Open Access
REVIEW
In vitro engineered models of neurodegenerative diseases
1 Bioengineering Department, Graduate School of Natural and Applied Sciences, Ege University, Izmir, 35040, Türkiye
2 Bioengineering Department, Faculty of Engineering and Natural Science, Celal Bayar University, Manisa, 45110, Türkiye
3 Bioengineering Department, Ege University, Izmir, 35040, Türkiye
4 Department of Biomedical Technologies, Graduate School of Natural and Applied Sciences, Ege University, Izmir, 35040, Türkiye
* Corresponding Author: AYLIN ŞENDEMIR. Email:
BIOCELL 2024, 48(1), 79-96. https://doi.org/10.32604/biocell.2023.045361
Received 24 August 2023; Accepted 21 November 2023; Issue published 30 January 2024
Abstract
Neurodegeneration is a catastrophic process that develops progressive damage leading to functional and structural loss of the cells of the nervous system and is among the biggest unavoidable problems of our age. Animal models do not reflect the pathophysiology observed in humans due to distinct differences between the neural pathways, gene expression patterns, neuronal plasticity, and other disease-related mechanisms in animals and humans. Classical in vitro cell culture models are also not sufficient for pre-clinical drug testing in reflecting the complex pathophysiology of neurodegenerative diseases. Today, modern, engineered techniques are applied to develop multicellular, intricate in vitro models and to create the closest microenvironment simulating biological, biochemical, and mechanical characteristics of the in vivo degenerating tissue. In THIS review, the capabilities and shortcomings of scaffold-based and scaffold-free techniques, organoids, and microfluidic models that best reflect neurodegeneration in vitro in the biomimetic framework are discussed.Keywords
More than 600 neurodegenerative diseases (NDs) associated with atrophy affecting the central and/or peripheral zones of the nervous system have been described [1]. NDs are identified as hereditary, idiopathic, and sporadic conditions attributed to progressive nervous system dysfunction. Alzheimer’s disease (AD), Parkinson’s disease (PD), multiple sclerosis (MS), Huntington’s disease (HD), amyotrophic lateral sclerosis (ALS), epilepsy, encephalitis, and brain cancers are some of the known NDs.
Despite significant advances in defining the pathogenesis of NDs, effective treatments are not currently available. This is mainly due to the complexity of human brain physiology, the inability to clarify cause-effect relationships in the pathologies, limited access to human brain tissues, and restrictions of in vivo and in vitro models that are used in understanding the disease phenotype.
Experimental models of NDs are critical to understanding the pathophysiology better and to evaluate the potential of new therapeutic approaches. Current experimental models of NDs focus on a particular aspect of a complex pathology, or, more reductively, they are “mechanism models” that provide the closest natural replication of the disease. For this reason, no matter how successful the pre-clinical studies are similar results cannot be obtained in clinical studies [2]. All experimental models, including transgenic rodents, invertebrate animal models, and in vitro cell culture models, have distinct characteristics and limitations. Therefore, it is very important to know the targeted pathophysiology in the planned study and to choose the appropriate model.
Animal models of NDs cannot precisely recapitulate the pathophysiology of the disease in humans since NDs rarely occur spontaneously in animals, and the pathological mechanisms in animals poorly reflect those in humans [3]. Ex vivo disease models have become important tools to complement in vivo models to closely examine damage mechanisms. While organotypic slice cultures provide advantages in terms of examining cell dynamics and disease mechanisms, the chance of modifying and engineering them as much as in vitro models is low and is limited to applications allowed by tissue integrity [4]. In vitro models allow the use of human cells, unraveling the doubts due to species differences. The common in vitro models are in two different culture systems: two-dimensional (2D) and three-dimensional (3D). Classical 2D in vitro models are the most basic culture systems studied, and essential information about cellular interactions is obtained from these models. 2D models simply comprise the induction of the relevant cell type (or types) in vitro and the application of a neurotoxin to damage the cell type of interest (Fig. 1). However, these systems fall short of modeling the complex cellular, biochemical, and physical microenvironment of human tissues. Therefore, it is necessary to engineer functional 3D in vitro models that mimic the microenvironment of tissues and cell-to-cell interactions that affect pathophysiology to be examined much more realistically. These engineered models allow the co-culture of cells, enabling the study of the interaction of different cell types, as well as the extracellular elements involved in disease pathogenesis [5].
Figure 1: In vitro ND modeling basic illustration. This figure is covered by the Biorender license.
Engineered in vitro disease models can be divided into three: scaffold-free systems, scaffold-based systems, and microfluidic platforms.
Scaffold-free tissue-engineered models produce matrix from cells based on cell-to-cell interactions without requiring exogenous materials. Organoid technology, one of the leading scaffold-free techniques, has great potential in disease modeling and allows researchers with the chance to produce more complex human disease models that can precisely describe clinical pathophysiology. Organoid technology has been developed from cultures of embryoid bodies, which are 3D aggregates of self-organizing stem cells to develop different tissues in vitro [6,7]. These embryoid bodies can spontaneously differentiate to form a variety of organized tissues or can be induced by factors to differentiate into specific lineages [8]. They physically resemble the organ’s in vivo cellular organization, architecture, and composition, in whole or in part, and may also mimic sectional or complete functions of the organ [9,10]. It is thought that human-derived brain organoids may help bridge the gap between in vitro model systems and therapeutic trials with a patient-specific, personalized medicine approach [11,12].
The most important limitation in the use of scaffold-free techniques is the lack of vascularization. Although existing brain organoid systems simulate the successful formation of the brain in the embryonic period, they are far from mimicking the mature brain structure [13]. Vascularization is notably required for oxygen penetration, nutrient supply, and effective neural differentiation [14,15]. The failure of oxygen delivery causes the formation of necrotic areas in the organoid center, affecting its natural development and potential neuronal migration pathways [16]. Constructing a vascular structure that provides adequate oxygen and nutrient delivery within the organoid is necessary to overcome the limited size of the organoids [17]. From the translational medicine and commercialization point of view, another limiting factor of scaffold-free models is the high number of cells required to produce the 3D tissue [18].
To overcome the disadvantages of the scaffold-free system, scaffold-based tissue-engineered ND modeling strategies have been developed. Scaffold-based models prevent premature cell death by providing nutrients and oxygen to the cells in the core, thanks to the porous structure they form. Scaffold-based, 3D in vitro models also remain viable for a longer period due to the mechanical stability of the scaffolds [19].
Tissue engineering scaffolds can be from natural or synthetic polymers and can be produced to have very different physical and microstructural properties such as a sponge, fiber, and hydrogel [20,21]. Commercially available Matrigel, which consists of four extracellular matrix (ECM) proteins, collagen IV, laminin, entactin or heparin sulfate proteoglycan perlecan [22], is often preferred in the creation of 3D in vitro disease models in hydrogel form. However, Matrigel poses a limitation in constructing a reliable model because it varies from batch to batch. This causes heterogeneity in the models created and affects the efficacy. Synthetic hydrogels, as well as natural and synthetic polymeric scaffolds in different microstructural forms, have been used to overcome this limitation [19].
Microfluidic platforms that provide controllable microenvironments offer the possibility to incorporate more cell types, as well as a dynamic perspective to disease models. Microfluidic platforms that apply mechanical forces over the cells (flow rate, pressure, shear stress) and more adjustable ambient conditions (temperature, pH) compared to scaffold-free and scaffold-based systems have the potential to increase the reliability of in vitro ND disease models [23].
This review compiles and summarizes the current examples in different 3D in vitro models of the most common NDs and discusses the advantages and shortcomings of different approaches.
AD accounts for 75% of dementia cases worldwide and affects an estimated 35 million people. By 2050, it is predicted that 152 million people will be affected by AD and other dementias [24]. Both hereditary and environmental factors trigger the development of the disease. Current therapies for AD are only at the level of slowing the progression of the symptoms and increasing the patient’s quality of life. A reversible and curative therapeutic approach has not yet been found.
Early-onset AD is genetically inherited in an autosomal dominant manner and accounts for less than 5% of all cases. AD reflects the presence of one of 200 mutations discovered in the amyloid precursor protein (APP), presenilin 1 (PSEN1), and presenilin 2 (PSEN2) genes [25]. On the other hand, late-onset is sporadic and cannot be genetically determined [26].
The physiopathology of AD is based on extracellular amyloid-β (Aβ) plaque accumulation, hyperphosphorylated tau (p-tau) protein accumulation, intracellular neurofibrillary tangle formation, loss of synapses, neuroinflammation, oxidative stress, and neuronal cell death.
Experimental models in AD have been animal models consisting of transgenic mice expressing human genes that cause the development of Aβ plaques and neurofibrillary tangles. The development of the first transgenic AD mouse model with Aβ units in 1995 paved the way for over 200 transgenic rodent lines. However, approximately 80% of the pre-clinical tests completed and deemed successful with these transgenic animal models failed in clinical trials [27]. These results showed that animal models simulated pathological events like Aβ accumulation, only mimicking early onset AD, but they did not completely carry the salient physiological features, such as neuronal loss and neurofibrillary tangle development [28].
Toxins like scopolamine, streptozotocin, colchicine, and heavy metals have been used in in vivo dementia models. However, these toxin-induced models do not reflect human pathophysiology as they cause local brain damage, lack disease progression, and induce high mortality. Nevertheless, toxin-induced models have been frequently used as they are the most realistic models so far, reflecting cognitive dysfunctions and dementia [29]. But in vitro modeling of dementia is much more difficult. The necessity of reflecting the cellular pathologies (inflammation, gliosis, autoimmunity, complement factors) occurring in AD has always been a limiting factor, apart from inducing AD. The most common toxins used to reflect reactive oxygen species (ROS), NADPH oxidase (NOX), inducible nitric oxide synthase (NOS), and neurotoxins induced by Aβ aggregates in vitro are Aβ monomers and oligomers [30–33].
Models created by neurons derived from immortalized cell lines (e.g., SHSY-5Y; human-derived neuroblastoma cell line) do not provide reliable results, as they show a quite different amyloidogenesis phenotype from degenerated neurons in AD [34]. 1–5 μM Aβ concentrations are required to cause cell death in vitro, while it is known that this concentration is 50 nM in natural brain tissue [35]. In studies with PC-12 cells, Aβ1–42 treatment increased the phosphorylation of tau at 10 µM, but not at lower concentrations [36,37]. In another study, 25 μM Aβ1–42 administration on PC-12 cells increased cell apoptosis [38].
3D in vitro models can mimic the physiology more realistically, as the 3D configuration of neurons affects cytoskeletal dynamics [5]. Similar to AD pathophysiology, there was glutamatergic rather than GABAergic toxicity in human embryonic stem cell (hESC)-derived neurons exposed to oligomeric pre-fibrillar Aβ forms. In this study, time-dependent Aβ accumulations and subsequent cell death were reported in Matrigel-coated ultra-low attachment plates [34]. Induced pluripotent stem cell (iPSC) based models enabled the discovery of some basic differences between healthy control groups and individuals with AD. Researchers have characterized several iPSC lines from donor cells from familial AD and sporadic AD patients. Studies on these cells have revealed differences such as increased Aβ, specifically amyloid β42 (Aβ42) and tau hyperphosphorylation compared to those in iPSCs derived from healthy controls. iPSC lines also reflected additional AD-related pathologies such as increased glycogen synthase kinase-3 beta (GSK3β) activation, increased large endosome number, and accumulation of intraneuronal Aβ oligomers [39].
PSEN1 and PSEN2 mutations are genetic factors that cause early-onset familial AD. In a study conducted by differentiating iPSCs produced from AD patient-derived fibroblasts with these mutations into neurons on gelatin-coated plates, the ratio of Aβ40 and Aβ42 synthesized by these neurons was higher than healthy controls in embryoid bodies [40]. This shows that the model reflects the familial AD pathophysiology.
Since it is impossible to obtain primary human neuronal cells from healthy (or diseased) donors, attention has been drawn to stem-cell-induced neurons for more realistic models. In a model with iPSCs obtained from blood cells of AD patients, 3D neuronal spheroids were created in 100 mm ultra-low-attachment plastic plates by inducing iPSC colonies with ROCK inhibitor and growth factors (epidermal growth factor (EGF), fibroblast growth factor-2 (FGF2), brain-derived neurotrophic factor (BDNF), neurotrophin-3 (NT3)). Researchers compared 3D spheroids with 2D culture and found that while there were differences in Aβ clearance between individual iPSCs in 2D, these differences were less in 3D [5]. Another research group using iPSCs derived from AD patients also generated brain organoids showing AD-like pathophysiologies such as hyperphosphorylated tau protein, Aβ aggregation, and endosome aberration. These pathologies were observed in a time-dependent manner harboring APP duplication or PSEN1 mutation [41]. This was a result revealing the similarity of the model to in vivo. In a similar study with the differentiation of iPSCs into neurons, it was reported that p21-activated kinase-mediated sensing of Aβ oligomers was only in the 3D model created in Puramatrix and F-actin-related proteins were clearly associated with disease processes in 3D [42].
Another in vitro AD model is produced by differentiating iPSCs derived from AD patients with the PSEN1 ΔE9 mutation into astrocytes. They demonstrated the pathological features of AD, such as increased Aβ production, altered cytokine release, and impaired Ca2+ homeostasis. These pathologies caused oxidative stress in astrocytes and suppressed neuronal support by reducing lactate production. This study also shows that PSEN1 mutant astrocytes display a serious disease phenotype and may contribute to AD progression [43].
Scaffolds that allow for greater physiological interactions in three dimensions between neurons and glia have also been used in AD models. Microenvironments that mimic the ECM, the presence of biochemical factors, and functional and structural molecules play a direct role in in vitro disease models.
In their study, Ranjan et al. formed a porous poly (lactic-co-glycolic acid) scaffold using a wet electrospinning technique and cultured familial AD-derived iPSCs on these scaffolds. Increased neuronal differentiation and spontaneous enhanced pathogenic Aβ42 and p-tau levels were observed in neurons carrying familial AD mutations, compared to age-matched healthy controls on scaffolds within seven days [44].
Cui et al. investigated the effect of self-assembled peptide hydrogel containing the YIGSR (Tyr-Ile-Gly-Ser-Arg) functional domain on the survival and neuronal differentiation of neural stem cells (NSCs) in in vitro and in vivo AD models. To examine the neuroprotective effect, 40 µM Aβ1–40 was applied to the cells simultaneously with the peptides. The researchers found that self-assembled peptides not only increased cell survival, but also reduced the number of cells undergoing apoptosis. In addition, neuronal differentiation was increased on these peptides when compared to self-assembled peptides without YIGSR. In rat AD models, NSCs with self-assembled peptide containing YIGSR increased cognitive rescue and decreased the number of cells proceeding to apoptosis and the Aβ production [45].
The development of cells in an organoid can also be achieved by using scaffolds. In the study of Choie et al., a 3D organoid was developed with human neural stem cells (hNSC) in Matrigel scaffolds, and extracellular Aβ aggregation and accumulation of tau in the dystrophic neurites and cell soma was observed in vitro for the first-time [46]. AD virus models were also used to understand disease pathophysiology. A 3D AD model was created in a study with Herpes simplex virus type I (HSV-1), which has recently been shown to play a role in AD development pathology. Silk sponge scaffolds with 500 to 600 µm pores were prepared as 6 mm discs, and 2 mm holes were drilled in the center to form annular scaffolds. After the scaffolds were coated with laminin (0.5 mg/mL), iPSC-derived hNSCs were seeded onto the scaffolds, and the next day, type I rat tail collagen gel was added. Organoids cultured for 4 weeks were infected by HSV-1, and Aβ plaque-like formations, gliosis, and neuroinflammation were reported [47].
Basic pathologies of AD were provided in a model tri-culture of neurons, astrocytes, and microglia on a 3D co-centric two-chamber microfluidic platform. High levels of Aβ-producing human neural progenitor cells (hNPCs) with FAD mutations and astrocyte-differentiated cells were cultivated in the central chamber, while microglia were cultivated in the outer angular chamber. The central and angular chambers were connected by migration channels. Using this platform, migration of microglial cells has been observed. Aβ aggregation, p-tau deposition, axonal cleavage, and neuroinflammatory activity such as NO, CCL2, IL8, TNF-α, and IFN-γ release were demonstrated [48]. This model, which is useful in elucidating neuro-glial interactions, can be used not only for AD but also for understanding other disease pathologies such as PD and MS.
Due to the experimental challenges of neurospheroid cultures and the fact that they are affected by variability, including size differences, variable cell culture procedures, including cell density and media composition, the researchers further developed uniformly sized neurospheroids. These neurospheroids were derived from NSCs with FAD mutations and human-derived iPSCs (hiPSCs) with a PDMS microarray platform produced by photolithography for high-throughput drug screening [49]. In the developed platform, dendrite formations were observed in neurospheroids containing mature neurons. After 8 weeks of culturing, these neurospheroids were reported to possess Aβ plaques and p-tau deposits [49].
The disruption of blood-brain barrier (BBB) in AD has been shown to precede disease pathology. In this regard, to investigate the role of BBB in AD, a three-channel microfluidic system was developed. Researchers used NSCs with FAD mutations in AD modeling and hCEMC/D3 cells (brain endothelial cell line) in BBB modeling. To modulate the differentiation periods of these two cells separately, sequential seeding was performed on the developed chip, and the intermediate channel was left filled with air until co-culture was initiated. Human NSCs were cultivated in Matrigel, and hCEMC/D3 cells were cultivated in collagen-filled channels. After the endothelial barrier was formed, the intermediate channel was filled with collagen type 1, and the interaction between differentiated NSCs, and the endothelial layer was ensured by diffusion of soluble factors. It was stated that this model showed pathologies such as increased BBB permeability, decreased endothelial junction markers (claudin-1, claudin-5, zonula occludens-1, occludin, VE-cadherin), increased expression of matrix-metalloproteinase-2 and ROS, and accumulation of Aβ peptides in the vascular endothelium [50].
The researchers investigated the effects of Aβ on accumulations of microglial cells in a microfluidic chemotaxis platform by subjecting the cells to different Aβ42 gradients and surface bound Aβ concentrations. Microglia chemotaxis for Aβ monomers and oligomers was determined to be most effective at two different Aβ42 concentrations (23 pg/mL and 23 ng/mL), and at lower concentrations of Aβ42, microglial migration was further enhanced by monocyte chemoattractant protein-1 (MCP-1) secreted by the microglia [51]. This study created a model to elucidate the microglial pathology in AD.
PD is the second most common ND after AD. The disease is characterized by the destruction of dopaminergic (DA) neurons in the substantia nigra (SN), particularly affecting the ventral component of the pars compacta [52].
In 1817, British physician James Parkinson reported his observations of six people as “shaking palsy.” Later, the studies on the understanding of PD with its modern name were made by Jean-Martin Charcot. In 1912, German neurologist Friedrich Lewy defined the cardinal neuropathological lesion that later bears his name: Lewy body (LB) [53].
LBs are cytoplasmic inclusions comprising several neurofilament proteins, together with the proteolytic proteins found in the remaining DA neurons. LBs are filamentous in nature and contain predominantly alpha-synuclein (α-Syn) and ubiquitin proteins. Although LBs are also found in other diseases, they are thought to be the pathological hallmark of PD [54]. Age is the primary risk factor for PD. The incidence of PD is 1%–2% between the ages of 50–65 and around 3.5% between the ages of 85–89 [55].
Although genetic mutations causing PD have not been identified, it is known that there are defects in α-Syn, parkin (PARK2), and ubiquitin C-terminal hydrolase-L1 (UCHL-1) genes, which play a role in the formation of LB protein aggregates characterized in neuropathology.
Various in vitro culture procedures have been developed to model the pathogenesis of PH. The most commonly used cell lines in PD studies are the PC12 cell line derived from rat pheochromocytoma and human-derived SH-SY5Y neuroblastoma cells with ion channels and neurotransmitter receptors that secrete catecholamines such as dopamine and can exhibit DA neuron-like properties [56,57]. Since these cells can be obtained more easily than primary cells and can maintain their structural stability, ensuing genetic manipulations, they provide great advantages in the production of toxicity-induced PD models and the use of these models in pharmaceutical trials.
Neurodegeneration, oxidative stress, loss of function of mitochondria, and formation of protein aggregates are among the known causes of PD. Models created by inducing PD by triggering the conversion of ROS by neurotoxins such as 6-hydroxydopamine (6-OHDA), 1-methyl 4-phenyl 1,2,3,6-tetrahydro-pyridine (MPTP), paraquat and rotenone have led to important discoveries in understanding the molecular pathways that occur during the degeneration and death of DA neurons [58]. One of the catecholaminergic neurotoxins, 6-OHDA, and its noradrenergic analog have been used for more than 30 years in the creation of in vitro and in vivo experimental models for PD studies. Since 6-OHDA has a chemical structure similar to dopamine and norepinephrine, it is recognized with high affinity by the dopamine transporters (DATs) and norepinephrine transporters (NETs) in the plasma membrane. Thus, it causes oxidative stress on the catecholaminergic pathways of the peripheral nervous system (PNS) and central nervous system (CNS) through ROS and hydroxyl radicals and causes degeneration of DA and noradrenergic neurons [59].
A significant change in PC-12 morphology and DNA and chromosome breaks in cells was observed with the apoptosis-triggering effect of 6-OHDA [60]. Similarly, 6-OHDA has been tested with neuroblastoma, cerebellar granule, and primary mesencephalic DA cell lines, which are frequently used as model cell lines in the literature, and the data obtained support that 6-OHDA activates oxidative stress and apoptotic pathways in 2D culture [61,62].
MPTP, unlike 6-OHDA, can quickly pass through the BBB and be metabolized to its active form. 1-methyl-4-phenylpyridinium (MPP+), which is stored in neurons via the DAT, and as a result, it plays a role in the pathophysiology of mitochondrial dysfunction by affecting the mitochondrial electron transport chain [63].
Many researchers have generated iPSCs derived from PD patients to obtain cells with susceptibility alleles in PD-related genes such as leucine-rich repeat kinase 2 (LRRK2), glucocerebrosidase (GBA), PARKIN, α-Syn, synaptophysin, and phosphatase and tensin homolog-(PTEN)-induced gene (PINK1) [48].
Developing an idiopathic PD model for the first time with organoids generated from iPSCs, the researchers were able to demonstrate a complex network of DA neurons. They observed statistical differences in expression levels of the LIM homeobox transcription factor alpha and tyrosine hydroxylase genes between organoids developed from healthy and PD cells [64]. In a study with iPSCs developed from a patient with a mutation in the LRRK2 gene, 3D neuro-ectodermal and intestinal organoids were created to examine the brain-gut relationship dependent on LRRK2. As a result of the differences seen in the gene expression profiles of these organoids, the researchers stated that PD may affect intestinal functions [65].
In a study aiming to standardize reproducible organoid production, human neuronal progenitor cells (NPCs) were cultured for 8 days in 96-well ultra-low adhesion plates until they formed colonies. On day 8, the colonies were embedded in geltrex (a gel containing ECM proteins such as laminin, fibronectin, collagen, entactin, and heparin sulfate proteoglycans) droplets, and maturation was initiated by differentiation media in 24-well ultra-low adhesion plates under shaking media for 30 days. This protocol developed standardized organoids that retained the basic aspects of midbrain development, such as DA neuron and astrocyte differentiation and compartmentalization, and can be utilized in PD models [66].
In a PD model developed using 3D bioprinting, DA neurons were cultured in ultra-short self-assembled tetrapeptide Ac-Ile-Val-Phe-Lys-NH2 (IVFK) and Ac-Ile-Val-Cha-Lys-NH2 (IVZK) scaffolds. DA neurons encapsulated in tetrapeptide gels responded to 6-OHDA. They recorded spontaneous electrophysiological activity of encapsulated DA neurons by multi-electrode arrays. The model supported vascularization (by human umbilical vein endothelial cells (HUVECs)), and neuronal culture was continued for more than one month [67].
In another study, PD was modeled by culturing SH-SY5Y and U-87MG glioblastoma cell lines on polyacrylonitrile (PAN) and Jefamine® doped polyacrylonitrile (PJ) electrospun scaffolds. PAN and PJ nanofibrous scaffolds promoted proliferation, differentiation, and 3D interaction of DA neuron and astrocyte-like cells and increased neuronal survival against PD mimetic toxin treatments, including rotenone, MPTP, MG132 proteasome inhibitor and buthionine sulphoximine (BSO) [68].
Researchers developed a different approach using iPSC, genetic, and scaffold technologies together in a model. Human iPSC-induced DA neurons derived from healthy people and PD patients harboring LRRK2/GBA mutations were cultured on 3D silk scaffolds produced by the melt-molding technique [69]. Sodium chloride particles with a diameter of 415–500 μm were added to the silk solution and removed by washing, creating pores on the silk scaffolds. The 3D platform supported DA neuronal network formation, whereas α-Syn and purine metabolite profiles showed changes in PD-derived cells, offering a realistic approach to PD pathophysiology [69].
There are several microfluidic models for PD. One of them is the model in which primary cortical neurons were cultivated on a microfluidic platform in the presence of recombinant α-Syn fibrils. They reported that neurons were able to internalize the α-Syn fibrils and transported them along axons resembling the characteristic spreading pattern of LBs [70].
Differentiating neuroepithelial cells derived from iPSCs into DA neurons within phase-guided microtiter plate format bioreactors brought a distinct perspective to PD modeling. Cells differentiated to functional DA neurons in a Matrigel-loaded microfluidic bioreactor with differentiation media in 30 days, and 19% showed high tyrosine hydroxylase levels [71].
In another study using the same type of microfluidic reactor, patient-specific PD-derived iPSCs carrying the LRRK2-G2019S mutation were differentiated into DA neurons. The DA differentiation, neurite length, and branching complexity in LRRK2-G2019S neurons decreased, and cell death increased due to changing mitochondrial morphology. DA phenotypes were found to be improved with the administration of an LRRK2 inhibitor [72].
The differentiation of PD-derived human neuroepithelial stem cells into DA neurons was performed on an automated cell culture platform integrating the microtiter plates with a robotic system for long-term monitoring and maintenance of the culture system. This system, called Pelican, has the potential to automate patient-specific disease modeling, enabling a versatile set of in vitro analyses [73].
MS is an inflammatory ND of the CNS with myelin destruction and is one of the most important causes of disability in young adults. It affects the quality of life of approximately 2.1 million individuals worldwide. The first clinical and pathological description of the disease was made by Charcot in 1868. Its prevalence varies between 2–200 per 100,000, depending on geographical features. It is more common in the 18–50 age range and in women. There are three phenotypes: clinically isolated syndrome, which is the first neurological picture associated with MS, a relapsing-remitting form with attacks and remissions, and a progressive form with gradually increasing disability [74,75]. MS is diagnosed by clinical findings meeting the McDonald criteria based on the clinical lesions and the presence of demyelinating plaques in brain magnetic resonance imaging (MRI) in regions typical for MS (periventricular, juxtacortical/cortical, infratentorial, and spinal) [76].
MS pathology is characterized by demyelinating areas called plaques or lesions that indicate the destruction of myelin sheaths and loss of myelin-forming cells (oligodendrocytes) in the white and gray matter of the CNS [77]. Although axons and neurons are mostly spared in early MS, neuroaxonal loss occurs as the disease progresses. The result is CNS atrophy and the production of multiple sclerotic glial scars in the lesions. Demyelinating plaques in white matter can be partially remyelinated [77–79].
Contrary to the accepted views that the CNS has a limited capacity for regeneration, a partial regenerative response (remyelination) is seen with the formation of new myelin sheaths following the damage to oligodendrocytes [80]. However, for many patients, remyelination eventually fails and the metabolic support that myelin sheaths normally provide to axons is lost, accompanied with axonal degeneration, and progressive degeneration that characterizes the later stage of MS progresses.
Experimental autoimmune encephalomyelitis (EAE) is generally considered a disease model for MS. However, this is insufficient—although EAE mimics an acute MS lesion, acute and chronic inflammation are lacking for modeling the neurodegeneration that characterizes progressive MS [81]. Disease models that reflect MS pathology require other mechanism patterns that are usually created using toxins that damage oligodendrocytes (thus leading to primary demyelination) or other cell types to varying degrees.
Animal studies have tended to the understanding of MS; however, they partially model the underlying pathogenic mechanisms [81]. Most of the commonly used CNS in vitro culture systems are of animal origin. At this point, in vitro models using human cells are more realistic since there are fundamental differences between the rodent and the human brains [82].
One of the biggest obstacles to in vitro myelination studies is understanding how two different cell types communicate effectively to perform a specific task. Myelination occurs as a result of neuro-glial interaction and provides efficient and rapid conduction of action potentials throughout the CNS. During CNS development, inductive and/or inhibitory signals originate and propagate with neuro-glial interaction that induces myelination. Mitogenic and trophic factors produced by neurons also modulate the proliferation and migration of oligodendrocyte precursor cells (OPCs). This multifactorial interaction resulting in myelination is difficult to study using in vitro methods [83,84].
Different toxins or immune-mediated inducers are frequently used in demyelination studies. One of the toxin-based demyelination models is the use of lysolecithin (LPC), which disrupts cell membranes, including myelin, by acting on lipid bilayers to form micelles. It has been shown to damage directly the myelin layer in vitro [85–88].
Lipopolysaccharide (LPS), which induces innate immune cells, is also used in modeling MS pathology [89–92]. Researchers investigating the effect of dimethyl fumarate (DMF) used in the treatment of relapsing-remitting MS in vitro used the oli-neu (primary mouse oligodendrocyte) cell line. By applying LPS-mediated inflammation to cells, researchers demonstrated that DMF induces differentiation of oli-neu cells into mature and potentially myelinating cells, and it has anti-inflammatory and antioxidant effects [93].
The cuprizone demyelination model is of particular interest among toxic models [94]. Cuprizone [bis (cyclohexanone) oxaldihydrazone] is a copper chelator. After cuprizone administration, cytochrome oxidase and monoamine oxidase are inhibited in mature oligodendrocytes, resulting in apoptosis due to mitochondrial damage. Cerebral microglia activation is observed in vivo as a result of the demyelination of axons and the production of tumor necrosis factor-alpha (TNFα) and interferon-gamma (IFNγ) by oligodendrocytes is increased [95–97].
In vitro systems that culture OPCs with CNS or PNS neurons are relatively simple, inexpensive, high-throughput models [98]. Oligodendrocytes express genes encoding cell-specific myelin structural proteins [99–101]. Isolated rat and mouse primary oligodendrocytes were shown to express most of the proteins and lipids associated with myelin membranes, but primary cultures of human oligodendrocytes are difficult to study due to the difficulty of reaching live human brain tissue. For this reason, immortalized human oligodendrocyte cell lines are preferred [82]. The human oligodendrocyte cell line (HOG) is a cell line created from a surgically removed oligodendroglioma and has been shown to express myelin basic protein (MBP) and some oligodendrocyte-specific proteins such as A2B5 [102]. It is frequently used in myelination and remyelination studies [103–105].
Myelinated brain organoids have recently turned into a new and important model system for myelination. Human cortical organoids contain GABAergic interneurons, a small number of oligodendrocytes, and OPCs located in the ventral forebrain, mimicking the dorsal forebrain composition [106]. A single-cell sequencing study reported that oligodendrocytes in ventral organoids go through developmental stages similar to those observed in the developing human brain [107]. During ventral organoid differentiation, with the addition of growth factors such as platelet-derived growth factor (PDGF-AA), Insulin-like growth factor 1 (IGF1), and NT3, the number of OPCs increases for about 2 months [108].
Neurons derived from iPSCs can be co-cultivated with iPSC-derived or primary OPCs and oligodendrocytes. Mixed culture approaches like this could enable the investigation of interactions between oligodendroglia and neurons. A group working with hiPSCs studied oligodendrocyte developmental stages by developing human oligodendrocyte spheroids in microwells. The researchers reported that cell morphologies change as they mature and begin to myelinate neurons by 100 days. They also showed that LPC causes oligodendrocyte loss, a process that can be monitored with live imaging [107].
Another study on hiPSCs reproducibly obtained oligodendrocytes and myelin in ‘oligocortical spheroids’ using MSC-derived extracellular vesicles (EVs). Myelin-related protein expressions were reached at week 20, and myelin structures were observed at week 30 [109].
Chesnut et al. developed another iPSC-derived brain organoid that can be used to study myelin damage. They examined the damage by applying multiple toxins to examine the myelination disruption on the brain organoid containing highly myelinated axons they developed in eight weeks in non-coated plates under constant shaking at 88 rpm. Researchers who tried cuprizone, bisphenol A, tris(1,3-dichloro-2-propyl) phosphate, and ibuprofen determined that myelination was affected only in cuprizone application [110]. All spheroid studies revealed that co-culture conditions and interactions of oligodendrocytes with neurons and/or astrocytes are important for mature myelination in in vitro scaffold-free culture conditions.
Studies to investigate the need for axonal signals to initiate oligodendrocyte myelination have shown that myelination does not depend solely on co-culture with neurons, but oligodendrocytes can also form myelin on surfaces such as paraformaldehyde-fixed axons [111].
The development of scaffolds that mimic axons has prompted the investigation of their effects on oligodendrocytes. With this approach, OPCs or oligodendrocytes are studied by culturing on nanofibers or microcapillary arrays. Such synthetic approaches are important not only to screen drugs for multiple sclerosis therapeutics but also to screen for oligodendrocyte association of all NDs.
Appropriate biomaterials that mimic the structure of axons and the three-dimensional microenvironment in the brain with their fibrous microarchitecture can also support cell migration, attachment, and survival, and increase the success of ND models as well as therapeutic studies. Electrospinning is an advantageous method for the reproducible and modifiable production of the physical properties of the extracellular matrix (e.g., pore and fiber size), chemical properties (e.g., cell adhesion proteins), and mechanical properties (e.g., Elasticity). In the literature, beneficial effects of electrospun fiber scaffolds on neural cell proliferation, viability, differentiation, and neurite formation have been demonstrated [112–114]. Furthermore, the functionalization of electrospun scaffolds with ECM components can control cell attachment and neurite outgrowth.
Lee et al. reported that the use of nanofibers offers occasions to study myelination mechanisms and is a complementary approach to induce myelination without requiring the presence of co-cultured neurons. Nanofiber scaffolds facilitate the investigation of oligodendrocyte dynamics and enable the testing of therapeutic agents without confounding neuronal effects. As demonstrated in the study, OPCs and oligodendrocytes are sensitive to the minimum fiber diameter threshold of 0.4 µm to initiate myelination-related processes (Fig. 2) [83].
Figure 2: Myelin wrapping of oligodendrocytes around electrospun fibers. This figure is covered by the Biorender license.
A research group has also developed synthetic mimics of biological axons that represent key geometric and mechanical characteristics by 3D printing of polydimethylsiloxane (PDMS), poly (2-hydroxyethyl methacrylate) (poly-HEMA) and polyhexanediol diacrylate-co-star-polyethylene glycol (poly (HDDA-co-star PEG)) by projection micro-stereolithography. The fiber surfaces were coated with laminin and poly-D-lysine. These artificial axons reflected the mechanical cues presented by neurons in vivo with similar stiffness to axons, enabling the study of myelination. Researchers reported that laminin-coated pillars were more ensheathed by oligodendrocytes compared to poly-D-lysine ones. For ~16 μm diameter laminin-coated pillars, the artificial axon with a stiffness of 140 kPa showed approximately three times more oligodendrocyte interaction compared to those with a stiffness of 0.4 kPa. Also, more oligodendrocyte interactions were observed in 10 µm axons compared to 20 µm diameter axons [115].
In addition to fibrous microstructures, myelination, and demyelination modeling studies are also carried out in hydrogels that allow cells to move in 3D. It was reported that myelination increased in Schwann cells co-cultivated with dorsal root ganglion (DRG) cells in gelatin meth acryloyl (GelMA) hydrogels with Young’s modulus of 32.6 ± 1.9 kPa and mean pore size of 10.3 μm. Schwann cells had a higher rate of myelination on GelMA compared to poly-D-lysine and collagen-coated surfaces [116].
Co-culture systems using rat DRG/Schwann cells are widely used to fundamentally study myelination in vitro. Researchers developing co-culture using polyvinylidene fluoride-trifluoro ethylene (PVDF-TrFE) scaffolds showed that aligned fibers-oriented Schwann cells and neurites and supported longer neurite extension of the DRG compared to cultures without scaffolds and monoculture. In addition, they reported that myelin structures formed around neurites in scaffolds and emphasized the importance of oriented fibers in myelination studies [117].
In another study, OPCs cultured on collagen microspheres differentiated into oligodendrocytes and formed myelin sheath in co-culture with DRG neurons [118]. This study shows that microspheres produced from biocompatible materials can also be used in myelination and neurite modeling studies and provide a supportive environment for cell growth.
Scaffold-based myelination models provide substantial evidence of the importance of mechanical as well as biochemical regulation of myelination.
Microfluidic devices are advantageous platforms for co-culturing neurons and oligodendrocytes in in vitro myelination models. Spontaneous formation and random orientation of myelin sheaths have made the analysis of myelination difficult in systems reported to be used in scaffold-based and scaffold-free myelination models. In organ-on-chip models, myelin formations can be examined by channeling the chip. In a study performed on the 3D-printed microchip, oligodendrocytes co-cultivated with DRG neurons interacted with neurites extending into an independent channel from the somas. Myelination capacity was demonstrated by overlapping MBP- and βIII-tubulin-positive staining and MBP segments were reported to be located along neurites. The myelin segment distribution followed the neurite network distribution, and the differences in myelin appearance were evident when compared with the classical culture [119].
Sharma et al. developed a microphysiological biomimetic chip that demonstrated neurite outgrowth (0.4–0.55 µm), myelination of Schwann cells (4.7%), and assessment of nerve conduction velocity (0.13–0.28 m/s) [120]. They created hydrogel scaffolds using micro-photolithography. Cell adhesion restrictive surfaces were created using 30% w/v polyethylene glycol dimethacrylate 1000 and 1.1 mM lithium phenyl-2,4,6-trimethylbenzoylphosphinate solution. After the formed channels were filled with Matrigel, motor neurons and Schwann cell co-cultured spheroids were transferred into the chip. For 4 weeks, the elongation of neurites along the canal and myelin formation of Schwann cells were monitored and evaluated [120].
Researchers who developed another 3D neuron culture platform on a microfluidic chip (Neuro-IMPACT) modeled neuronal network, BBB, and myelination processes in this high-throughput scanning platform [121]. The developed chip enabled 3D neuron culture for a long time and enabled the examination of neuro-endothelial and neuro-glial interactions on the same platform. The functionality of the model was demonstrated by BBB permeability testing, imaging of myelin sheaths, and observing neuronal functionality with intracellular calcium changes.
Table 1 gives a summary of the selected studied in vitro models of ND.
Other Neurodegenerative Diseases
Adult-onset HD, which mostly affects striatal and cortical neurons in the brain, is characterized by motor abnormalities, cognitive decline, and psychiatric symptoms, with an incidence of 7–10 per 100,000 [122]. HD is an ND characterized by the increase of cytosine-adenine-guanine (CAG) trinucleotide repeats in the gene encoding the Huntington protein (HTT) and forming a polyglutamine (PolyQ) extension. HTT is a protein involved in the removal of protein misfolding and clearance of abnormal gene expressions, and directly affects neuronal apoptosis and cytoskeletal elements. The role of HTT in the pathogenesis of HD has also been used as a tool for in vitro experiments, and HTT accumulation was induced in vitro as a result of transfection of cell lines (SH SY5Y, PC12, Neuro2a) with vectors containing poly (CAG) such as HTT exon-1 protein [123–125].
As in other NDs, many iPSC-based HD models have also been studied [126–128]. In the first study to report iPSCs derived from HD patients, cells reflecting the underlying disease pathologies, such as increased lysosomal activity, CAG stability, and increased lysosomal activity were obtained [129].
On-organ-chip approaches have also been developed for modeling HD. Researchers modeling presynaptic, synaptic, and postsynaptic areas with HD-mutant mouse cells in 3 separate regions of a chip examined intracellular events. Having developed a platform that models the pathophysiology of HD, researchers have observed cellular and network dysfunctions [130]. Presynaptic and postsynaptic pathways were examined in another HD on-chip study, which was developed to examine drug interaction with the pathogenic mechanism, and pridopidine was found to maintain synaptic transmission and synapse homeostasis [131].
Tissue engineering scaffold usage is still limited in developing HD models, but they are used to develop therapeutics in HD [132,133].
Prion Disease is similar to AD and PD in terms of its pathology related to misfolding of another protein, cellular prion-related protein (PrPC). Although 2D in vitro models have failed to represent the disease mechanism, 3D spheroid models based on murine NSCs (Collins & Haigh, 2017) [134] and hiPSCs [135] showed more promising results for simulating the accumulation of prions. Also, the advantage of iPSCs was the presentation of the genetic mutations related to the disease, as well as the simulation of astrogliosis, which is a hallmark of several NDs.
Different than other NDs, ALS affects mainly motor neurons and progresses faster. The majority of the in vitro models used for ALS are based on the genetic mechanisms of the disease, although only a minority (5%–10%) of ALS cases are familial [136]. Motor neurons derived from iPSC of ALS patients were used to model the disease [137]. These models showed increased misfolded superoxide dismutase 1 (SOD1), accepted as a distinct marker of ALS [138]. However, SOD1 expression is much higher in mouse iPSC models compared to human iPSCs, raising concern about the validity of human models [139]. The fused-in sarcoma (FUS) gene, Tar DNA binding protein (TDP-43), and chromosome 9 open reading frame 72 (C9ORF72) are also other common markers used in iPSC derived ALS models [137].
Neuromuscular junctions (NMJs) are also affected in several neurodegenerative and neuroimmunological disorders like ALS and Myasthenia gravis. In vitro models of NMJs have been recently established using microfluidic platforms and bioreactors [140,141]. Two chambered microfluidic platforms were used to culture motor neurons that can extend their axons through microchannels to reach and innervate muscle cells [142,143]. It is also possible to incorporate electrical stimulation to these compartmentalized systems [144].
Microfabrication techniques allow the functionalization of surfaces to achieve better attachment of neurons and myocytes. A PDMS platform that utilized micro-cantilevers was used for the co-culture of motor neurons and myocytes and allowed to measure muscle contraction force due to stimulation. This platform is accepted as the first tissue-engineered model of the NMJ [145]. In a spheroid model of NMJ, skeletal muscle cells were co-cultured with iPSC-derived motor neurons [146]. In an on-chip system using optogenetic technology, iPSC cells were differentiated into motor neurons, and a motor unit model was created by co-culture of skeletal myocytes to facilitate the examination of ALS pathogenesis [147]. The same group enhanced their microfluidic platform by also incorporating the BBB into the same device [148].
Since neuroinflammation is a major hallmark of NDs, in vitro systems that study microglia are also of crucial interest. There are various murine and human microglia cell lines, as well as in vitro models for culturing primary microglia or differentiating microglia from stem cells; however, 2D cultures have failed to mimic the necessary microenvironment for the presentation of the correct phenotypes [149,150]. Successful integration of iPSC-derived microglia in brain organoids [151], electrospun poly-caprolactone nanofibers [152], collagen [153] and RADA-16 peptide hydrogels [154] were shown to possess more success in representing neuroinflammation in 3D. The existence of ECM proteins in the scaffolds provide a more in vivo-like microglial morphology [153]. Functionalization of PCL fibers with graphene also contributed to a similar, ramified, non-phagocytic morphology closer to the in vivo resting state of the glia [155].
There is increasing evidence that gut microbiota plays an important role in NDs pathophysiologies (microbiota-gut-brain axis (MGBA) [156]. Microfluidic chips would provide an excellent platform for in vitro simulation of MGBA, since they furnish the possibility to incorporate different interconnected channels that can be designed to culture various microbiota, as well as the different cell types of the CNS simultaneously on a single device, and to study their one-to-one and/or collective interactions. A recent European Research Council (ERC) funded project (MINERVA, Grant no. 724734) aims to engineer platforms to study the effects of MGBA on AD. Studies have reported intestinal inflammation in chip models [157]. Although challenging, MGBA-on-chip models would provide a significant impact on our understanding of the complex relations between our gut microbiota, our immune system, and the NDs.
Although two-dimensional in vitro models reflect some pathophysiological conditions, they are insufficient because they cannot fully reflect the complex brain physiology. To fill this gap between conventional 2D in vitro models and clinical applications, 3D-engineered in vitro models form a bridge (Table 2).
NDs are a serious health problem characterized by progressive loss of cells in the brain. The mechanisms that cause NDs are still being identified. In vitro ND models are vital to understanding the mechanisms of these diseases, developing early detection methods, and finding effective treatments.
With the 3D-engineered models discussed in this review, it is possible to characterize the mechanisms of occurrence of NDs and enable the discovery of new treatment strategies. Particularly, ND-on-chip models offer the opportunity for high-throughput screening of potential therapeutic compounds. Researchers can assess the efficacy and safety of a large number of drug candidates, leading to faster and more efficient drug discovery processes. However, it should be noted that these models are still incomplete.
The development of better 3D in vitro models based on cells from the patient will be able to reflect the genetic and epigenetic background that contributes to the pathology of NDs. In addition, the development of precision medicine treatments will accelerate with these personalized models. A major drawback of iPSCs in modeling NDs is the intrinsic rejuvenation of somatic cells during the reprogramming stage and subsequent failure in replicating the aging effects closely associated with most NDs.
One of the biggest challenges in the progress and testing of drugs targeting the CNS is the BBB. Therefore, complex in vitro models should include fluidic systems capable of mimicking the BBB. Recent studies include vascularization, considering this obstacle. In addition, it is necessary to develop 3D in vitro models in which immune system cells are included to examine the role of neuro-immune relations on neurodegeneration in detail.
Continued advancements in bioengineering techniques, such as microfluidics, bioprinting, and organ-on-a-chip technologies, are enhancing the capabilities of 3D-engineered ND models. Employment of these technologies in an orchestrated, hybrid approach enables the creation of more complex and interconnected multicellular networks, better control of cellular microenvironments, inclusion of the biochemical and mechanical extracellular matrix cues, and the integration of vascular and immune components, further enhancing the model’s physiological relevance (Fig. 3).
Figure 3: The development of hybrid approaches can provide more realistic disease models. This figure is covered by the Biorender license.
While 3D-engineered in vitro ND models hold immense promise, several challenges need to be addressed. These include improving the reproducibility and standardization of model generation, enhancing the complexity and maturation of neuronal networks, and integrating multiple cell types and functions into the models. Future research should focus on addressing these challenges to maximize the potential of 3D-engineered models.
In conclusion, in vitro ND models are one of the remarkable areas open to development. By establishing more accurate and realistic in vitro disease models, faster and more effective drug discovery will be achieved, and personalized patient care will be improved in NDs.
Acknowledgement: None.
Funding Statement: The authors received no specific funding for this study. Zehra Gül Morçimen is supported by the TUBITAK-BIDEB 2211-A National Scholarship Program for Ph.D. Students and the YOK 100/2000 Scholarship in the field of smart and innovative materials.
Author Contributions: The authors confirm contribution to the paper as follows: literature review and discussion: Morçimen ZG, Taşdemir Ş, Şendemir A; draft manuscript preparation: Morçimen ZG, Taşdemir Ş, Şendemir A. All authors reviewed and approved the final version of the manuscript.
Availability of Data and Materials: Data sharing not applicable to this article as no datasets were generated or analyzed during the current study.
Ethics Approval: Not applicable.
Conflicts of Interest: The authors declare that they have no conflicts of interest to report regarding the present study.
References
1. Matilla-Dueñas A, Corral-Juan M, Rodríguez-Palmero Seuma A, Vilas D, Ispierto L, Morais S, et al. Rare neurodegenerative diseases: clinical and genetic update. Adv Exp Med Biol. 2017;1031:443–96. doi:https://doi.org/10.1007/978-3-319-67144-4_25/COVER. [Google Scholar] [CrossRef]
2. Takebe T, Imai R, Ono S. The current status of drug discovery and development as originated in united states academia: the influence of industrial and academic collaboration on drug discovery and development. Clin Transl Sci. 2018;11(6):533–624. doi:https://doi.org/10.1111/cts.v11.6. [Google Scholar] [CrossRef]
3. Klonarakis M, De Vos M, Woo EK, Ralph LT, Thacker JS, Gil-Mohapel J. The three sisters of fate: genetics, pathophysiology and outcomes of animal models of neurodegenerative diseases. Neurosci Biobehav Rev. 2022;135:104541. doi:https://doi.org/10.1016/j.neubiorev.2022.104541. [Google Scholar] [PubMed] [CrossRef]
4. Patar A, Dockery P, Howard L, McMahon SS. Cell viability in three ex vivo rat models of spinal cord injury. J Anat. 2019;234:244–51. doi:https://doi.org/10.1111/joa.12909. [Google Scholar] [PubMed] [CrossRef]
5. Lee HK, Velazquez Sanchez C, Chen M, Morin PJ, Wells JM, Hanlon EB, et al. Three-dimensional human neuro-spheroid model of Alzheimer’s disease based on differentiated induced pluripotent stem cells. PLoS One. 2016;11(9):e0163072. doi:https://doi.org/10.1371/journal.pone.0163072. [Google Scholar] [PubMed] [CrossRef]
6. Simunovic M, Brivanlou AH. Embryoids, organoids and gastruloids: new approaches to understanding embryogenesis. Development. 2017;144(6):976–85. doi:https://doi.org/10.1242/dev.143529. [Google Scholar] [PubMed] [CrossRef]
7. Qian XY, Nam Nguyen H, Song MM, Tang H, Song H, Ming G. Brain-region-specific organoids using mini-bioreactors for modeling ZIKV exposure. Cell. 2016;165(5):1238–54. doi:https://doi.org/10.1016/j.cell.2016.04.032. [Google Scholar] [PubMed] [CrossRef]
8. Lancaster MA, Knoblich JA. Organogenesis in a dish: modeling development and disease using organoid technologies. Science. 2014;345(6194):21. doi:https://doi.org/10.1126/science.1247125. [Google Scholar] [PubMed] [CrossRef]
9. Shah SB, Singh A. Cellular self-assembly and biomaterials-based organoid models of development and diseases. Acta Biomat. 2017;15(53):29–45. doi:https://doi.org/10.1016/j.actbio.2017.01.075. [Google Scholar] [PubMed] [CrossRef]
10. Wang H. Modeling neurological diseases with human brain organoids. Front Synaptic Neurosci. 2018;10:346589. doi:https://doi.org/10.3389/fnsyn.2018.00015/BIBTEX. [Google Scholar] [CrossRef]
11. Di Lullo E, Kriegstein AR. The use of brain organoids to investigate neural development and disease. Nat Rev Neurosci. 2017;18(10):573–84. doi:https://doi.org/10.1038/nrn.2017.107. [Google Scholar] [PubMed] [CrossRef]
12. Mason JO, Price DJ. Building brains in a dish: prospects for growing cerebral organoids from stem cells. Neurosci. 2016;334:105–18. doi:https://doi.org/10.1016/j.neuroscience.2016.07.048. [Google Scholar] [PubMed] [CrossRef]
13. Grebenyuk S, Ranga A. Engineering organoid vascularization. Front Bioeng Biotech. 2019;7:39. doi:https://doi.org/10.3389/fbioe.2019.00039. [Google Scholar] [PubMed] [CrossRef]
14. Mansour AA, Gonçalves JT, Bloyd CW, Li H, Fernandes S, Quang D, et al. An in vivo model of functional and vascularized human brain organoids. Nat Biotechnol. 2018;36(5):432–41. doi:https://doi.org/10.1038/nbt.4127. [Google Scholar] [PubMed] [CrossRef]
15. Yin X, Mead BE, Safaee H, Langer R, Karp JM, Levy O. Engineering stem cell organoids. Cell Stem Cell. 2016;18(1):25–38. doi:https://doi.org/10.1016/j.stem.2015.12.005. [Google Scholar] [PubMed] [CrossRef]
16. Giandomenico SL, Lancaster MA. Probing human brain evolution and development in organoids. Curr Opin Cell Biol. 2017;44:36–43. doi:https://doi.org/10.1016/j.ceb.2017.01.001. [Google Scholar] [PubMed] [CrossRef]
17. Mansour AAF, Schafer ST, Gage FH. Cellular complexity in brain organoids: current progress and unsolved issues. Semin Cell Dev Biol. 2021;111:32–9. doi:https://doi.org/10.1016/j.semcdb.2020.05.013. [Google Scholar] [PubMed] [CrossRef]
18. de Pieri A, Rochev Y, Zeugolis DI. Scaffold-free cell-based tissue engineering therapies: advances, shortfalls and forecast. Npj Regen Med. 2021;6(1):1–15. doi:https://doi.org/10.1038/s41536-021-00133-3. [Google Scholar] [PubMed] [CrossRef]
19. Nikolova MP, Chavali MS. Recent advances in biomaterials for 3D scaffolds: A review. Bioact Mat. 2019;4(2019):271–92. doi:https://doi.org/10.1016/j.bioactmat.2019.10.005. [Google Scholar] [PubMed] [CrossRef]
20. Eltom A, Zhong G, Muhammad A. Scaffold techniques and designs in tissue engineering functions and purposes: a review. Adv Mat Sci Eng. 2019;2019:3429527. doi:https://doi.org/10.1155/2019/3429527. [Google Scholar] [CrossRef]
21. Zhang F, King MW. Biodegradable polymers as the pivotal player in the design of tissue engineering scaffolds. Adv Healthc Mat. 2020;9(13):1901358. doi:https://doi.org/10.1002/adhm.201901358. [Google Scholar] [PubMed] [CrossRef]
22. Ma P, Chen Y, Lai X, Zheng J, Ye E, Loh XJ, et al. The translational application of hydrogel for organoid technology: challenges and future perspectives. Macromol Biosci. 2021;21(10):2100191. doi:https://doi.org/10.1002/mabi.202100191. [Google Scholar] [PubMed] [CrossRef]
23. Kasper C, Egger D, Senatov F, Raghunath M, Chariyev-Prinz F, Louit A, et al. In vitro 3D modeling of neurodegenerative diseases. Bioeng. 2023;10(1):93. doi:https://doi.org/10.3390/BIOENGINEERING10010093. [Google Scholar] [PubMed] [CrossRef]
24. Nichols E, Steinmetz JD, Vollset SE, Fukutaki K, Chalek J, Abd-Allah F. Estimation of the global prevalence of dementia in 2019 and forecasted prevalence in 2050: an analysis for the global burden of disease study 2019. Lancet Public Health. 2022;7(2):e105–25. doi:https://doi.org/10.1016/S2468-2667(21)00249-8. [Google Scholar] [PubMed] [CrossRef]
25. Slanzi A, Iannoto G, Rossi B, Zenaro E, Constantin G. In vitro models of neurodegenerative diseases. Front Cell Dev Biol. 2020;8:534138. doi:https://doi.org/10.3389/fcell.2020.00328/BIBTEX. [Google Scholar] [CrossRef]
26. Balin BJ, Hudson AP. Etiology and pathogenesis of late-onset Alzheimer’s disease. Curr Allergy Asthma Rep. 2014;14(3):1–10. doi:https://doi.org/10.1007/s11882-013-0417-1/METRICS. [Google Scholar] [CrossRef]
27. Ulaganathan S, Pitchaimani A. Spontaneous and familial models of Alzheimer’s disease: challenges and advances in preclinical research. Life Sci. 2023;328:121918. doi:https://doi.org/10.1016/j.lfs.2023.121918. [Google Scholar] [PubMed] [CrossRef]
28. Henley DB, Sundell KL, Sethuraman G, Dowsett SA, May PC. Safety profile of semagacestat, a gamma-secretase inhibitor: IDENTITY trial findings. Curr Med Res Opin. 2014;30(10):2021–32. doi:https://doi.org/10.1185/03007995.2014.939167. [Google Scholar] [PubMed] [CrossRef]
29. More SV, Kumar H, Cho DY, Yun YS, Choi DK. Toxin-induced experimental models of learning and memory impairment. Int J Mol Sci. 2016;17(9):1447. doi:https://doi.org/10.3390/ijms17091447. [Google Scholar] [PubMed] [CrossRef]
30. Cole GM, Morihara T, Lim GP, Yang F, Begum A, Frautschy SA. NSAID and antioxidant prevention of Alzheimer’s disease: lessons from in vitro and animal models. Ann N Y Acad Sci. 2004;1035(1):68–84. doi:https://doi.org/10.1196/annals.1332.005. [Google Scholar] [PubMed] [CrossRef]
31. Ferreira ST, Vieira MNN, De Felice FG. Soluble protein oligomers as emerging toxins in Alzheimer’s and other amyloid diseases. IUBMB Life. 2007;59(4–5):332–45. doi:https://doi.org/10.1080/15216540701283882. [Google Scholar] [PubMed] [CrossRef]
32. Ghosh S, Ali R, Verma S. Aβ-oligomers: A potential therapeutic target for Alzheimer’s disease. Int J Biol Macromol. 2023;239:124231. doi:https://doi.org/10.1016/j.ijbiomac.2023.124231. [Google Scholar] [PubMed] [CrossRef]
33. Madhu P, Mukhopadhyay S. Distinct types of amyloid-β oligomers displaying diverse neurotoxicity mechanisms in Alzheimer’s disease. J Cell Biochem. 2021;122(11):1594–1608. doi:https://doi.org/10.1002/jcb.30141. [Google Scholar] [PubMed] [CrossRef]
34. Vazin T, Ball KA, Lu H, Park H, Ataeijannati Y, Head-Gordon T, et al. Efficient derivation of cortical glutamatergic neurons from human pluripotent stem cells: a model system to study neurotoxicity in Alzheimer’s disease. Neurobiol Dis. 2014;62(477–86):62–72. doi:https://doi.org/10.1016/j.nbd.2013.09.005. [Google Scholar] [PubMed] [CrossRef]
35. Sengupta P, Garai K, Sahoo B, Shi Y, Callaway DJE, Maiti S. The amyloid β peptide (Aβ1-40) is thermodynamically soluble at physiological concentrations. Biochem. 2003;42(35):10506–13. doi:https://doi.org/10.1021/bi0341410. [Google Scholar] [PubMed] [CrossRef]
36. Hu M, Waring JF, Gopalakrishnan M, Li J. Role of GSK-3β activation and α7 nAChRs in Aβ1-42-induced tau phosphorylation in PC12 cells. J Neurochem. 2008;106(3):1371–7. doi:https://doi.org/10.1111/j.1471-4159.2008.05483.x. [Google Scholar] [PubMed] [CrossRef]
37. Qiu C, Wang YP, Pan XD, Liu XY, Chen Z, Liu LB. Exendin-4 protects Aβ(1-42) oligomer-induced PC12 cell apoptosis. Am J Transl Res. 2016;8(8):3540–8. [Google Scholar] [PubMed]
38. Hadipour E, Tayarani-Najaran Z, Fereidoni M. Vitamin K2 protects PC12 cells against Aβ(1-42) and H2O2-induced apoptosis via p38 MAP kinase pathway. Nutr Neurosci. 2018;23(5):343–52. doi:https://doi.org/10.1080/1028415X.2018.1504428. [Google Scholar] [PubMed] [CrossRef]
39. Barak M, Fedorova V, Pospisilova V, Raska J, Vochyanova S, Sedmik J, et al. Human iPSC-derived neural models for studying Alzheimer’s disease: from neural stem cells to cerebral organoids. Stem Cell Rev Rep. 2022;18(2):792–820. doi:https://doi.org/10.1007/s12015-021-10254-3. [Google Scholar] [PubMed] [CrossRef]
40. Yagi T, Ito D, Okada Y, Akamatsu W, Nihei Y, Yoshizaki T, et al. Modeling familial Alzheimer’s disease with induced pluripotent stem cells. Hum Mol Genet. 2011;20(23):4530–9. doi:https://doi.org/10.1093/hmg/ddr394. [Google Scholar] [PubMed] [CrossRef]
41. Raja WK, Mungenast AE, Lin YT, Ko T, Abdurrob F, Seo J, et al. Self-organizing 3D human neural tissue derived from induced pluripotent stem cells recapitulate Alzheimer’s disease phenotypes. PLoS One. 2016;11(9):e0161969. doi:https://doi.org/10.1371/journal.pone.0161969. [Google Scholar] [PubMed] [CrossRef]
42. Zhang D, Pekkanen-Mattila M, Shahsavani M, Falk A, Teixeira AI, Herland A. 3D Alzheimer’s disease culture model and the induction of P21-activated kinase mediated sensing in iPSC derived neurons. Biomaterials. 2014;35(5):1420–8. doi:https://doi.org/10.1016/j.biomaterials.2013.11.028. [Google Scholar] [PubMed] [CrossRef]
43. Oksanen M, Petersen AJ, Naumenko N, Puttonen K, Lehtonen Š., Gubert Olivé M, et al. PSEN1 mutant iPSC-derived model reveals severe astrocyte pathology in Alzheimer’s disease. Stem Cell Rep. 2017;9(6):1885. doi:https://doi.org/10.1016/j.stemcr.2017.10.016. [Google Scholar] [PubMed] [CrossRef]
44. Ranjan VD, Qiu L, Lee JWL, Chen X, Jang SE, Chai C, et al. A microfiber scaffold-based 3D in vitro human neuronal culture model of Alzheimer’s disease. Biomat Sci. 2020;8(17):4861–74. doi:https://doi.org/10.1039/D0BM00833H. [Google Scholar] [PubMed] [CrossRef]
45. Guo Hong C, Shui Jin S, Jia Jun Y, Jian Ren L, Hai Dong G. Designer self-assemble peptides maximize the therapeutic benefits of neural stem cell transplantation for alzheimer’s disease via enhancing neuron differentiation and paracrine action. Mol Neurobiol. 2016;53(2):1108–23. doi:https://doi.org/10.1007/s12035-014-9069-y/FIGURES/10. [Google Scholar] [CrossRef]
46. Choi SH, Kim YH, Hebisch M, Sliwinski C, Lee S, D’Avanzo C, et al. A three-dimensional human neural cell culture model of Alzheimer’s disease. Nat. 2014;515(7526):274–8. doi:https://doi.org/10.1038/nature13800. [Google Scholar] [PubMed] [CrossRef]
47. Cairns DM, Rouleau N, Parker RN, Walsh KG, Gehrke L, Kaplan DL. A 3D human brain-like tissue model of herpes-induced Alzheimer’s disease. Sci Adv. 2020;6(19):eaay8828. doi:https://doi.org/10.1126/sciadv.aay8828. [Google Scholar] [PubMed] [CrossRef]
48. Park J, Wetzel I, Marriott I, Dréau D, D’Avanzo C, Kim DY, et al. A 3D human triculture system modeling neurodegeneration and neuroinflammation in Alzheimer’s disease. Nat Neurosci. 2018;21(7):941–51. doi:https://doi.org/10.1038/s41593-018-0175-4. [Google Scholar] [PubMed] [CrossRef]
49. Jorfi M, D’avanzo C, Tanzi RE, Kim DY, Irimia D. Human neurospheroid arrays for in vitro studies of Alzheimer’s disease. Sci Rep. 2018;8(1):2450. doi:https://doi.org/10.1038/s41598-018-20436-8. [Google Scholar] [PubMed] [CrossRef]
50. Shin Y, Choi H, Kim E, Bylykbashi E, Kim JA, Chung S, et al. Blood-brain barrier dysfunction in a 3D in vitro model of Alzheimer’s disease. Adv Sci. 2019;6(20):1900962. doi:https://doi.org/10.1002/advs.201900962. [Google Scholar] [PubMed] [CrossRef]
51. Cho H, Hashimoto T, Wong E, Hori Y, Wood LB, Zhao L, et al. Microfluidic chemotaxis platform for differentiating the roles of soluble and bound amyloid-β on microglial accumulation. Sci Rep. 2013;3. doi:https://doi.org/10.1038/SREP01823. [Google Scholar] [PubMed] [CrossRef]
52. Pedrosa DJ, Timmermann L. Review: management of Parkinson’s disease. Neuropsychiatr Dis Treat. 2013;9:321–40. doi:https://doi.org/10.2147/NDT. [Google Scholar] [CrossRef]
53. Goetz CG. The history of Parkinson’s disease: early clinical descriptions and neurological therapies. Cold Spring Harb Perspect Med. 2011;1(1):a008862. doi:https://doi.org/10.1101/cshperspect.a008862. [Google Scholar] [PubMed] [CrossRef]
54. Thornton E, Vink R. Treatment with a substance p receptor antagonist is neuroprotective in the intrastriatal 6-hydroxydopamine model of early Parkinson’s disease. PLoS One. 2012;7(4):e34138. doi:https://doi.org/10.1371/journal.pone.0034138. [Google Scholar] [PubMed] [CrossRef]
55. Zeng XS, Geng WS, Jia JJ, Chen L, Zhang PP. Cellular and molecular basis of neurodegeneration in Parkinson disease. Front Aging Neurosci. 2018;17(10):109. doi:https://doi.org/10.3389/fnagi.2018.00109/BIBTEX. [Google Scholar] [CrossRef]
56. Cheng M, Zhang X, Wang M, Huang H, Ma J. A facile electrochemical sensor based on well-dispersed graphene-molybdenum disulfide modified electrode for highly sensitive detection of dopamine. J Electroanal Chem. 2017;786:1–7. doi:https://doi.org/10.1016/j.jelechem.2017.01.012. [Google Scholar] [CrossRef]
57. Lázaro DF, Pavlou MAS, Outeiro TF. Cellular models as tools for the study of the role of alpha-synuclein in Parkinson’s disease. Exp Neurol. 2017;298:162–71. doi:https://doi.org/10.1016/j.expneurol.2017.05.007. [Google Scholar] [PubMed] [CrossRef]
58. Mai JK, Paxinos G. The human nervous system. Elsevier Academic Press; 2012. [Google Scholar]
59. Varešlija D, Tipton KF, Davey GP, McDonald AG. 6-Hydroxydopamine: a far from simple neurotoxin. J Neural Transm. 2020;127(2):213–30. doi:https://doi.org/10.1007/s00702-019-02133-6. [Google Scholar] [PubMed] [CrossRef]
60. Walkinshaw G, Waters CM. Neurotoxin-induced cell death in neuronal PC12 cells is mediated by induction of apoptosis. Neurosci. 1994;63(4):975–87. doi:https://doi.org/10.1016/0306-4522(94)90566-5. [Google Scholar] [PubMed] [CrossRef]
61. Blum D, Torch S, Nissou MF, Benabid AL, Verna JM. Extracellular toxicity of 6-hydroxydopamine on PC12 cells. Neurosci Lett. 2000;283(3):193–6. doi:https://doi.org/10.1016/S0304-3940(00)00948-4. [Google Scholar] [PubMed] [CrossRef]
62. Blum D, Torch S, Lambeng N, Nissou MF, Benabid AL, Sadoul R, et al. Molecular pathways involved in the neurotoxicity of 6-OHDA, dopamine and MPTP: contribution to the apoptotic theory in Parkinson’s disease. Prog Neurobiol. 2001;65(2):135–72. doi:https://doi.org/10.1016/S0301-0082(01)00003-X. [Google Scholar] [PubMed] [CrossRef]
63. Salari S, Bagheri M. In vivo, in vitro and pharmacologic models of Parkinson’s disease. Physiol Res. 2019;68:17–24. doi:https://doi.org/10.33549/physiolres.933895. [Google Scholar] [PubMed] [CrossRef]
64. Chlebanowska P, Tejchman A, Sułkowski M, Skrzypek K, Majka M. Use of 3D organoids as a model to study idiopathic form of Parkinson’s disease. Int J Mol Sci. 2020;21(3):694. doi:https://doi.org/10.3390/ijms2103069421. [Google Scholar] [CrossRef]
65. Son MY, Sim H, Son YS, Jung KB, Lee MO, Oh JH, et al. Distinctive genomic signature of neural and intestinal organoids from familial Parkinson’s disease patient-derived induced pluripotent stem cells. Neuropathol Appl Neurobiol. 2017;43(7):584–603. doi:https://doi.org/10.1111/nan.12396. [Google Scholar] [PubMed] [CrossRef]
66. Nickels SL, Modamio J, Mendes-Pinheiro B, Monzel AS, Betsou F, Schwamborn JC. Reproducible generation of human midbrain organoids for in vitro modeling of Parkinson’s disease. Stem Cell Res. 2020;46:101870. doi:https://doi.org/10.1016/j.scr.2020.101870. [Google Scholar] [PubMed] [CrossRef]
67. Abdelrahman S, Alsanie WF, Khan ZN, Albalawi HI, Felimban RI, Moretti M, et al. A Parkinson’s disease model composed of 3D bioprinted dopaminergic neurons within a biomimetic peptide scaffold. Biofabrication. 2022;14(4):044103. doi:https://doi.org/10.1088/1758-5090/ac7eec. [Google Scholar] [PubMed] [CrossRef]
68. Chemmarappally JM, Pegram HCN, Abeywickrama N, Fornari E, Hargreaves AJ, de Girolamo LA, et al. A co-culture nanofibre scaffold model of neural cell degeneration in relevance to Parkinson’s disease. Sci Rep. 2020;10(1):2767. doi:https://doi.org/10.1038/s41598-020-59310-x. [Google Scholar] [PubMed] [CrossRef]
69. Fiore NJ, Ganat YM, Devkota K, Batorsky R, Lei M, Lee K, et al. Bioengineered models of Parkinson’s disease using patient-derived dopaminergic neurons exhibit distinct biological profiles in a 3D microenvironment. Cell Mol Life Sci. 2022;79(2):1–20. doi:https://doi.org/10.1007/s00018-021-04047-7. [Google Scholar] [PubMed] [CrossRef]
70. Freundt EC, Maynard N, Clancy EK, Roy S, Bousset L, Sourigues Y, et al. Neuron-to-neuron transmission of α-synuclein fibrils through axonal transport. Ann Neurol. 2012;72(4):517–24. doi:https://doi.org/10.1002/ana.23747. [Google Scholar] [PubMed] [CrossRef]
71. Moreno EL, Hachi S, Hemmer K, Trietsch SJ, Baumuratov AS, Hankemeier T, et al. Differentiation of neuroepithelial stem cells into functional dopaminergic neurons in 3D microfluidic cell culture. Lab Chip. 2015;15(11):2419–28. doi:https://doi.org/10.1039/C5LC00180C. [Google Scholar] [PubMed] [CrossRef]
72. Bolognin S, Fossépré M, Qing X, Jarazo J, Ščančar J, Moreno EL, et al. 3D cultures of Parkinson’s Disease-specific dopaminergic neurons for high content phenotyping and drug testing. Adv Sci. 2019;6(1):1800927. doi:https://doi.org/10.1002/advs.201800927. [Google Scholar] [PubMed] [CrossRef]
73. Kane KIW, Moreno EL, Hachi S, Walter M, Jarazo J, Oliveira MAP, et al. Automated microfluidic cell culture of stem cell derived dopaminergic neurons. Sci Rep. 2019;9(1):1–12. doi:https://doi.org/10.1038/s41598-018-34828-3. [Google Scholar] [PubMed] [CrossRef]
74. Lublin FD. New multiple sclerosis phenotypic classification. Eur Neurol. 2014;72(Suppl. 1):1–5. doi:https://doi.org/10.1159/000367614. [Google Scholar] [PubMed] [CrossRef]
75. Lublin FD. Reingold defining the clinical course of multiple sclerosis: results of an international survey. National Multiple Sclerosis Society (USA) advisory committee on clinical trials of new agents in multiple sclerosis. Neurol. 1996;46(4):907–11. doi:https://doi.org/10.1212/WNL.46.4.907. [Google Scholar] [PubMed] [CrossRef]
76. Thompson AJ, Banwell BL, Barkhof F, Carroll WM, Coetzee T, Comi G, et al. Diagnosis of multiple sclerosis: 2017 revisions of the McDonald criteria. Lancet Neurol. 2018;17(2):162–73. doi:https://doi.org/10.1016/S1474-4422(17)30470-2. [Google Scholar] [PubMed] [CrossRef]
77. Dendrou CA, Fugger L, Friese MA. Immunopathology of multiple sclerosis. Nat Rev Immun. 2015;15(9):545–58. doi:https://doi.org/10.1038/nri3871. [Google Scholar] [PubMed] [CrossRef]
78. Chard DT, Griffin CM, Parker GJM, Kapoor R, Thompson AJ, Miller DH. Brain atrophy in clinically early relapsing-remitting multiple sclerosis. Brain. 2002;125(2):327–37. doi:https://doi.org/10.1093/brain/awf025. [Google Scholar] [PubMed] [CrossRef]
79. Popescu BF, Lucchinetti CF. Meningeal and cortical grey matter pathology in multiple sclerosis. BMC Neurol. 2012;12(1):1–8. doi:https://doi.org/10.1186/1471-2377-12-11/FIGURES/4. [Google Scholar] [CrossRef]
80. Unal DB, Caliari SR, Lampe KJ. Engineering biomaterial microenvironments to promote myelination in the central nervous system. Brain Res Bull. 2019;152(6):159–74. doi:https://doi.org/10.1016/j.brainresbull.2019.07.013. [Google Scholar] [PubMed] [CrossRef]
81. Kipp M, van der Star B, Vogel DYS, Puentes F, van der Valk P, Baker D, et al. Experimental in vivo and in vitro models of multiple sclerosis: EAE and beyond. Mult Scler Relat Disord. 2012;1(1):15–28. doi:https://doi.org/10.1016/j.msard.2011.09.002. [Google Scholar] [PubMed] [CrossRef]
82. Madill M, Fitzgerald D, O’Connell KE, Dev KK, Shen S, FitzGerald U. In vitro and ex vivo models of multiple sclerosis. Drug Discov Today. 2016;21(9):1504–11. doi:https://doi.org/10.1016/j.drudis.2016.05.018. [Google Scholar] [PubMed] [CrossRef]
83. Lee S, Leach MK, Redmond SA, Chong SYC, Mellon SH, Tuck SJ, et al. A culture system to study oligodendrocyte myelination processes using engineered nanofibers. Nat Methods. 2012;9(9):917–22. doi:https://doi.org/10.1038/nmeth.2105. [Google Scholar] [PubMed] [CrossRef]
84. Walsh AD, Nguyen LT. Binder MD miRNAs in microglia: important players in multiple sclerosis pathology. ASN Neuro. 2021;13(3):1759091420981182. doi:https://doi.org/10.1177/1759091420981182. [Google Scholar] [PubMed] [CrossRef]
85. Birgbauer E, Rao TS, Webb M. Lysolecithin induces demyelination in vitro in a cerebellar slice culture system. J Neurosci Res. 2004;78(2):157–66. doi:https://doi.org/10.1002/jnr.20248. [Google Scholar] [PubMed] [CrossRef]
86. Dehghan S, Aref E, Raoufy MR, Javan M. An optimized animal model of lysolecithin induced demyelination in optic nerve; more feasible, more reproducible, promising for studying the progressive forms of multiple sclerosis. J Neurosci Meth. 2021;352:109088. doi:https://doi.org/10.1016/j.jneumeth.2021.109088. [Google Scholar] [PubMed] [CrossRef]
87. Pavelko KD, van Engelen BGM, Rodriguez M. Acceleration in the rate of CNS remyelination in lysolecithin-induced demyelination. J Neurosci. 1998;18(7):2498–505. doi:https://doi.org/10.1523/JNEUROSCI.18-07-02498.1998. [Google Scholar] [PubMed] [CrossRef]
88. Zhang M, Hugon G, Bouillot C, Bolbos R, Langlois JB, Billard T, et al. Evaluation of myelin radiotracers in the lysolecithin rat model of focal demyelination: Beware of pitfalls!. Contrast Media Mol Imaging. 2019;2019(5):9294586. doi:https://doi.org/10.1155/2019/9294586. [Google Scholar] [PubMed] [CrossRef]
89. Desai RA, Davies AL, Tachrount M, Kasti M, Laulund F, Golay X, et al. Cause and prevention of demyelination in a model multiple sclerosis lesion. Ann Neurol. 2016;79(4):591–604. doi:https://doi.org/10.1002/ana.24607. [Google Scholar] [PubMed] [CrossRef]
90. Felts PA, Woolston AM, Fernando HB, Asquith S, Gregson NA, Mizzi OJ, et al. Inflammation and primary demyelination induced by the intraspinal injection of lipopolysaccharide. Brain. 2005;128(7):1649–66. doi:https://doi.org/10.1093/brain/awh516. [Google Scholar] [PubMed] [CrossRef]
91. Skripuletz T, Miller E, Grote L, Gudi V, Pul R, Voss E, et al. Lipopolysaccharide delays demyelination and promotes oligodendrocyte precursor proliferation in the central nervous system. Brain Behav Immun. 2011;25(8):1592–606. doi:https://doi.org/10.1016/j.bbi.2011.05.009. [Google Scholar] [PubMed] [CrossRef]
92. Zhang F, Yao SY, Whetsell WO, Sriram S. Astrogliopathy and oligodendrogliopathy are early events in CNS demyelination. Glia. 2013;61(8):1261–73. doi:https://doi.org/10.1002/glia.22513. [Google Scholar] [PubMed] [CrossRef]
93. Guerriero C, Puliatti G, Di Marino T, Tata AM. Effects mediated by dimethyl fumarate on in vitro oligodendrocytes: implications in multiple sclerosis. Int J Mol Sci. 2022;23(7):3615. doi:https://doi.org/10.3390/ijms23073615. [Google Scholar] [PubMed] [CrossRef]
94. Fries M, Mertens M, Teske N, Kipp M, Beyer C, Willms T, et al. Water-soluble cuprizone derivative: synthesis, characterization, and in vitro studies. ACS Omega. 2019;4(1):1685–9. doi:https://doi.org/10.1021/acsomega.8b02523. [Google Scholar] [CrossRef]
95. Bonetto G, Charalampopoulos I, Gravanis A, Karagogeos D. The novel synthetic microneurotrophin BNN27 protects mature oligodendrocytes against cuprizone-induced death, through the NGF receptor TrkA. Glia. 2017;65(8):1376–94. doi:https://doi.org/10.1002/glia.23170. [Google Scholar] [PubMed] [CrossRef]
96. Rodnichenko A. Implementation of a toxic cuprizone model of demyelination in vitro. Cell Organ Transplantol. 2018;6(1):93–8. doi:https://doi.org/10.22494/COT.V6I1.84. [Google Scholar] [CrossRef]
97. Vega-Riquer JM, Mendez-Victoriano G, Morales-Luckie RA, Gonzalez-Perez O. Five decades of cuprizone, an updated model to replicate demyelinating diseases. Curr Neuropharmacol. 2019;17(2):129–41. doi:https://doi.org/10.2174/1570159X15666170717120343. [Google Scholar] [PubMed] [CrossRef]
98. Chan JR, Watkins TA, Cosgaya JM, Zhang C, Chen L, Reichardt LF, et al. NGF controls axonal receptivity to myelination by schwann cells or oligodendrocytes. Neuron. 2004;43(2):183. doi:https://doi.org/10.1016/j.neuron.2004.06.024. [Google Scholar] [PubMed] [CrossRef]
99. Baumann N, Pham-Dinh D. Biology of oligodendrocyte and myelin in the mammalian central nervous system. Physiol Rev. 2001;81(2):871–927. doi:https://doi.org/10.1152/physrev.2001.81.2.871. [Google Scholar] [PubMed] [CrossRef]
100. Campagnoni AT, Macklin WB. Cellular and molecular aspects of myelin protein gene expression. Mol Neurobiol. 1988;2(1):41–89. doi:https://doi.org/10.1007/BF02935632. [Google Scholar] [PubMed] [CrossRef]
101. Stadelmann C, Timmler S, Barrantes-Freer A, Simons M. Myelin in the central nervous system: structure, function, and pathology. Physiol Rev. 2019;99(3):1381–431. doi:https://doi.org/10.1152/physrev.00031.2018. [Google Scholar] [PubMed] [CrossRef]
102. Post GR, Dawson G. Characterization of a cell line derived from a human oligodendroglioma. Mol Chem Neuropathol. 1992;16(3):303–17. doi:https://doi.org/10.1007/BF03159976. [Google Scholar] [PubMed] [CrossRef]
103. Buntinx M, Gielen E, Van Hummelen P, Raus J, Ameloot M, Steels P, et al. Cytokine-induced cell death in human oligodendroglial cell lines. II: alterations in gene expression induced by interferon-γ and tumor necrosis factor-α. J Neurosci Res. 2004;76(6):846–61. doi:https://doi.org/10.1002/jnr.20117. [Google Scholar] [PubMed] [CrossRef]
104. Martínez-Pinilla E, Rubio-Sardón N, Peláez R, García-álvarez E, Del Valle E, Tolivia J, et al. Neuroprotective effect of apolipoprotein D in cuprizone-induced cell line models: a potential therapeutic approach for multiple sclerosis and demyelinating diseases. Int J Mol Sci. 2021;22(3):1260. doi:https://doi.org/10.3390/IJMS22031260. [Google Scholar] [PubMed] [CrossRef]
105. Martínez-pinilla E, Rubio-sardón N, Villar-conde S, Navarro G, Del Valle E, Tolivia J, et al. Cuprizone-induced neurotoxicity in human neural cell lines is mediated by a reversible mitochondrial dysfunction: relevance for demyelination models. Brain Sci. 2021;11(2):272. doi:https://doi.org/10.3390/brainsci11020272. [Google Scholar] [PubMed] [CrossRef]
106. Blanchard JW, Victor MB, Tsai LH. Dissecting the complexities of Alzheimer disease with in vitro models of the human brain. Nat Rev Neurol. 2021;18(1):25–39. doi:https://doi.org/10.1038/s41582-021-00578-6. [Google Scholar] [PubMed] [CrossRef]
107. Marton RM, Miura Y, Sloan SA, Li Q, Revah O, Levy RJ, et al. Differentiation and maturation of oligodendrocytes in human three-dimensional neural cultures. Nat Neurosci. 2019;22(3):484. doi:https://doi.org/10.1038/s41593-018-0316-9. [Google Scholar] [PubMed] [CrossRef]
108. Madhavan M, Nevin ZS, Shick HE, Garrison E, Clarkson-Paredes C, Karl M, et al. Induction of myelinating oligodendrocytes in human cortical spheroids. Nat Methods. 2018;15(9):700–6. doi:https://doi.org/10.1038/s41592-018-0081-4. [Google Scholar] [PubMed] [CrossRef]
109. Campbell Baker N. Therapeutic effect of mesencymal stem cell derşved extracellular vesicles on 3D model of oligocortical spheroids (Ph.D. Thesis). Boston University: USA; 2022. [Google Scholar]
110. Chesnut M, Paschoud H, Repond C, Smirnova L, Hartung T, Zurich MG, et al. Human IPSC-derived model to study myelin disruption. Int J Mol Sci. 2021;22(17):9473. doi:https://doi.org/10.3390/ijms22179473/S1. [Google Scholar] [CrossRef]
111. Rosenberg SS, Kelland EE, Tokar E, De La Torre AR, Chan JR. The geometric and spatial constraints of the microenvironment induce oligodendrocyte differentiation. Proc Natl Acad Sci USA. 2008;105(38):14662–7. doi:https://doi.org/10.1073/pnas.0805640105. [Google Scholar] [PubMed] [CrossRef]
112. Jakobsson A, Ottosson M, Zalis MC, O’Carroll D, Johansson UE, Johansson F. Three-dimensional functional human neuronal networks in uncompressed low-density electrospun fiber scaffolds. Nanomed: NBM. 2017;13(4):1563–73. doi:https://doi.org/10.1016/J.NANO.2016.12.023. [Google Scholar] [PubMed] [CrossRef]
113. Kaur N, Han W, Li Z, Madrigal MP, Shim S, Pochareddy S, et al. Neural stem cells direct axon guidance via their radial fiber scaffold. Neuron. 2020;107(6):1197–211.e9. doi:https://doi.org/10.1016/j.neuron.2020.06.035. [Google Scholar] [PubMed] [CrossRef]
114. Zhang N, Milbreta U, Chin JS, Pinese C, Lin J, Shirahama H, et al. Biomimicking fiber scaffold as an effective in vitro and in vivo microRNA screening platform for directing tissue regeneration. Adv Sci. 2022;9(14):e2201954. doi:https://doi.org/10.1002/ADVS.201800808. [Google Scholar] [PubMed] [CrossRef]
115. Espinosa-Hoyos D, Jagielska A, Homan KA, Du H, Busbee T, Anderson DG, et al. Engineered 3D-printed artificial axons. Sci Rep. 2018;8(1):478. doi:https://doi.org/10.1038/s41598-017-18744-6. [Google Scholar] [PubMed] [CrossRef]
116. Shahidi S, Janmaleki M, Riaz S, Sanati Nezhad A, Syed N. A tuned gelatin methacryloyl (GelMA) hydrogel facilitates myelination of dorsal root ganglia neurons in vitro. Mater Sci Eng C Mater Biol Appl. 2012;126(3):112131. doi:https://doi.org/10.1016/J.MSEC.2021.112131. [Google Scholar] [PubMed] [CrossRef]
117. Wu S, Chen MS, Maurel P, Lee YS, Bunge MB, Arinzeh TL. Aligned fibrous PVDF-TrFE scaffolds with Schwann cells support neurite extension and myelination in vitro. J Neural Eng. 2018;15(5):056010. doi:https://doi.org/10.1088/1741-2552/aac77f. [Google Scholar] [PubMed] [CrossRef]
118. Yao L, Phan F, Li Y. Collagen microsphere serving as a cell carrier supports oligodendrocyte progenitor cell growth and differentiation for neurite myelination in vitro. Stem Cell Res Ther. 2013;4(5):1–8. doi:https://doi.org/10.1186/scrt320/FIGURES/4. [Google Scholar] [CrossRef]
119. Ristola M, Sukki L, Azevedo MM, Seixas AI, Relvas JB, Narkilahti S, et al. A compartmentalized neuron-oligodendrocyte co-culture device for myelin research: design, fabrication and functionality testing. J Micromech Microeng. 2019;29(6):065009. doi:https://doi.org/10.1088/1361-6439/ab16a7. [Google Scholar] [CrossRef]
120. Sharma AD, McCoy L, Jacobs E, Willey H, Behn JQ, Nguyen H, et al. Engineering a 3D functional human peripheral nerve in vitro using the nerve-on-a-chip platform. Sci Rep. 2019;9(1):1–12. doi:https://doi.org/10.1038/s41598-019-45407-5. [Google Scholar] [PubMed] [CrossRef]
121. Lee SR, Hyung S, Bang S, Lee Y, Ko J, Lee S, et al. Modeling neural circuit, blood-brain barrier, and myelination on a microfluidic 96 well plate. Biofabrication. 2019;11(3):035013. doi:https://doi.org/10.1088/1758-5090/ab1402. [Google Scholar] [PubMed] [CrossRef]
122. Osaki T, Shin Y, Sivathanu V, Campisi M, Kamm RD. In vitro microfluidic models for neurodegenerative disorders. Adv Healthc Mate. 2018;7(2):1700489. doi:https://doi.org/10.1002/adhm.201700489. [Google Scholar] [PubMed] [CrossRef]
123. Cisbani G, Cicchetti F. An in vitro perspective on the molecular mechanisms underlying mutant huntingtin protein toxicity. Cell Death Dis. 2012;3(8):e382. doi:https://doi.org/10.1038/cddis.2012.121. [Google Scholar] [PubMed] [CrossRef]
124. Scotter EL, Goodfellow CE, Graham ES, Dragunow M, Glass M. Neuroprotective potential of CB1 receptor agonists in an in vitro model of Huntington’s disease. Br J Pharmacol. 2010;160(3):747–61. doi:https://doi.org/10.1111/j.1476-5381.2010.00773.x. [Google Scholar] [PubMed] [CrossRef]
125. Wang J, Pfleger CM, Friedman L, Vittorino R, Zhao W, Qian X, et al. Potential application of grape derived polyphenols in huntington’s disease. Transl Neurosci. 2010;1(2):95. doi:https://doi.org/10.2478/v10134-010-0022-y. [Google Scholar] [PubMed] [CrossRef]
126. Jeon I, Lee N, Li JY, Park IH, Park KS, Moon J, et al. Neuronal properties, in vivo effects, and pathology of a Huntington’s disease patient-derived induced pluripotent stem cells. Stem Cells. 2012;30(9):2054–62. doi:https://doi.org/10.1002/stem.1135. [Google Scholar] [PubMed] [CrossRef]
127. Mattis VB, Svendsen SP, Ebert A, Svendsen CN, King AR, Casale M, et al. Induced pluripotent stem cells from patients with Huntington’s disease show CAG-repeat-expansion-associated phenotypes. Cell Stem Cell. 2012;11(2):264–78. doi:https://doi.org/10.1016/j.stem.2012.04.027. [Google Scholar] [PubMed] [CrossRef]
128. Vigont V, Nekrasov E, Shalygin A, Gusev K, Klushnikov S, Illarioshkin S, et al. Patient-specific iPSC-based models of Huntington’s disease as a tool to study store-operated calcium entry drug targeting. Front Pharmacol. 2018;9:384042. doi:https://doi.org/10.3389/fphar.2018.00696/BIBTEX. [Google Scholar] [CrossRef]
129. Camnasio S, Carri AD, Lombardo A, Grad I, Mariotti C, Castucci A, et al. The first reported generation of several induced pluripotent stem cell lines from homozygous and heterozygous Huntington’s disease patients demonstrates mutation related enhanced lysosomal activity. Neurobiol Dis. 2012;46(1):41–51. doi:https://doi.org/10.1016/j.nbd.2011.12.042. [Google Scholar] [PubMed] [CrossRef]
130. Virlogeux A, Moutaux E, Christaller W, Genoux A, Bruyère J, Fino E, et al. Reconstituting corticostriatal network on-a-chip reveals the contribution of the presynaptic compartment to Huntington’s disease. Cell Rep. 2018;22(1):110–22. doi:https://doi.org/10.1016/j.celrep.2017.12.013. [Google Scholar] [PubMed] [CrossRef]
131. Lenoir S, Lahaye RA, Vitet H, Scaramuzzino C, Virlogeux A, Capellano L, et al. Pridopidine rescues BDNF/TrkB trafficking dynamics and synapse homeostasis in a Huntington disease brain-on-a-chip model. Neurobiol Dis. 2022;173:105857. doi:https://doi.org/10.1016/j.nbd.2022.105857. [Google Scholar] [PubMed] [CrossRef]
132. Akhtar A, Farzamrad V, Moradi AR, Yar M, Bazzar M. Emerging polymeric biomaterials and manufacturing-based tissue engineering approaches for neuro regeneration-A critical review on recent effective approaches. Smart Mater Med. 2023;4(7):337–55. doi:https://doi.org/10.1016/j.smaim.2022.11.007. [Google Scholar] [CrossRef]
133. Hardy JG, Lin P, Schmidt CE. Biodegradable hydrogels composed of oxime crosslinked poly(ethylene glycolhyaluronic acid and collagen: a tunable platform for soft tissue engineering. J Biomater Sci Polym Ed. 2015;26(3):143–61. doi:https://doi.org/10.1080/09205063.2014.975393. [Google Scholar] [PubMed] [CrossRef]
134. Collins SJ, Haigh CL. Simplified murine 3D neuronal cultures for investigating neuronal activity and neurodegeneration. Cell Biochem Biophys. 2017;75(1):3–13. doi:https://doi.org/10.1007/s12013-016-0768-z. [Google Scholar] [PubMed] [CrossRef]
135. Matamoros-Angles A, Gayosso LM, Richaud-Patin Y, di Domenico A, Vergara C, Hervera A, et al. iPS cell cultures from a gerstmann-sträussler-scheinker patient with the Y218N PRNP mutation recapitulate tau pathology. Mol Neurobiol. 2018;55(4):3033–48. doi:https://doi.org/10.1007/s12035-017-0506-6. [Google Scholar] [PubMed] [CrossRef]
136. Gois AM, Mendonça DMF, Freire MAM, Santos JR. In vitro and in vivo models of amyotrophis lateral sclerosis: an updated overview. Brain Res Bull. 2020;159:32–43. doi:https://doi.org/10.1016/j.brainresbull.2020.03.012. [Google Scholar] [PubMed] [CrossRef]
137. Hawrot J, Imhof S, Wainger BJ. Modeling cell-autonomous motor neuron phenotypes in ALS using iPSCs. Neurobiol Dis. 2020;134(10):104680. doi:https://doi.org/10.1016/j.nbd.2019.104680. [Google Scholar] [PubMed] [CrossRef]
138. Imamura K, Izumi Y, Watanabe A, Tsukita K, Woltjen K, Yamamoto T, et al. The Src/c-Abl pathway is a potential therapeutic target in amyotrophic lateral sclerosis. Sci Transl Med. 2017;9(391):eaaf3962. doi:https://doi.org/10.1126/scitranslmed.aaf3962. [Google Scholar] [PubMed] [CrossRef]
139. Chen H, Qian K, Du Z, Cao J, Petersen A, Liu H, et al. Modeling ALS with iPSCs reveals that mutant SOD1 misregulates neurofilament balance in motor neurons. Cell Stem Cell. 2014;14(6):796–809. doi:https://doi.org/10.1016/j.stem.2014.02.004. [Google Scholar] [PubMed] [CrossRef]
140. Strickland JB, Davis-Anderson K, Micheva-Viteva S, Twary S, Iyer R, Harris JF, et al. Optimization of application-driven development of in vitro neuromuscular junction models. Tissue Eng Part B Rev. 2022;28(6):1180–91. doi:https://doi.org/10.1089/ten.teb.2021.0204. [Google Scholar] [PubMed] [CrossRef]
141. Vila OF, Qu Y, Vunjak-Novakovic G. In vitro models of neuromuscular junctions and their potential for novel drug discovery and development. Expert Opin Drug Discov. 2019;15(3):307–17. doi:https://doi.org/10.1080/17460441.2020.1700225. [Google Scholar] [PubMed] [CrossRef]
142. Park HS, Liu S, McDonald J, Thakor N, Yang IH. Neuromuscular junction in a microfluidic device. Annu Int Conf IEEE Eng Med Biol Soc. 2013;2013:2833–5. doi:https://doi.org/10.1109/EMBC.2013.6610130. [Google Scholar] [PubMed] [CrossRef]
143. Southam KA, King AE, Blizzard CA, McCormack GH, Dickson TC. Microfluidic primary culture model of the lower motor neuron-neuromuscular junction circuit. J Neurosci Meth. 2013;218(2):164–9. doi:https://doi.org/10.1016/j.jneumeth.2013.06.002. [Google Scholar] [PubMed] [CrossRef]
144. Santhanam N, Kumanchik L, Guo X, Sommerhage F, Cai Y, Jackson M, et al. Stem cell derived phenotypic human neuromuscular junction model for dose response evaluation of therapeutics. Biomaterials. 2018;166:64–78. doi:https://doi.org/10.1016/j.biomaterials.2018.02.047. [Google Scholar] [PubMed] [CrossRef]
145. Morimoto Y, Kato-Negishi M, Onoe H, Takeuchi S. Three-dimensional neuron-muscle constructs with neuromuscular junctions. Biomaterials. 2013;34(37):9413–9. doi:https://doi.org/10.1016/j.biomaterials.2013.08.062. [Google Scholar] [PubMed] [CrossRef]
146. Bakooshli MA, Lippmann ES, Mulcahy B, Iyer N, Nguyen CT, Tung K, et al. A 3D culture model of innervated human skeletal muscle enables studies of the adult neuromuscular junction. Elife. 2019;14(8):e44530. doi:https://doi.org/10.7554/eLife.44530.033. [Google Scholar] [CrossRef]
147. Osaki T, Uzel SGM, Kamm RD. Microphysiological 3D model of amyotrophic lateral sclerosis (ALS) from human iPS-derived muscle cells and optogenetic motor neurons. Sci Adv. 2018;4(10):eaat5847. doi:https://doi.org/10.1126/sciadv.aat5847. [Google Scholar] [PubMed] [CrossRef]
148. Osaki T, Sivathanu V, Kamm RD. Engineered 3D vascular and neuronal networks in a microfluidic platform. Sci Rep. 2018;8(1):5168. doi:https://doi.org/10.1038/s41598-018-23512-1. [Google Scholar] [PubMed] [CrossRef]
149. Timmerman R, Burm SM, Bajramovic JJ. An overview of in vitro methods to study microglia. Front Cell Neurosci. 2018;12:242. doi:https://doi.org/10.3389/fncel.2018.00242. [Google Scholar] [PubMed] [CrossRef]
150. Watson PMD, Kavanagh E, Allenby G, Vassey M. Bioengineered 3D glial cell culture systems and applications for neurodegeneration and neuroinflammation. SLAS Discov: Adv Life Sci. 2017;22(5):583–601. doi:https://doi.org/10.1177/2472555217691450. [Google Scholar] [PubMed] [CrossRef]
151. Abud EM, Ramirez RN, Martinez ES, Healy LM, Nguyen CHH, Newman SA, et al. iPSC-derived human microglia-like cells to study neurological diseases. Neuron. 2017;94(2):278–93.e9. doi:https://doi.org/10.1016/j.neuron.2017.03.042. [Google Scholar] [PubMed] [CrossRef]
152. Pires LR, Rocha DN, Ambrosio L, Pêgo AP. The role of the surface on microglia function: implications for central nervous system tissue engineering. J R Soc Interface. 2015;12(103):20141224. doi:https://doi.org/10.1098/rsif.2014.1224. [Google Scholar] [PubMed] [CrossRef]
153. Haw RTY, Tong CK, Yew A, Lee HC, Phillips JB, Vidyadaran S. A three-dimensional collagen construct to model lipopolysaccharide-induced activation of BV2 microglia. J Neuroinflamm. 2014;11(1):841. doi:https://doi.org/10.1186/1742-2094-11-134. [Google Scholar] [PubMed] [CrossRef]
154. Pöttler M, Zierler S, Kerschbaum HH. An artificial three-dimensional matrix promotes ramification in the microglial cell-line, BV-2. Neurosci Lett. 2006;410(2):137–40. doi:https://doi.org/10.1016/j.neulet.2006.09.082. [Google Scholar] [PubMed] [CrossRef]
155. Zhou K, Motamed S, Thouas GA, Bernard CC, Li D, Parkington HC, et al. Graphene functionalized scaffolds reduce the inflammatory response and supports endogenous neuroblast migration when implanted in the adult brain. PLoS One. 2016;11(3):e0151589. doi:https://doi.org/10.1371/journal.pone.0151589. [Google Scholar] [PubMed] [CrossRef]
156. Cryan JF, O’riordan KJ, Cowan CSM, Sandhu KV, Bastiaanssen TFS, Boehme M, et al. The microbiota-gut-brain axis. Physiol Rev. 2019;99(4):1877–2013. doi:https://doi.org/10.1152/physrev.00018.2018. [Google Scholar] [PubMed] [CrossRef]
157. Boeri L, Perottoni S, Izzo L, Giordano C, Albani D. Microbiota-host immunity communication in neurodegenerative disorders: bioengineering challenges for in vitro modeling. Adv Healthc Mater. 2021;10(7):2002043. doi:https://doi.org/10.1002/adhm.202002043. [Google Scholar] [PubMed] [CrossRef]
158. Duval K, Grover H, Han LH, Mou Y, Pegoraro AF, Fredberg J, et al. Modeling physiological events in 2D vs. 3D cell culture. Physiology. 2017;32(4):266–77. doi:https://doi.org/10.1152/physiol.00036.2016. [Google Scholar] [PubMed] [CrossRef]
159. Sun M, Liu A, Yang X, Gong J, Yu M, Yao X, et al. 3D cell culture—can it be as popular as 2D cell culture? Adv Nanobiomed Res. 2021;1(5):2000066. doi:https://doi.org/10.1002/anbr.202000066. [Google Scholar] [CrossRef]
160. Whitehouse C, Corbett N, Brownlees J. 3D models of neurodegeneration: implementation in drug discovery. Trends Pharmacol Sci. 2023b;44(4):208–21. doi:https://doi.org/10.1016/j.tips.2023.01.005. [Google Scholar] [PubMed] [CrossRef]
161. Gitler AD, Dhillon P, Shorter J. Neurodegenerative disease: models, mechanisms, and a new hope. Dis Model Mech. 2017;10(5):499–502. doi:https://doi.org/10.1242/dmm.030205. [Google Scholar] [PubMed] [CrossRef]
162. Nikolakopoulou P, Rauti R, Voulgaris D, Shlomy I, Maoz BM, Herland A. Recent progress in translational engineered in vitro models of the central nervous system. Brain. 2020;143(11):3181–213. doi:https://doi.org/10.1093/brain/awaa268. [Google Scholar] [PubMed] [CrossRef]
163. Slanzi A, Iannoto G, Rossi B, Zenaro E, Constantin G. In vitro models of neurodegenerative diseases. Front Cell Dev Biol. 2020b;8:328. doi:https://doi.org/10.3389/FCELL.2020.00328/FULL. [Google Scholar] [CrossRef]
164. Fontoura JC, Viezzer C, dos Santos FG, Ligabue RA, Weinlich R, Puga RD, et al. Comparison of 2D and 3D cell culture models for cell growth, gene expression and drug resistance. Mater Sci Eng C Mater Biol Appl. 2020;107:110264. doi:https://doi.org/10.1016/j.msec.2019.110264. [Google Scholar] [PubMed] [CrossRef]
165. Habanjar O, Diab-Assaf M, Caldefie-Chezet F, Delort L. 3D cell culture systems: tumor application, advantages, and disadvantages. Int J Mol Sci. 2021;22(22):12200. doi:https://doi.org/10.3390/ijms222212200. [Google Scholar] [PubMed] [CrossRef]
166. Centeno EGZ, Cimarosti H, Bithell A. 2D versus 3D human induced pluripotent stem cell-derived cultures for neurodegenerative disease modelling. Mol Neurodegener. 2018;13(1):1–15. doi:https://doi.org/10.1186/s13024-018-0258-4. [Google Scholar] [PubMed] [CrossRef]
167. Siney EJ, Kurbatskaya K, Chatterjee S, Prasannan P, Mudher A, Willaime-Morawek S, et al. Modelling neurodegenerative diseases in vitro: recent advances in 3D iPSC technologies. AIMS Cell Tissue Eng. 2018;2(1):1–23. doi:https://doi.org/10.3934/celltissue.2018.1.1. [Google Scholar] [CrossRef]
Cite This Article
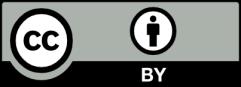
This work is licensed under a Creative Commons Attribution 4.0 International License , which permits unrestricted use, distribution, and reproduction in any medium, provided the original work is properly cited.