplanting exogenous neural stem cells as a promising strategy for treating neurodegenerative diseases [39,40] and traumatic brain injury [41,42]. Transplanted neural stem cells were initially shown to replace damaged neurons and glial cells. On the other hand, recent studies have shown that transplanted neural stem cells may have a therapeutic effect by changing the microenvironment where endogenous neural stem cells are located, which affects endogenous stem cells [43–45]. Ma et al. found that exosomes are key components of neural stem cells affecting the internal environment, which provides the possibility for the therapeutic effect of neural stem cell transplantation. These cells can secrete growth factor-rich exosomes and alter their surrounding environment in ways that promote neural stem cell proliferation [46]. Exosomes promote the proliferation of NSCs and increase the number of endogenous NSCs, providing the basis for the treatment of neurological diseases.
Exosomes promote self-differentiation
Under physiological conditions, neural stem cells differentiate into neurons and glial cells. The researchers found that the differentiation of such cells was not random. Whenever there are changes in the microenvironment, neural stem cells will change the amount of cell differentiation. In the process of neural development, neural stem cells with the characteristics of radial glial cells produce neurons and glial cells in a Spatio-temporal manner. This complex process is driven by external and internal signals, which ultimately determine the type and number of adult nerve cells. Stronati et al. found that exosomes derived from differentiated neural stem cells can guide neural stem cells to differentiate into glial lineages in a dose-dependent way. They still lack a mechanism for further research, and the experiment was only conducted in vitro, which failed to demonstrate that this phenomenon occurred in vivo [47]. Yuan et al. found rich expression of miRNA (miR-9) in the exosomes secreted by neural stem cells and found that miR-9 promotes the differentiation of neural stem cells and the maturation of neurons and glia. At the same time, the team also found that Hes-1 (Hairy and enhancer of split 1 protein) may be the target of the exosomes, and the loss of Hes1 function can promote NSC differentiation [48]. Even though the above experiments have some flaws, they give us an idea of how to make exosome-based acellular therapy strategies.
Exosomes promote cell survival
In the progress of the disease, cell injury and apoptosis are inevitable, and the decrease of normal cells inevitably leads to the aggravation of the condition and corresponding functional damage. Therefore, promoting cell survival is a critical step in delaying the progress of disease or treatment. Promoting cell survival is not only a way to protect the cells in the diseased area, but it is also a way to ensure that transplanted cells will live. The researchers found that exosomes derived from neural stem cells can promote cell survival [49]. In vitro experiments, Ma et al. found that exosomes derived from neural stem cells promote the survival of neural stem cells by regulating the kinase pathway. In the experiment, the team confirmed that exosomes derived from neural stem cells have anti-apoptotic effects. The extracellular signal-regulated kinase (ERK) signaling pathway is one of the most important intracellular pathways in the regulation of cell death. The activation of the ERK pathway significantly increases the viability of neural progenitor cells in stroke. However, this pathway may play a dual role in controlling the apoptosis of other types of brain cells, such as neurons. Therefore, the role of the ERK pathway in apoptosis is controversial, which indicates that the anti-apoptotic effect of Exo derived from induced neural stem/progenitor cell (iNPC) may not apply to all brain cells [46].
In addition to protecting the survival of neural stem cells, researchers are also trying to use exosomes to protect the survival of other cells. Katsur et al. found that exosomes derived from neural stem cells may delay the myocardial mitochondrial permeability transition pore (MPTP) opening through the gp130 signal pathway and downstream janus kinase/signal transducer and activator of transcription (JAK/STAT) pathway. The opening of MPTP is the cause of cardiomyocyte death after reperfusion [50]. Exosomes promote cell survival, reduce cell death in the lesion area, and delay disease progression.
Aging is an inevitable part of how cells stay alive, and the nervous system is a vital part of that process [51,52]. Scientists try to delay the arrival of cell senescence under inevitable aging. Some studies believe that the hypothalamus plays a significant role in the occurrence of senescence [53,54], but the mechanism is still unclear. Natale et al. found that the exosomes of neural stem cells can balance the recruitment of forkhead box O (FoxO) in related gene promoters and resist insulin resistance-induced neurogenic aging [55]. Zhang et al. have found that the hypothalamus plays this procedural role. In response to this finding, the team conducted further research. The pace of aging was accelerated after neural stem cell ablation in the hypothalamus. When NSCs were injected into the hypothalamic region, aging was delayed, but no significant effect was found in the group injected with astrocytes and mesenchymal stem cells. The team then found that the anti-aging effect of NSCs is due to the endocrine function of cells rather than neurogenesis. NSCs-derived exosomes also play an important role in controlling aging [56].
Application of Exosomes Derived from Neural Stem Cells
Stroke has become a major health risk and a massive problem for the world’s healthcare system. A systematic analysis of the 2019 global burden of disease study shows that from 1990 to 2019, the absolute number of strokes worldwide increased by 70.0%, and the number of stroke deaths increased by 43.0% [57]. Ischemic strokes accounted for 62.4% of all strokes in 2019. It has always been the most significant proportion of all stroke events. Tissue plasminogen activator is the only effective drug for treating stroke and must be given within 4.5 h after stroke. Tissue-type plasminogen activator therapy, however, can only help a small number of people [58,59], because there is a short time window for treatment [60]. The therapeutic effect of drugs is significantly weakened for the treatment of peripheral blood administration due to the existence of the blood-brain barrier. Exosomes, on the other hand, can cross the blood-brain barrier and reach the target area.
Researchers found that exosomes derived from neural stem cells are a great way to treat ischemic stroke [61–63]. In the stroke process, the cells around the ischemic focus will die due to ischemia, hypoxia, inflammation, and other factors, and the blood-brain barrier will be damaged to a certain extent. At this time, the protection of surrounding cells is essential. After adding neural stem cell-derived exosomes to the model of neuronal apoptosis induced by CoCl2, Li et al. found that the vitality of neurons increased and the apoptosis rate decreased under hypoxia [64]. The researchers found that NSCs-derived exosomes promoted the proliferation and migration of brain microvascular endothelial cells (BMEC) through miR-9 and reduced cell death under hypoxia. They also found that NSCs-derived exosomes improved cerebrovascular alterations [65]. Injecting neural stem cell-derived exosomes into rats resulted in a smaller lesion area and enhanced spontaneous movement. Further investigations demonstrated that the neuronal survival rate in the ischemic boundary zone was boosted after the injection of neural stem cell exosomes [66]. Through cell and animal experiments, Sun et al. found that neural stem cell exosomes had a protective effect on astrocytes after the oxygen-glucose deprivation (OGD) model. In addition, exosomes produced by neural stem cells were also discovered to greatly diminish the size of cerebral infarction in animal studies [67]. Furthermore, to protect cells, controlling the progression of inflammation is also an essential treatment after stroke. Tian et al. inhibited the progression of inflammation after ischemia by tail vein injection of neural stem cell-derived exosomes into the stroke area. On this basis, the researchers also found that adding neural stem cells to other substances (arginine-glycine-aspartic acid (RGD)-4C peptide) can improve the therapeutic effect of the exosomes in the treatment of ischemic stroke [68]. The researchers additionally discovered that exosomes derived from NSCs promote the expression of nuclear factor erythroid 2-related factor 2 (NRF2) and the M2 polarization of microglia via obesity-associated gene (FTO) transfer, resulting in neuroprotective effects [69]. Zhang et al. introduced the pro-inflammatory factor interferon-γ (IFN-γ) into neural stem cell exosomes to generate IFN-γ-hNSC-Exo. Without affecting the secretion of exosomes, this exosome played a better therapeutic role in both OGD model cells and stroke model rats [70]. In the same way, Zhu et al. used exosomes made from NSCs that carried brain-derived neurotrophic factor (BDNF-hNSC-Exo) to improve cell survival in models of oxidative stress significantly. In rats with stroke, BDNF-hNSC-Exo stopped microglia from becoming active and helped endogenous neural stem cells turn into neurons [71]. Given that exosomes derived from neural stem cells (NSC-derived Exos) are rapidly metabolized and cleared from the body, Gu et al. employed biomaterials as a delivery system to encapsulate these exosomes within a hyaluronic acid (HAD) hydrogel. This hydrogel was then injected directly into the lesion site, facilitating a sustained release of the Exos. The study demonstrated that the HAD hydrogel could reliably and continuously release the Exos, which, in a stroke model using mice, promoted cerebral angiogenesis and aided in the neurological recovery following an ischemic stroke. This approach highlights the potential for using biomaterials to enhance the therapeutic effects of NSC-derived Exos in stroke treatment [72]. The researchers also compared the exosomes derived from neural stem cells with those derived from mesenchymal stem cells. The results showed that exosomes derived from neural stem cells improved middle-aged rodents’ cells, tissues, and functions. However, exosomes generated from mesenchymal stem cells had a weak effect [73]. There are few studies on nerve stem-derived exosomes in the treatment of stroke. And the mechanism of this kind of exosomes in the treatment of stroke is still unclear. According to the current therapeutic effect, it is worth further study by researchers (Fig. 3).
Figure 3: Exosomes secreted by stem cells act on target cells through paracellular effects to produce therapeutic effects on the nervous system, such as promoting neural remodeling and neovascularization, repairing the damaged blood-brain barrier, and counteracting neuroinflammation, etc. (By Figdraw). Abbreviations: ESCs, Embryonic stem cells; iPSCs, Induced pluripotent stem cells; MVE, Multivesicular endosome; ER, Endoplasmic reticulum.
In pathophysiology, we know that neurodegenerative diseases are caused by the loss of neurons or myelin sheath. Since neurodegenerative diseases are caused by the loss of neurons or myelin sheath, it makes sense to use neural stem cells to turn into neurons that match the disease’s symptoms and pathological processes. Because of this, researchers used exosomes produced by NSCs to treat neurodegenerative diseases, and their research showed that NSCs-derived exosomes worked well.
In the Parkinson’s disease (PD) model, Lee et al. found that NSC-derived exosomes have potential protective effects in vivo and in vitro. It stops PD by lowering the number of reactive oxygen species inside cells and the related apoptotic pathways [74]. In addition, the exosomes induce the down-regulation of inflammatory factors and reduce the loss of dopamine neurons in vivo. In AD, the blood-brain barrier will be damaged to a certain extent. Liu et al. used NSC-derived exosomes to reverse the BBB defect caused by AD [75]. In terms of symptom relief, Apodaca et al. used NSCs-derived exosomes through retroorbital vein injection to improve the symptoms of AD mice, such as fear resolution, memory consolidation, and reduction of anxiety-related behaviors. In addition to symptoms, the team also found that exosomes treated AD mice with dense core amyloid beta plaque accumulation and microglial activation. These results are linked to the fact that proinflammatory cytokines have partially returned to normal levels in the blood of AD mice. Significantly, exosome treatment reduced synaptic loss associated with cognitive improvement in the brain [76]. In addition, Li et al. also found that NSCs-derived exosomes can improve the cognitive ability of AD mice, enhance mitochondrial function, sirtuin 1 (SIRT1) activation, and synaptic activity, reduce the inflammatory response, and improve the progression in AD mice [77]. Mitochondrial dysfunction is indicative of Alzheimer’s disease. NSCs-derived exosomes have been demonstrated to increase the levels of SIRT1, enhance the production of factors related to mitochondrial biogenesis, inhibit the activation of astrocytes, and reduce the production of amyloid-β. These exosomes activate the SIRT1-peroxisome proliferator-activated receptor-γ coactivator-1α (PGC1α) signaling pathway and promote the synthesis of nuclear respiratory factor 1 (NRF1) and cytochrome C oxidase IV (COXIV), which in turn facilitates mitochondrial biogenesis [77]. NSCs-derived exosomes not only improve the cognitive and memory functions of neurodegenerative diseases but also delay pathological changes. When its mechanism of action is further clarified, it will become one of the important strategies for the treatment of neurodegenerative diseases.
Spinal cord injury (SCI) is similar to traumatic brain injury (TBI). SCI is a neurological disease with a poor prognosis and a low degree of functional recovery. It seriously affects the quality of life of patients and their families. However, the current treatment of spinal cord injury is limited. The pathological changes after spinal cord injury can be divided into primary and secondary. The initial stage is the initial damage caused by the impact, followed by rapid and gradual secondary damage. During this stage of spinal cord injury, glial cells and nerve cells die because of many things, such as edema, neuroinflammation, excitotoxicity, etc. Therefore, stem cell transplantation is one of the most promising treatment strategies for spinal cord injury. Many studies have shown that stem cells and their derivatives can change the pathological environment and promote the regeneration of damaged neurons, regenerating axons, nutritional support, or a combination of both [78,79]. NSCs-derived exosomes were used to treat SCI, and researchers have made some achievements in this field.
Rong et al. found that NSCs-derived exosomes can significantly improve the degree of spinal cord injury and promote functional recovery in SCI rats. Those exosomes can regulate early neuronal apoptosis and inhibit the inflammatory processes in SCI model rats by inducing autophagy. NSCs-derived exosomes were found to contain 14-3-3T protein, which was identified as a critical component in the mechanism by which NSCs-derived exosomes repair spinal cord injury via autophagy. The overexpression of 14-3-3T protein enhanced the anti-apoptotic and anti-inflammatory effects of NSC-derived exosomes and further promoted the recovery of functional behavior after SCI [22,80]. In addition, Zhong et al. found that NSCs-derived exosomes can promote the migration, proliferation, and tubular formation of spinal cord microvascular cells in vitro. In vivo experiments, neural stem cell-derived exosomes promoted the increase of local microvascular regeneration, reduced syringomyelia, and improved the recovery of limb function in SCI mice, which may be related to vascular endothelial growth factor A (VEGF-A) carrying. However, the specific mechanism has not been further studied [81]. For SCI therapy, NSCs-derived exosomes may be one of the options for cell-free therapy.
Researchers use NSCs-derived exosomes for the investigation of a wide variety of disorders, not just those affecting the central nervous system. The current research results showed that NSCs-derived exosomes also have a good effect on other diseases. In the study of glioma, Adamus et al. injected NSC-derived exosomes encapsulated antisense oligonucleotides into mice, which inhibited the growth of subcutaneous tumors more effectively than the same number of oligonucleotides alone. They enhanced the anti-tumor effect on mouse glioma [82]. In the experiment of cardiac infarction, Katsur et al. found that NSCs-derived exosomes delayed the opening of myocardial mitochondrial permeability transition pores in cell experiments and reduced the infarct size in mice with myocardial infarction in animal experiments [50]. We don’t know enough about the molecular pathways that overnutrition and metabolic diseases hurt cognitive functioning. Spinelli et al. intranasally injected NSCs-derived exosomes into high-fat diet mice. They found that NSCs-derived exosomes reversed memory impairment associated with a high-fat diet through the cAMP response element-binding protein (CREB)-BDNF signaling pathway, providing evidence for the potential therapeutic effect of NSCs-derived exosomes on cognitive impairment associated with metabolic diseases [83]. Zhang et al. found that NSCs in the hypothalamus control the rate of aging through miRNAs of exosomes [56]. Li et al. demonstrated that NSC-Exo displays therapeutic efficacy in promoting wound healing. Additionally, the study revealed that NSC-Exo enhances the migration of human dermal fibroblasts (HDF) cells and tube formation of human umbilical vein endothelial cells (HUVECs). Furthermore, in vivo studies on a mouse model of skin injury revealed that NSC-Exo accelerates wound healing. The potential underlying mechanism may involve the regulation of inflammation and the function of neural growth factors, such as neuro-derived neurotrophic factor (NDNF) [84].
NSCs-derived exosomes, possessing inherent advantages such as low immunogenicity, favorable biocompatibility, and targeted delivery capabilities, have emerged as promising therapeutic candidates for a wide array of diseases (Table 1). These include, but are not limited to, neurological disorders, cardiovascular diseases, metabolic conditions, cognitive impairments, and compromised tissue repair. The above studies have elucidated that the therapeutic efficacy of NSC exosomes stems from their multi-faceted mechanisms of action. These encompass the secure delivery of therapeutic agents across biological barriers, the preservation and transport of bioactive molecules such as miRNAs, proteins, and growth factors, and the intricate modulation of cellular functions. Notably, NSC exosomes have demonstrated the capacity to promote cell survival and regeneration, mitigate inflammatory responses, and fine-tune cell signaling pathways. While the majority of research on NSC exosomes remains preclinical, their multifaceted therapeutic potential underscores their promise as novel therapeutics or adjunctive treatment modalities. Future investigations focused on unraveling the intricacies of their mechanisms of action and optimizing treatment strategies will be pivotal in propelling their clinical translation and unlocking their full therapeutic benefit for patients.
Study Other Directions of Exosomes Derived from Neural Stem Cells
As research gets more in-depth, the problems with using NSCs-derived exosomes to treat diseases are slowly coming to light [87,88]. First, the targeting ability of the NSCs-derived exosomes is poor. After exosomes are injected into the body fluid circulation, most of the transplanted exosomes are intercepted by organs such as the liver and lung, thus reducing the number of exosomes reaching the diseased area and reducing the therapeutic effect. Second, the loaded content of exosomes cannot reach an effective therapeutic concentration. The exosomes are processed and assembled in donor cells and contain most of the bioactive substances of donor cells, but the load content of functional molecules used by exosomes for therapeutic purposes is relatively low.
Various transformation mechanisms have been devised to increase the targeting, stability, and loading capacity of exosomes, as a result of the mentioned shortcomings of natural exosomes. Exosomes that have been edited by these procedures are referred to as “engineering exosomes”. The modification involves preparing engineered exosomes carrying target miRNA, drugs, siRNA, and proteins via electroporation, incubation, chemical conjugation, and other techniques. In addition, specially engineered exosomes can also be obtained by changing donor cells’ growth environment and genetic material.
The development of innovative biomaterials, such as hydrogels and nanoparticles, has created new opportunities for precise exosome delivery. Sun et al. reported the development of a novel method utilizing silk fibroin cryosponges for the sustained and regulated release of exosomes within engineered tissues. These cryosponges function by employing an enzymatic response mechanism to regulate the release kinetics of exosomes. Notably, in vivo experiments showed that exosomes remained active within the undigested sponge matrix for two full months, outlasting the retention capabilities of commonly utilized fibrin glue in clinical settings. The utilization of exosome-laden sponges demonstrated effective angiogenesis and tissue ingrowth, thereby validating the efficacy of this method in achieving sustained release while preserving the bioactivity of the exosomes [89]. In yet another investigation, Gu et al. integrated neural stem cell-derived exosomes into an injectable hyaluronic acid hydrogel. Direct stereotactic injection of hydrogels into the ischemic core enables the achievement of sustained exosome release at the site of injury [72]. Zhou et al. created modular satellite nanoparticles that employed electrostatic interactions to immobilize negatively charged macrophage membranes and exosomes onto positively charged nanoparticles. These nanoparticles were designed to take advantage of the natural targeting ability of macrophage membranes and the blood-brain barrier permeability of the nanoparticles, facilitating targeted delivery of exosomes to cerebral ischemic regions and enhancing their therapeutic effectiveness [90]. The following examples demonstrate the significant potential of biomaterials in the precise and directed delivery of exosomes. As materials science continues to advance and our understanding of disease development deepens, it is anticipated that even more advanced biomaterials will be developed, leading to improved and safer exosome delivery methods and expediting the translation of exosome-based therapies into clinical applications.
Using exosomes made from NSCs to treat neurological and other human diseases has sparked much hope, but it also comes with a few problems.
There are still many problems in the preparation of exosomes. First, the current preparation method for exosomes mainly uses techniques such as ultra-high-speed centrifugation to separate and clean exosomes [91]. The disadvantage of this method is that it takes a long time to purify exosomes and the number of exosomes obtained is very limited. Although there are some advanced methods that may have the potential for large-scale extraction of exosomes, such as tangential flow filtration (The direction of liquid flow is perpendicular to the direction of filtration) or size exclusion chromatography (Particle separated only according to the volume of molecules), these methods are still in the research stage. The clinical application of exosomes will inevitably use a large number of exosomes, and the large-scale preparation of exosomes will be a major clinical technical problem. Second, Identification of exocrine bodies. In cell culture, it is unavoidable to use medium supplements, such as fetal bovine serum. Exosomes are also contained in fetal bovine serum, so standard characterization of desired exosomes is necessary. Third, when exosomes are applied clinically, the problem that must be solved is the dosage and specific mechanism of exosomes. Determining the optimal dose of NSC-derived exosomes starts with understanding how they work. This may help to deepen our understanding of the heterogeneity of NSC-derived exosomes and improve purification protocols. After the above problems are solved, we may be able to obtain more exosomes with better functional activity. As for whether NSCs-derived exosomes can have a good therapeutic effect on different types of neurological diseases, giving specific biological activity to NSCs-derived exosomes as a marker of therapeutic efficacy is a possible way to solve this problem.
Making NSCs-derived exosomes into drug-loading systems or engineered exosomes is part of the current research direction of NSC-derived exosomes. In the above research directions, exogenous substances will be loaded into exosomes. Whether these loaded exogenous substances will interfere with endogenous substances, thereby causing off-target effects (i.e., drug side effects). This question deserves deep consideration by researchers. The clinical use of exosomes is hindered when the effects of off-target effects outweigh the therapeutic benefits of exosomes. There are many substances in exosomes. When exosomes are loaded with drugs or engineered, whether the components in exosomes have changed, and whether these changed substances will have adverse effects on the human body, which deserves further clarification.
Exosomes are secreted by cells. When cells contain toxic components, exosomes may contain some toxic components, so it is very important to choose a safe cell source. In order to solve these problems, it is necessary to select suitable test objects and prepare the most suitable exocrine agents in clinical trials. A thorough understanding of the mechanism of exosomes can better guide the selection of exosomes.
To better target the exosomes to the desired area, improve the efficacy of exosomes in treating diseases, and reduce the targeting effect, researchers have come up with different solutions to these problems. Currently, many researchers use stereotactic, intravenous injection, aerosol inhalation-intranasal administration, and other methods. However, different ways of transplantation have their advantages and disadvantages. For example, the stereotactic injection can accurately inject the exosomes to a specific site, which will inevitably damage the skull and brain tissue. Intravenous injection, aerosol inhalation, and other methods are non-invasive, but before the exosomes enter the lesion area, there has been much loss (Much of the exosomes are intercepted by the liver and lungs). Only a tiny part of the exosomes entered the focus. A better way might be to inject exosomes via arteries, which are less likely to be intercepted by organs. However, for multiple exosome injections, the arterial injection may need further improvement. Similar to the limitations of stem cell therapy, the limited accumulation of exosomes in the damaged brain poses a challenge to its clinical application. In order to overcome this obstacle, intranasal administration has become a non-invasive and effective way to deliver drugs to the central nervous system. By using smell and trigeminal axons, this method can bypass the blood-brain barrier and deliver therapeutic drugs directly to the brain. It is worth noting that the exosome can easily enter the neural pathway using this method because of its small size [92] (Fig. 4). At this time, researchers need to find a better mode of drug administration that requires targeting exosomes to focus on areas with sufficient quantities. This is more beneficial for improving the therapeutic effect of exosomes because it also reduces the off-targeted effect.
Figure 4: Various injection methods of exosomes and some of their disadvantages, such as intravenous injection, aerosol inhalation (organ interception), arterial injection (cannot be injected in the same site multiple times), stereotactic injection (brain injury, skull injury) (By Figdraw).
Numerous recent advances have been made in researching exosomes generated from NSCs, even though many obstacles remain. There is currently preclinical use of exosomes produced from NSCs. According to an increasing number of preclinical investigations, NSCs-derived exosome therapy has also been shown to hold promise in treating a wide range of illnesses, according to an increasing number of preclinical investigations. Compared to other types of stem cell-derived exocrine bodies, NSC-derived exosomes exhibit a greater affinity for the nervous system. Furthermore, as previously stated, NSCs-derived exosomes possess the ability to regulate neural stem cells, more effectively activate dormant neural stem cells in the brain, and encourage neural stem cells to participate in the repair of the nervous system.
Exosomes derived from neural stem cells unveil immense therapeutic potential, particularly in the realm of neurological disorders. This comprehensive review delves deeply into the therapeutic advantages and potential mechanisms of action of neural stem cell exosomes, summarizing their research progress in neurological and other diseases. Nevertheless, the review exhibits some shortcomings, such as a lack of comparative analysis of different administration routes. Furthermore, despite exosomes demonstrating vast potential in the treatment of neurological disorders, their current clinical application remains restricted. Standardization of the production, purification, and storage processes of exosomes is imperative to ensure treatment consistency and efficacy. Further exploration of the distribution, metabolism, and excretion mechanisms of exosomes in vivo will aid in optimizing their clinical application. The significance of this study lies in emphasizing the potential of exosomes in the treatment of neurological disorders, providing a clear direction for future research aimed at enhancing treatment outcomes through the improvement of exosome production and administration methods, as well as the development of targeted strategies. However, the clinical translation and application of neural stem cell exosome therapy still face challenges. Future research should focus on refining production and administration methods, delving deeper into mechanisms, and advancing clinical trials to promote a broader application of exosomes in clinical settings. It is believed that through thorough investigation of the aforementioned key issues, neural stem cell exosomes are poised to be applied in clinical practice soon, bringing new hope to patients.
Acknowledgement: None.
Funding Statement: This work was partially sponsored by the National Key R&D Program of China (2022YFA1104900 and 2022YFA1104904), the Innovation Team Project of General Universities in Guangdong Province (2023KCXTD007), the Special Project in Key Fields of General Universities in Guangdong Province (2021ZDZX2011), the President Foundation of Southern Medical University Hospital of Integrated Traditional Chinese and Western Medicine (1202101003).
Author Contributions: The authors confirm contribution to the paper as follows: study conception and design: Lukui Chen; literatures collection: Jiajun Huang, Rong Li, Wentong Lin, Yifan Zhang, Zhinuo Li; analysis and interpretation of results: Jiajun Huang, Zhihan Zhu, Wei Wang, Jiale Liu; draft manuscript preparation: Jiajun Huang, Zhihan Zhu, Wei Wang, Qiankun Zhang, Jiale Liu; manuscript revised: Waqas Ahmed, Deng Lu, Yong Hu, Ahasn Ali Khan, Hengsen Cai. All authors reviewed the results and approved the final version of the manuscript.
Availability of Data and Materials: All data generated during this study are included in this published article.
Ethics Approval: Not applicable.
Conflicts of Interest: The authors declare that they have no conflicts of interest to report regarding the present study.
References
1. Adams KV, Morshead CM. Neural stem cell heterogeneity in the mammalian forebrain. Prog Neurobiol. 2018;170(4):2–36. doi:10.1016/j.pneurobio.2018.06.005. [Google Scholar] [PubMed] [CrossRef]
2. Gage FH, Temple S. Neural stem cells: generating and regenerating the brain. Neuron. 2013;80(3):588–601. doi:10.1016/j.neuron.2013.10.037. [Google Scholar] [PubMed] [CrossRef]
3. Englund C, Fink A, Lau C, Pham D, Daza RA, Bulfone A, et al. Pax6, Tbr2, and Tbr1 are expressed sequentially by radial glia, intermediate progenitor cells, and postmitotic neurons in developing neocortex. J Neurosci. 2005;25(1):247–51. doi:10.1523/JNEUROSCI.2899-04.2005. [Google Scholar] [PubMed] [CrossRef]
4. Noctor SC, Flint AC, Weissman TA, Dammerman RS, Kriegstein AR. Neurons derived from radial glial cells establish radial units in neocortex. Nature. 2001;409(6821):714–20. doi:10.1038/35055553. [Google Scholar] [PubMed] [CrossRef]
5. Cheung TH, Rando TA. Molecular regulation of stem cell quiescence. Nat Rev Mol Cell Biol. 2013;14(6):329–40. doi:10.1038/nrm3591. [Google Scholar] [PubMed] [CrossRef]
6. Ihrie RA, Alvarez-Buylla A. Lake-front property: a unique germinal niche by the lateral ventricles of the adult brain. Neuron. 2011;70(4):674–86. doi:10.1016/j.neuron.2011.05.004. [Google Scholar] [PubMed] [CrossRef]
7. Ming GL, Song H. Adult neurogenesis in the mammalian brain: significant answers and significant questions. Neuron. 2011;70(4):687–702. doi:10.1016/j.neuron.2011.05.001. [Google Scholar] [PubMed] [CrossRef]
8. Yao J, Mu Y, Gage FH. Neural stem cells: mechanisms and modeling. Protein Cell. 2012;3(4):251–61. doi:10.1007/s13238-012-2033-6. [Google Scholar] [PubMed] [CrossRef]
9. Assinck P, Duncan GJ, Plemel JR, Lee MJ, Stratton JA, Manesh SB, et al. Myelinogenic plasticity of oligodendrocyte precursor cells following spinal cord contusion injury. J Neurosci. 2017;37(36):8635–54. doi:10.1523/JNEUROSCI.2409-16.2017. [Google Scholar] [PubMed] [CrossRef]
10. Huang H, Young W, Chen L, Feng S, Zoubi ZMA, Sharma HS, et al. Clinical cell therapy guidelines for neurorestoration (IANR/CANR 2017). Cell Transplant. 2018;27(2):310–24. doi:10.1177/0963689717746999. [Google Scholar] [PubMed] [CrossRef]
11. Stonesifer C, Corey S, Ghanekar S, Diamandis Z, Acosta SA, Borlongan CV. Stem cell therapy for abrogating stroke-induced neuroinflammation and relevant secondary cell death mechanisms. Prog Neurobiol. 2017;158:94–131. [Google Scholar] [PubMed]
12. Malliaras K, Li TS, Luthringer D, Terrovitis J, Cheng K, Chakravarty T, et al. Safety and efficacy of allogeneic cell therapy in infarcted rats transplanted with mismatched cardiosphere-derived cells. Circulation. 2012;125(1):100–12. [Google Scholar] [PubMed]
13. Marban E. The secret life of exosomes: what bees can teach us about next-generation therapeutics. J Am Coll Cardiol. 2018;71(2):193–200. [Google Scholar] [PubMed]
14. Gong YH, Wang YM, Qu Q, Hou ZK, Guo TW, Xu YQ, et al. Nanoparticle encapsulated core-shell hydrogel for on-site BMSCs delivery protects from iron overload and enhances functional recovery. J Control Release. 2020;320:381–91. [Google Scholar] [PubMed]
15. Liu Y, Hsu YH, Huang APH, Hsu SH. Semi-interpenetrating polymer network of hyaluronan and chitosan self-healing hydrogels for central nervous system repair. Acs Appl Mater Inter. 2020;12(36):40108–20. [Google Scholar]
16. Long J, Gu CY, Zhang QK, Liu JL, Huang JJ, Li YJ, et al. Extracellular vesicles from medicated plasma of Buyang Huanwu decoction-preconditioned neural stem cells accelerate neurological recovery following ischemic stroke. Front Cell Dev Biol. 2023;11:1096329. doi:10.3389/fcell.2023.1096329. [Google Scholar] [PubMed] [CrossRef]
17. Baulch JE, Acharya MM, Allen BD, Ru N, Chmielewski NN, Martirosian V, et al. Cranial grafting of stem cell-derived microvesicles improves cognition and reduces neuropathology in the irradiated brain. Proc Natl Acad Sci U S A. 2016;113(17):4836–41. doi:10.1073/pnas.1521668113. [Google Scholar] [PubMed] [CrossRef]
18. Leavitt RJ, Acharya MM, Baulch JE, Limoli CL. Extracellular vesicle-derived miR-124 resolves radiation-induced brain injury. Cancer Res. 2020;80(19):4266–77. doi:10.1158/0008-5472.CAN-20-1599. [Google Scholar] [PubMed] [CrossRef]
19. Smith SM, Giedzinski E, Angulo MC, Lui T, Lu C, Park AL, et al. Functional equivalence of stem cell and stem cell-derived extracellular vesicle transplantation to repair the irradiated brain. Stem Cells Transl Med. 2020;9(1):93–105. doi:10.1002/sctm.18-0227. [Google Scholar] [PubMed] [CrossRef]
20. Li B, Liu J, Gu G, Han X, Zhang Q, Zhang W. Impact of neural stem cell-derived extracellular vesicles on mitochondrial dysfunction, sirtuin 1 level, and synaptic deficits in Alzheimer’s disease. J Neurochem. 2020;154(5):502–18. doi:10.1111/jnc.15001. [Google Scholar] [PubMed] [CrossRef]
21. Losurdo M, Pedrazzoli M, D’Agostino C, Elia CA, Massenzio F, Lonati E, et al. Intranasal delivery of mesenchymal stem cell-derived extracellular vesicles exerts immunomodulatory and neuroprotective effects in a 3xTg model of Alzheimer’s disease. Stem Cells Transl Med. 2020;9(9):1068–84. doi:10.1002/sctm.19-0327. [Google Scholar] [PubMed] [CrossRef]
22. Rong Y, Liu W, Wang J, Fan J, Luo Y, Li L, et al. Neural stem cell-derived small extracellular vesicles attenuate apoptosis and neuroinflammation after traumatic spinal cord injury by activating autophagy. Cell Death Dis. 2019;10(5):340. doi:10.1038/s41419-019-1571-8. [Google Scholar] [PubMed] [CrossRef]
23. Ahmed W, Kuniyan MS, Jawed AM, Chen L. Engineered extracellular vesicles for drug delivery in therapy of stroke. Pharmaceutics. 2023;15(9):2173. doi:10.3390/pharmaceutics15092173. [Google Scholar] [PubMed] [CrossRef]
24. Raposo G, Nijman HW, Stoorvogel W, Liejendekker R, Harding CV, Melief CJ, et al. B lymphocytes secrete antigen-presenting vesicles. J Exp Med. 1996;183(3):1161–72. doi:10.1084/jem.183.3.1161. [Google Scholar] [PubMed] [CrossRef]
25. Théry C, Amigorena S, Raposo G, Clayton A. Isolation and characterization of exosomes from cell culture supernatants and biological fluids. Curr Protoc Cell Biol. 2006;30(1):3–22. doi:10.1002/0471143030.cb0322s30. [Google Scholar] [PubMed] [CrossRef]
26. Lotvall J, Valadi H. Cell to cell signalling via exosomes through esRNA. Cell Adh Migr. 2007;1(3):156–8. [Google Scholar] [PubMed]
27. Valadi H, Ekstrom K, Bossios A, Sjostrand M, Lee JJ, Lotvall JO. Exosome-mediated transfer of mRNAs and microRNAs is a novel mechanism of genetic exchange between cells. Nat Cell Biol. 2007;9(6):654–9. [Google Scholar] [PubMed]
28. Feng J, Waqas A, Zhu Z, Chen L. Exosomes: applications in respiratory infectious diseases and prospects for coronavirus disease 2019 (COVID-19). J Biomed Nanotechnol. 2020;16(4):399–418. [Google Scholar] [PubMed]
29. Fang SB, Zhang HY, Wang C, He BX, Liu XQ, Meng XC, et al. Small extracellular vesicles derived from human mesenchymal stromal cells prevent group 2 innate lymphoid cell-dominant allergic airway inflammation through delivery of miR-146a-5p. J Extracell Vesicles. 2020;9(1):1723260. [Google Scholar] [PubMed]
30. Feng J, Zhang Y, Zhu Z, Gu C, Waqas A, Chen L. Emerging exosomes and exosomal MiRNAs in spinal cord injury. Front Cell Dev Biol. 2021;9:703989. [Google Scholar] [PubMed]
31. Kim H, Kim EH, Kwak G, Chi SG, Kim SH, Yang Y. Exosomes: cell-derived nanoplatforms for the delivery of cancer therapeutics. Int J Mol Sci. 2020;22(1):14. [Google Scholar] [PubMed]
32. Fu W, Lei C, Liu S, Cui Y, Wang C, Qian K, et al. CAR exosomes derived from effector CAR-T cells have potent antitumour effects and low toxicity. Nat Commun. 2019;10(1):4355. [Google Scholar] [PubMed]
33. Wu P, Zhang B, Ocansey DKW, Xu W, Qian H. Extracellular vesicles: a bright star of nanomedicine. Biomaterials. 2021;269:120467. [Google Scholar] [PubMed]
34. Chen G, Huang AC, Zhang W, Zhang G, Wu M, Xu W, et al. Exosomal PD-L1 contributes to immunosuppression and is associated with anti-PD-1 response. Nature. 2018;560(7718):382–6. [Google Scholar] [PubMed]
35. Lotvall J, Hill AF, Hochberg F, Buzas EI, Di Vizio D, Gardiner C, et al. Minimal experimental requirements for definition of extracellular vesicles and their functions: a position statement from the international society for extracellular vesicles. J Extracell Vesicles. 2014;3:26913. [Google Scholar] [PubMed]
36. Xu R, Rai A, Chen M, Suwakulsiri W, Greening DW, Simpson RJ. Extracellular vesicles in cancer-implications for future improvements in cancer care. Nat Rev Clin Oncol. 2018;15(10):617–38. doi:10.1038/s41571-018-0036-9. [Google Scholar] [PubMed] [CrossRef]
37. Hoshino A, Costa-Silva B, Shen TL, Rodrigues G, Hashimoto A, Tesic Mark M, et al. Tumour exosome integrins determine organotropic metastasis. Nature. 2015;527(7578):329–35. doi:10.1038/nature15756. [Google Scholar] [PubMed] [CrossRef]
38. Janas T, Janas MM, Sapon K, Janas T. Mechanisms of RNA loading into exosomes. FEBS Lett. 2015;589(13):1391–8. doi:10.1016/j.febslet.2015.04.036. [Google Scholar] [PubMed] [CrossRef]
39. Ager RR, Davis JL, Agazaryan A, Benavente F, Poon WW, LaFerla FM, et al. Human neural stem cells improve cognition and promote synaptic growth in two complementary transgenic models of Alzheimer’s disease and neuronal loss. Hippocampus. 2015;25(7):813–26. doi:10.1002/hipo.22405. [Google Scholar] [PubMed] [CrossRef]
40. Goldberg NRS, Marsh SE, Ochaba J, Shelley BC, Davtyan H, Thompson LM, et al. Human neural progenitor transplantation rescues behavior and reduces alpha-synuclein in a transgenic model of dementia with lewy bodies. Stem Cells Transl Med. 2017;6(6):1477–90. doi:10.1002/sctm.16-0362. [Google Scholar] [PubMed] [CrossRef]
41. Hou B, Ma J, Guo X, Ju F, Gao J, Wang D, et al. Exogenous neural stem cells transplantation as a potential therapy for photothrombotic ischemia stroke in kunming mice model. Mol Neurobiol. 2017;54(2):1254–62. doi:10.1007/s12035-016-9740-6. [Google Scholar] [PubMed] [CrossRef]
42. Tang Y, Wang J, Lin X, Wang L, Shao B, Jin K, et al. Neural stem cell protects aged rat brain from ischemia-reperfusion injury through neurogenesis and angiogenesis. J Cereb Blood Flow Metab. 2014;34(7):1138–47. doi:10.1038/jcbfm.2014.61. [Google Scholar] [PubMed] [CrossRef]
43. Ni W, Ramalingam M, Li YM, Park JH, Dashnyam K, Lee JH, et al. Immunomodulatory and anti-inflammatory effect of neural stem/progenitor cells in the central nervous system. Stem Cell Rev Rep. 2023;19(4):866–85. doi:10.1007/s12015-022-10501-1. [Google Scholar] [PubMed] [CrossRef]
44. Ottoboni L, von Wunster B, Martino G. Therapeutic plasticity of neural stem cells. Front Neurol. 2020;11:7. doi:10.3389/fneur.2020.00148. [Google Scholar] [PubMed] [CrossRef]
45. De Gioia R, Biella F, Citterio G, Rizzo F, Abati E, Nizzardo M, et al. Neural stem cell transplantation for neurodegenerative diseases. Int J Mol Sci. 2020;21(9):3103. doi:10.3390/ijms21093103. [Google Scholar] [PubMed] [CrossRef]
46. Ma Y, Xu X, Li C, Wang Y, Zhu J, Xia X, et al. Induced neural progenitor cell-derived extracellular vesicles promote neural progenitor cell survival via extracellular signal-regulated kinase pathway. CNS Neurosci Ther. 2021;27(12):1605–9. doi:10.1111/cns.13744. [Google Scholar] [PubMed] [CrossRef]
47. Stronati E, Conti R, Cacci E, Cardarelli S, Biagioni S, Poiana G. Extracellular vesicle-induced differentiation of neural stem progenitor cells. Int J Mol Sci. 2019;20(15):3691. doi:10.3390/ijms20153691. [Google Scholar] [PubMed] [CrossRef]
48. Yuan P, Ding L, Chen H, Wang Y, Li C, Zhao S, et al. Neural stem cell-derived exosomes regulate neural stem cell differentiation through miR-9-Hes1 axis. Front Cell Dev Biol. 2021;9:601600. doi:10.3389/fcell.2021.601600. [Google Scholar] [PubMed] [CrossRef]
49. Liu JL, Lin CQ, Gu CY, Zhang QK, Feng TL, Duan WJ, et al. Mechanism of neural stem cell-derived exosomal miR-9a-5p overexpression improving survival and neurogenesis in ischemic stroke rats. J Biomed Nanotechnol. 2023;19(11):1963–78. doi:10.1166/jbn.2023.3710. [Google Scholar] [CrossRef]
50. Katsur M, He Z, Vinokur V, Corteling R, Yellon DM, Davidson SM. Exosomes from neuronal stem cells may protect the heart from ischaemia/reperfusion injury via JAK1/2 and gp130. J Cell Mol Med. 2021;25(9):4455–65. doi:10.1111/jcmm.16515. [Google Scholar] [PubMed] [CrossRef]
51. Alcedo J, Kenyon C. Regulation of C. elegans longevity by specific gustatory and olfactory neurons. Neuron. 2004;41(1):45–55. doi:10.1016/S0896-6273(03)00816-X. [Google Scholar] [PubMed] [CrossRef]
52. Riera CE, Huising MO, Follett P, Leblanc M, Halloran J, Van Andel R, et al. TRPV1 pain receptors regulate longevity and metabolism by neuropeptide signaling. Cell. 2014;157(5):1023–36. doi:10.1016/j.cell.2014.03.051. [Google Scholar] [PubMed] [CrossRef]
53. Zhang G, Li J, Purkayastha S, Tang Y, Zhang H, Yin Y, et al. Hypothalamic programming of systemic ageing involving IKK-β, NF-κB and GnRH. Nature. 2013;497(7448):211–6. doi:10.1038/nature12143. [Google Scholar] [PubMed] [CrossRef]
54. Sadagurski M, Landeryou T, Cady G, Bartke A, Bernal-Mizrachi E, Miller RA. Transient early food restriction leads to hypothalamic changes in the long-lived crowded litter female mice. Physiol Rep. 2015;3(4):e12379. doi:10.14814/phy2.12379. [Google Scholar] [PubMed] [CrossRef]
55. Natale F, Leone L, Rinaudo M, Sollazzo R, Barbati SA, La Greca F, et al. Neural stem cell-derived extracellular vesicles counteract insulin resistance-induced senescence of neurogenic niche. Stem Cells. 2022;40(3):318–31. doi:10.1093/stmcls/sxab026. [Google Scholar] [PubMed] [CrossRef]
56. Zhang Y, Kim MS, Jia B, Yan J, Zuniga-Hertz JP, Han C, et al. Hypothalamic stem cells control ageing speed partly through exosomal miRNAs. Nature. 2017;548(7665):52–7. [Google Scholar] [PubMed]
57. Ma Q, Li R, Wang L, Yin P, Wang Y, Yan C, et al. Temporal trend and attributable risk factors of stroke burden in China, 1990–2019: an analysis for the global burden of disease study 2019. Lancet Public Health. 2021;6(12):e897–906. [Google Scholar] [PubMed]
58. Hughes D, Judge C, Murphy R, Loughlin E, Costello M, Whiteley W, et al. Association of blood pressure lowering with incident dementia or cognitive impairment: a systematic review and meta-analysis. JAMA. 2020;323(19):1934–44. [Google Scholar] [PubMed]
59. Marko M, Posekany A, Szabo S, Scharer S, Kiechl S, Knoflach M, et al. Trends of r-tPA (Recombinant tissue-type plasminogen activator) treatment and treatment-influencing factors in acute ischemic stroke. Stroke. 2020;51(4):1240–7. [Google Scholar] [PubMed]
60. Goldstein LB. Acute ischemic stroke treatment in 2007. Circulation. 2007;116(13):1504–14. [Google Scholar] [PubMed]
61. Zhang G, Chen L, Guo X, Wang H, Chen W, Wu G, et al. Comparative analysis of microRNA expression profiles of exosomes derived from normal and hypoxic preconditioning human neural stem cells by next generation sequencing. J Biomed Nanotechnol. 2018;14(6):1075–89. doi:10.1166/jbn.2018.2567. [Google Scholar] [PubMed] [CrossRef]
62. Zhu Z, Kalyan BS, Chen L. Therapeutic potential role of exosomes for ischemic stroke. Brain Sci Adv. 2019;5(2):128–43. doi:10.1177/2096595820902588. [Google Scholar] [CrossRef]
63. Zhao X, Zhu J, Chen S, Liu R, Long T. Neural stem cell-derived exosomes improve neurological function in rats with cerebral ischemia-reperfusion injury by regulating microglia-mediated inflammatory response. J Inflamm Res. 2023;16:3079–92. doi:10.2147/JIR.S414121. [Google Scholar] [PubMed] [CrossRef]
64. Li B, Wei H-Y, Yang Y, Yin M-X, Hu C-L, Lu Y, et al. Neural stem cell-derived exosomes inhibit apoptosis of neurons induced by hypoxia neural cells. Chin J Pathophysiol. 2018;34(4):717–22+28 (In Chinese). [Google Scholar]
65. Deng X, Hu X, Wang S, Zhao H, Wei Y, Fu J, et al. Neural stem cell-derived exosomes regulate cell proliferation, migration, and cell death of brain microvascular endothelial cells via the miR-9/Hes1 axis under hypoxia. Animal Model Exp Med. 2024;7(1):24–35. doi:10.1002/ame2.12394. [Google Scholar] [PubMed] [CrossRef]
66. Mahdavipour M, Hassanzadeh G, Seifali E, Mortezaee K, Aligholi H, Shekari F, et al. Effects of neural stem cell-derived extracellular vesicles on neuronal protection and functional recovery in the rat model of middle cerebral artery occlusion. Cell Biochem Funct. 2020;38(4):373–83. doi:10.1002/cbf.3484. [Google Scholar] [PubMed] [CrossRef]
67. Sun X, Jung JH, Arvola O, Santoso MR, Giffard RG, Yang PC, et al. Stem cell-derived exosomes protect astrocyte cultures from in vitro ischemia and decrease injury as post-stroke intravenous therapy. Front Cell Neurosci. 2019;13:394. doi:10.3389/fncel.2019.00394. [Google Scholar] [PubMed] [CrossRef]
68. Tian T, Cao L, He C, Ye Q, Liang R, You W, et al. Targeted delivery of neural progenitor cell-derived extracellular vesicles for anti-inflammation after cerebral ischemia. Theranostics. 2021;11(13):6507–21. doi:10.7150/thno.56367. [Google Scholar] [PubMed] [CrossRef]
69. Li Z, Chen Z, Peng J. Neural stem cell-derived exosomal FTO protects neuron from microglial inflammatory injury by inhibiting microglia NRF2 mRNA m6A modification. J Neurogenet. 2023;37(3):103–14. doi:10.1080/01677063.2023.2259995. [Google Scholar] [PubMed] [CrossRef]
70. Zhang G, Zhu Z, Wang H, Yu Y, Chen W, Waqas A, et al. Exosomes derived from human neural stem cells stimulated by interferon gamma improve therapeutic ability in ischemic stroke model. J Adv Res. 2020;24(17):435–45. doi:10.1016/j.jare.2020.05.017. [Google Scholar] [PubMed] [CrossRef]
71. Zhu ZH, Jia F, Ahmed W, Zhang GL, Wang H, Lin CQ, et al. Neural stem cell-derived exosome as a nano-sized carrier for BDNF delivery to a rat model of ischemic stroke. Neural Regen Res. 2023;18(2):404–9. doi:10.4103/1673-5374.346466. [Google Scholar] [PubMed] [CrossRef]
72. Gu C, Li Y, Liu J, Liu S, Long J, Zhang Q, et al. Neural stem cell-derived exosomes-loaded adhesive hydrogel controlled-release promotes cerebral angiogenesis and neurological function in ischemic stroke. Exp Neurol. 2023;370(Suppl. 1):114547. doi:10.1016/j.expneurol.2023.114547. [Google Scholar] [PubMed] [CrossRef]
73. Webb RL, Kaiser EE, Scoville SL, Thompson TA, Fatima S, Pandya C, et al. Human neural stem cell extracellular vesicles improve tissue and functional recovery in the murine thromboembolic stroke model. Transl Stroke Res. 2018;9(5):530–9. doi:10.1007/s12975-017-0599-2. [Google Scholar] [PubMed] [CrossRef]
74. Lee EJ, Choi Y, Lee HJ, Hwang DW, Lee DS. Human neural stem cell-derived extracellular vesicles protect against Parkinson’s disease pathologies. J Nanobiotechnol. 2022;20(1):198. doi:10.1186/s12951-022-01356-2. [Google Scholar] [PubMed] [CrossRef]
75. Liu Y, Huber CC, Wang H. Disrupted blood-brain barrier in 5xFAD mouse model of Alzheimer’s disease can be mimicked and repaired in vitro with neural stem cell-derived exosomes. Biochem Biophys Res Commun. 2020;525(1):192–6. [Google Scholar]
76. Apodaca LA, Baddour AAD, Garcia Jr C, Alikhani L, Giedzinski E, Ru N, et al. Human neural stem cell-derived extracellular vesicles mitigate hallmarks of Alzheimer’s disease. Alzheimers Res Ther. 2021;13(1):57. doi:10.1186/s13195-021-00791-x. [Google Scholar] [PubMed] [CrossRef]
77. Li B, Chen Y, Zhou Y, Feng X, Gu G, Han S, et al. Neural stem cell-derived exosomes promote mitochondrial biogenesis and restore abnormal protein distribution in a mouse model of Alzheimer’s disease. Neural Regen Res. 2024;19(7):1593–601. doi:10.4103/1673-5374.385839. [Google Scholar] [PubMed] [CrossRef]
78. Salewski RP, Mitchell RA, Li L, Shen C, Milekovskaia M, Nagy A, et al. Transplantation of induced pluripotent stem cell-derived neural stem cells mediate functional recovery following thoracic spinal cord injury through remyelination of axons. Stem Cells Transl Med. 2015;4(7):743–54. doi:10.5966/sctm.2014-0236. [Google Scholar] [PubMed] [CrossRef]
79. Sharp J, Frame J, Siegenthaler M, Nistor G, Keirstead HS. Human embryonic stem cell-derived oligodendrocyte progenitor cell transplants improve recovery after cervical spinal cord injury. Stem Cells. 2010;28(1):152–63. doi:10.1002/stem.245. [Google Scholar] [PubMed] [CrossRef]
80. Rong Y, Liu W, Lv C, Wang J, Luo Y, Jiang D, et al. Neural stem cell small extracellular vesicle-based delivery of 14-3-3t reduces apoptosis and neuroinflammation following traumatic spinal cord injury by enhancing autophagy by targeting Beclin-1. Aging. 2019;11(18):7723–45. doi:10.18632/aging.102283. [Google Scholar] [PubMed] [CrossRef]
81. Zhong D, Cao Y, Li CJ, Li M, Rong ZJ, Jiang L, et al. Neural stem cell-derived exosomes facilitate spinal cord functional recovery after injury by promoting angiogenesis. Exp Biol Med. 2020;245(1):54–65. doi:10.1177/1535370219895491. [Google Scholar] [PubMed] [CrossRef]
82. Adamus T, Hung CY, Yu C, Kang E, Hammad M, Flores L, et al. Glioma-targeted delivery of exosome-encapsulated antisense oligonucleotides using neural stem cells. Mol Ther Nucleic Acids. 2022;27:611–20. doi:10.1016/j.omtn.2021.12.029. [Google Scholar] [PubMed] [CrossRef]
83. Spinelli M, Natale F, Rinaudo M, Leone L, Mezzogori D, Fusco S, et al. Neural stem cell-derived exosomes revert HFD-dependent memory impairment via CREB-BDNF signalling. Int J Mol Sci. 2020;21(23):8994. doi:10.3390/ijms21238994. [Google Scholar] [PubMed] [CrossRef]
84. Li J, Gao H, Xiong Y, Wang L, Zhang H, He F, et al. Enhancing cutaneous wound healing based on human induced neural stem cell-derived exosomes. Int J Nanomed. 2022;17:5991–6006. doi:10.2147/IJN.S377502. [Google Scholar] [PubMed] [CrossRef]
85. Li WY, Zhu QB, Jin LY, Yang Y, Xu XY, Hu XY. Exosomes derived from human induced pluripotent stem cell-derived neural progenitor cells protect neuronal function under ischemic conditions. Neural Regen Res. 2021;16(10):2064–70. doi:10.4103/1673-5374.308665. [Google Scholar] [PubMed] [CrossRef]
86. Zhang L, Graf I, Kuang Y, Zheng X, Haupt M, Majid A, et al. Neural progenitor cell-derived extracellular vesicles enhance blood-brain barrier integrity by NF-κB (Nuclear Factor-κB)-dependent regulation of ABCB1 (ATP-binding cassette transporter B1) in stroke mice. Arterioscler Thromb Vasc Biol. 2021;41(3):1127–45. [Google Scholar] [PubMed]
87. Li SP, Lin ZX, Jiang XY, Yu XY. Exosomal cargo-loading and synthetic exosome-mimics as potential therapeutic tools. Acta Pharmacol Sin. 2018;39(4):542–51. doi:10.1038/aps.2017.178. [Google Scholar] [PubMed] [CrossRef]
88. Yang L, Han B, Zhang Z, Wang S, Bai Y, Zhang Y, et al. Extracellular vesicle-mediated delivery of circular RNA SCMH1 promotes functional recovery in rodent and nonhuman primate ischemic stroke models. Circulation. 2020;142(6):556–74. doi:10.1161/CIRCULATIONAHA.120.045765. [Google Scholar] [PubMed] [CrossRef]
89. Sun M, Li Q, Yu H, Cheng J, Wu N, Shi W, et al. Cryo-self-assembled silk fibroin sponge as a biodegradable platform for enzyme-responsive delivery of exosomes. Bioact Mater. 2022;8(22):505–14. doi:10.1016/j.bioactmat.2021.06.017. [Google Scholar] [PubMed] [CrossRef]
90. Zhou H, Wu Y, Li M, Liang QR, Dong W, Li Q, et al. Modular satellite nanoparticles for remedying primary and secondary injury in cerebral ischemia-reperfusion. Adv Funct Mater. 2024:2315274. [Google Scholar]
91. Ahmed W, Huang S, Chen L. Engineered exosomes derived from stem cells: a new brain-targeted strategy. Expert Opin Drug Deliv. 2024;21(1):91–110. doi:10.1080/17425247.2024.2306877. [Google Scholar] [PubMed] [CrossRef]
92. Gotoh S, Kawabori M, Fujimura M. Intranasal administration of stem cell-derived exosomes for central nervous system diseases. Neural Regen Res. 2024;19(6):1249–55. doi:10.4103/1673-5374.385875. [Google Scholar] [PubMed] [CrossRef]
Cite This Article
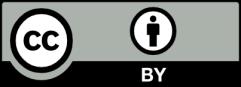
This work is licensed under a Creative Commons Attribution 4.0 International License , which permits unrestricted use, distribution, and reproduction in any medium, provided the original work is properly cited.