Open Access
ARTICLE
Anti-proliferative effect of Annona extracts on breast cancer cells
1 Biomolecular Sciences Institute, Florida International University, Miami, 33199, USA
2 Department of Biological Sciences, Florida International University, Miami, 33199, USA
3 Biochemistry Ph.D. Program, Florida International University, Miami, 33199, USA
4 Fairchild Tropical Botanic Garden, Coral Gables, 33156, USA
5 International Center of Tropical Botany, Florida International University, Miami, 33199, USA
* Corresponding Author: Manuel A. Barbieri,
(This article belongs to the Special Issue: Natural Products for Chronic Inflammatory Diseases: Pharmacology and Toxicology)
BIOCELL 2023, 47(8), 1835-1852. https://doi.org/10.32604/biocell.2023.029076
Received 31 January 2023; Accepted 01 June 2023; Issue published 28 August 2023
Abstract
Backgorund: Fruits and seed extracts of Annona montana have significant cytotoxic potential in several cancer cells. This study evaluates the effect of A. montana leaves hexane extract on several signaling cascades and gene expression in metastatic breast cancer cells upon insulin-like growth factor-1 (IGF-1) stimulation. Methods: MTT assay was performed to determine the proliferation of cancer cells. Propidium iodide staining and flow cytometry analysis of Annexin V binding was utilized to measure the progression of the cell cycle and the induction of apoptosis. Protein expression and phosphorylation were determined by western blotting analysis to examine the underlying cellular mechanism triggered upon treatment with A. montana leaves hexane extract. Results: A. montana leaves hexane (subfraction V) blocked the constitutive stimulation of the PI3K/mTOR signaling pathways. This inhibitory effect was associated with apoptosis induction as evidenced by the positivity with Annexin V and terminal deoxynucleotidyl transferase dUTP nick end labeling (TUNNEL) staining, activation of caspase-3, and cleavage of PPAR. It also limited the expression of various downstream genes that regulate proliferation, survival, metastasis, and angiogenesis (i.e., cyclin D1, survivin, COX-2, and VEGF). It increased the expression of p53 and p21. Interestingly, we also observed that this extract blocked the activation of AKT and ERK without affecting the phosphorylation of the IGF-1 receptor and activation of Ras upon IGF-1 stimulation. Conclusion: Our study indicates that A. montana leaves (sub-fraction V) extract exhibits a selective anti-proliferative and proapoptotic effect on the metastatic MDA-MB-231 breast cancer cells through the involvement of PI3K/AKT/mTOR/S6K1 pathways.Keywords
Based on the World Health Organization (WHO) report, more than 80% of the world population depends on traditional and alternative medicine for their primary healthcare needs (Adams, 2007; Sharifi-Rad et al., 2019; WHO, 2019). More than 60% of used anti-cancer drugs originate from a natural source. Still, their active constituents and cellular/molecular targets have yet to be fully understood. The anti-cancer activity of most natural products is often related to regulating immune function, inducing apoptosis or autophagy, or inhibiting uncontrolled cell growth and invasion (Huang et al., 2021; Yang et al., 2021; Lederer and Huber, 2022). The anti-cancer activity of most natural products is often related to regulating signaling transduction pathways, inducing apoptosis, or inhibiting uncontrolled cell growth and invasion (Sharifi-Rad et al., 2019; Huang et al., 2021). Cell signal transduction is regulated by various cell surface receptors (i.e., insulin growth factor-1 receptor (IGF-1R)). IGF-1 plays a crucial role in cellular physiology by modulating several signaling pathways, such as the mitogen-activated protein kinases (MAPKs) and phosphatidylinositol-4,5-bisphosphate 3-kinase (PI3K)/protein kinase B (Akt)/mammalian target of rapamycin (mTOR). These are essential enzymes regulated via several intracellular stimuli and control key cellular functions, such as proliferation, survival, and growth (Sharma et al., 2022; Zhang et al., 2022). Several species of genus Annona, in particular, known for a range of biomedical applications as well as in folk medicine, have been investigated (Rupprecht et al., 1990; Chang and Wu, 2001; Pardhasaradhi et al., 2005; Lim, 2012; Pratheeshkumar et al., 2012; Ribeiro et al., 2013; Formagio et al., 2015; Kumar et al., 2015; Tundis et al., 2017). Several studies have been conducted on A. muricata and A. squamosa, focusing on their anti-cancer properties against different cancer cell lines (Wang et al., 2014; Syed Najmuddin et al., 2016). Other studies revealed the anti-proliferative effect of A. cherimola leaves and seeds on cancer and leukemic cells (Suresh et al., 2011; Arunjyothi et al., 2012; Haykal et al., 2019). Interestingly, aqueous and methanol extracts from A. muricata leaves lowered the blood glucose level and showed antioxidant effects on diabetic rats (Adeyemi et al., 2008). Recent studies revealed the anti-proliferative effect of A. montana fruit on several cancer cell lines. Extracts from its fruits, seeds, and leaves have significant cytotoxic potential in several cancer cells (Wu et al., 1995; Wang et al., 2001; Chuang et al., 2008; Alvarez Colom et al., 2009; Bailon-Moscoso et al., 2016). Furthermore, methanol, hexane, and ethyl acetate extracts from A. montana reduced the formation of fat cells (Leung et al., 2022). Administering extracts prepared from A. squamosa also inhibits glycemic and lipemic indices and the levels of the antioxidant enzymes (i.e., glutathione reductase) (Gupta et al., 2005; Kaleem et al., 2006). However, there are currently no studies on the effect of extracts from the A. montana leaves on the signaling transduction pathways driven by IGF-1 in breast cancer cells. Thus, due to the vital role of IGF-1 on PI3K-Akt-mTOR and MAPKs signaling pathways in cellular functioning and pathologic modification (AsghariHanjani and Vafa, 2019), we decided to investigate whether hexane extracts of A. montana utilize their anti-cancer effects by modulating the PI3K/AKT/mTOR/S6K1 and MAPK signaling driven by IGF-1 in breast cancer cells. Thus, natural compounds in A. montana extracts could lead to the dysregulation of gene expression and inhibition of cell proliferation that affect apoptosis and promote proliferation, migration, and invasion in cancer cells. In addition, we analyzed the anti-proliferative effects of A. montana extracts on several human tumor cell lines.
Chemicals, reagents, and antibodies
All cell lines (HeLa, HepG2, A549, A431, MCF-7, PCS-600-010, MDA-MD-231, MCF-7, and SK-MEL-28) were purchased from American Type Culture Collection (ATCC, Manassas, VA). Dulbecco’s modified Eagles medium-high glucose (DMEM), penicillin/streptomycin, and L-glutamine were purchased from Mediatech, Inc. (Manassas, VA). Unless otherwise indicated, all antibodies were acquired from Cell Signaling Technology (Boston, MA). Glyceraldehyde 3-phosphate dehydrogenase (GAPDH) antibodies were obtained from Sigma-Aldrich (St. Louis, MO). All secondary antibodies were purchased from Jackson ImmunoResearch Laboratories (West Grove, PA). IGF-1 was purchased from Cell Signaling Technology (Beverly, MA). Inhibitors (i.e., Wortmannin, PF-3758309, FR-180204) were obtained from Cayman Chemical. ISIS2503 oligonucleotide inhibitor was prepared as previously described (Chen et al., 1996). Other chemicals were obtained from Sigma unless otherwise stated.
Preparation of Annona montana extract
A. montana leaves were collected from the Redland region (Possum Trot Tropical Farm) in Miami, Florida, USA, by Dr. M.A. Barbieri and Mr. R. Barnum. Our botanist verified the taxonomic identity at the herbarium of the Department of Biological Sciences at Florida International University (Voucher: FTG182701, Fairchild Herbarium). The leaves of A. montana were separated from one individual plant and cleaned using running tap water. Then, the leaves were oven-dried in a convection oven at 60°C for 32 h. Dried leaves (2000 mg) were cut into small pieces and extracted with methanol (100 mL) in a 250 mL round bottom flask connected to a reflux condenser heated at 65°C for 30 min. The plant materials were filtered and washed twice with methanol (25 mL). All materials (extract and washes) were collected and pooled. Evaporation of the methanol extract by rotavapor/desiccation yielded a residue of 1180 mg for A. montana methanol extract (AmMe). Then each methanol extract was either dissolved in dimethyl sulfoxide (DMSO) at a final concentration of 100 mg/mL plant extract or partitioned with hexane, ethyl acetate, and water, respectively, as described early (Chin et al., 2014). An aliquot (518 mg A. montana methanol extract) of the residue from the methanol extraction was partitioned between methanol and hexane. The hexane was removed from the methanolic phase and treated as previously described (Priestap et al., 2014). Finally, the residue was partitioned between water and ethyl acetate. All fractions collected (i.e., hexane-AmH-, ethyl acetate-Amea- and water-Amw-) were then individually evaporated and concentrated by rotorvapor/desiccation as previously described (Priestap et al., 2014). The hexane, ethyl acetate, and water extracts yielded 223, 102, and 95 mg of residue for A. montana, representing 82% of the initial methanol extract. A pool of several hexane fractions was collected (1000 mg), and was further fractionated using gravity column chromatography with hexane-ethyl acetate to increase polarity up to 90% ethyl acetate, then 100% ethyl acetate yielded twelve sub-fractions of increasing polarity (F1-F12). Each sub-fraction was dried, dissolved in DMSO, and tested for activity cell proliferation, cell cycle, invasion, colony formation, and Western blotting analysis.
Gas chromatography (GC) and gas chromatography/mass spectrometry (GC/MS) analyses
GC/MS determinations were carried out in a Hewlett Packard model 6890 instrument coupled to a Q-Mass 910 quadrupole selective detector (Perkin-Elmer) as previously described (Chin et al., 2014; Priestap et al., 2014). GC/FID determinations were performed on a Trace GC Ultra apparatus (Thermo Electron Corporation), and the output was recorded using a ChromQuest version 4.1 data system. Analyses were performed on capillary columns DB-5MS at the abovementioned conditions (Adams, 2007; Araujo et al., 2007; Babushok et al., 2011; Yan et al., 2013).
All cell lines were cultured according to standard mammalian tissue culture protocols and sterile technique as indicated by ATCC. Cell lines were cultured for less than 2 months, splitting them twice a week in a humidified incubator with 5% CO2 at 37°C. Hela, SK-MEL-28, A431, A549, HepG2, MDA-MB-231, and MFC-7 cells were grown in DMEM (Gibco BRL, Gaithersburg, MD, USA) supplemented with 10% fetal bovine serum (FBS), 50 U/mL penicillin, and 50 μg/mL streptomycin. MCF-10A cells were grown in DMEM supplemented with 10% FBS, 50 U/mL penicillin, 50 μg/mL streptomycin, 10 ng/mL human epidermal growth factor (hEGF), 100 ng/mL cholera toxins, 5 μg/mL bovine insulin and 500 ng/mL hydrocortisone. PCS-600-010 cells were grown in mammary epithelial cell basal medium supplemented with 10% FBS, 50 U/mL penicillin, 50 μg/mL streptomycin, 100 ng/mL recombinant human transforming growth factor-alpha (rhTGF-α), 5 μg/mL Apotransferrin, 1 μM epinephrine, 5 μg/mL recombinant human insulin (rhI) and 100 ng/mL hydrocortisone. Cells were harvested at approximately 80% confluence and then plated for subsequent passage and treatments.
Cell cycle progression was determined by flow cytometry analysis (Alvarez et al., 2010). Cells were incubated in the absence or presence of A. montana extract for 24 h. A suspension of cells was fixed with methanol (70%) at 4°C, washed, and stained with propidium iodide (PI). Cells were analyzed with an Acurri-C6 Flow Cytometer (BD Biosciences, San Diego, CA), and cell cycle progression was measured with the Modfit LT program (Verity Software House, Topsham, ME).
The Annexin V/fluorescein isothiocyanate (FITC) assay was performed using Annexin V Kit (BD Pharmigen, USA) to analyze the potential of A. montana extract in causing apoptosis. (The Annexin V signal indicates cellular apoptosis, while PI staining indicates necrotic or late apoptosis) (Elmore et al., 2016). The cells were seeded in a 6-well plate at a concentration of 2.4 × 105 cells/mL and incubated overnight. The next day, the seeded cells were treated with A. montana extract and incubated for 24, 48, and 72 h. The cells were harvested at a time point, and the resulting pellets were resuspended in a binding buffer. To stain the cell suspension, 25 μL of FITC Annexin V and 5 μL of PI were added and allowed to stand in a dark place at room temperature for 15 min. Afterward, the stained cells were analyzed by an Acurri-C6 Flow Cytometer (BD Biosciences, San Diego, CA).
Terminal deoxynucleotidyl transferase dUTP nick end labeling (TUNEL) assay
Cells were seeded onto 6-well plates at a density of 1 × 106 cells per well, incubated for one day, and then treated with A. montana extract for 24 h. The cells were collected and washed with 1 mL phosphate buffer saline (PBS). Cells were fixed with paraformaldehyde for 30 min at room temperature, washed, and then permeabilized by 0.2% Triton X-100 for 15 min. After incubation, cells were washed and resuspended in 1 mL of PBS containing TUNEL solution (de Felice et al., 2019) and incubated for 1 h at 37°C in the dark. Afterward, the stained cells were analyzed by an Acurri-C6 Flow Cytometer (BD Biosciences, San Diego, CA).
Cells (3.5 × 105) were seeded in a 6-well plate and incubated overnight. To evaluate migration (non-directional migration) the next day, a straight wound line was drawn across the 100% confluent attached cell layer with pipette tips as previously described (Cappiello et al., 2018). Plates were washed to remove unattached cells with PBS and replaced with a fresh DMEM medium. A. montana extract was added to the wells, and images of the closure of the wound were recorded at 24 h using the inverted microscope equipped with a camera.
Cells were seeded in 96-well plates at 2 × 103 cells/well density in 100 μL medium and incubated overnight. The next day, the seeded cells were treated with A. montana extract or subfraction and incubated for 8 h (for extract) or 24, 48, and 72 h (for subfraction). After the incubation, the MTT solution (5 mg/mL) was added to the plates, and the cells were incubated at 37°C for 6 h. The formazan, derived from MTT by living cells, was dissolved in 10% SDS (150 μL per well), and the absorbance was measured at OD595 nm as described (Mosmann, 1983). All MTT experiments were performed in duplicate and repeated at least three times.
Cell migration and invasion assays
Cells (~70% confluent) were serum-starved for 24 h. Then, cells were seeded (2 × 105 cells/mL) in the insert chamber coated with solidified Matrigel (BD Biosciences) for the invasion assay, whereas, for the migration assay (directional migration), the Matrigel basement membrane did not coat the chamber. In the lower compartment of the chamber, 2 mL of DMEM supplemented with 10% FBS and the desired concentration of A. montana extract were added. The inserts were incubated in a 37°C CO2 incubator for 24 h. The inserts were removed afterward, and the inner side of the inserts was swabbed to remove the non-invaded cells. The outer side of the inserts bearing the migrated/invaded cells was then fixed in methanol for 30 min before being stained with 0.01% of crystal violet. Images of the membranes were acquired or counted under an inverted microscope with a camera (×100). The dye was extracted, and the absorbance reading was obtained using a spectrophotometer (Hulkower and Herber, 2011).
Anchorage-independent colony formation assay
For measurement of anchorage-independent growth, cells were trypsinized and resuspended in a culture medium containing 0.9% methylcellulose (Fluka, Saint Louis, MO) in DMEM containing 5% FBS. Aliquots of 1 mL containing 2 × 103 cells were plated on 35-mm bacteriological dishes in the absence or presence of A. montana extract containing 5% FBS. As previously described, colonies were counted under an inverted microscope after 14–16 days (Borowicz et al., 2014).
Cells were grown in a 48-well culture plate in DMEM with 10% FBS containing antibiotics and then incubated with either DMSO vehicle or A. montana extract for 24 h. After incubation, the released lactate dehydrogenase (LDH) into the culture supernatant was measured by the colorimetric LDH Cytotoxicity Assay (BioVision) according to the manufacturer’s instructions (Smith et al., 2011).
To prepare cell lysates, cell monolayers were washed with ice-cold lysis buffer (20 mM Tris–HCl pH 7.5, 150 mM NaCl, 1 mM Na2 EDTA, 1% NP40, and 0.1% Na deoxycholate) containing both protease and phosphatase inhibitors. Lysates were collected by centrifugation, and protein concentrations were estimated by using the BCA protein assay (Thermo Fisher Scientific, Inc., Pittsburgh, PA) following the manufacturer’s instructions. Proteins were resolved by sodium dodecyl sulfate-polyacrylamide gel electrophoresis (SDS-PAGE) and transferred to nitrocellulose membranes, which were blocked, probed with the specific antibodies, and then visualized by western blotting analysis. Relative levels of MMP-2 and MMP-9 proteins were determined by densitometry using the ratio of MMP-2 and MMP-9 to GAPDH. Relative levels of PI3K, AKT, SGK1, JNK, ERK, mTOR, p38, and RAF-1 were determined using the ratio of p-PI3K, p-AKT, p-SGK1, p-JNK, p-ERK, p-mTOR, p-p38, p-RAF-1 to t-PI3K, t-AKT, t-SGK1, t-JNK, t-ERK, t-mTOR, t-p38, and t-RAF-1, respectively. Relative levels of survivin, IAP-1, IAP-2, Cyclin-D1, COX-2, VEGF, Caspase, PARP, p53, IGF-1R, and p21 proteins were determined by densitometry using the ratio of these proteins to GAPDH as previously described (Tall et al., 2001).
Cells were lysed using a buffer containing 20 mM HEPES (pH 7.5), 100 mM NaCl, 1 mM dithiothreitol, 5 mM MgCl2, 5% (v/v) glycerol, and 1% (v/v) Triton-X-100 supplemented with 2 mM phenylmethylsulfonyl fluoride (lysis buffer). Lysates (1 mL) were then incubated with 100 μL of glutathione beads containing 10 μg of GST-RAF-RBD at 4°C while rocking for 1 h. After incubation, the beads were washed three times using Hank’s balanced salt solution (HBSS) with 5 mg/mL bovine serum albumin (BSA) (HBSS-BSA). The pull-downs were subjected to SDS-PAGE and analyzed by western blotting using an anti-Ras antibody as previously described (Camalier et al., 2010).
All experiments were done in duplicates, and they were repeated at least three times. Values are represented as the standard error of the mean of triplicates (SEM), and the statistical significance was analyzed by two-way ANOVA or two-tail student’s t-test. Results with *p < 0.05, **p < 0.01, and ***p < 0.001 were statistically significant.
Anti-proliferative effect of Annona montana extracts on several types of cancer cell lines
Cancer cells must continuously refurbish cellular metabolism to fulfill the demands of growth and proliferation (Fares et al., 2020). Cell proliferation was determined by comparing the survival of cells for 24 h in the untreated cells, which was normalized to 100%. Cells were treated once with different concentrations of three types of A. montana extracts (i.e., A. montana methanol, hexane, ethyl acetate, and water). Fig. 1A shows that the A. montana methanol extract inhibited the proliferation of human tumorigenic MCF-7 and metastatic MDA-MB-231 breast cancer cell lines. Specifically, the A. montana methanol extract shows an IC50 of 315 ± 15 μg/mL for MDA-MB-231 cells and an IC50 of 245 ± 8 μg/mL for MCF-7 cells, respectively. Fig. 1A also shows the effect of the A. montana methanol extract on the proliferation of MCF-10A, which was considerably lower. It only inhibited 19.5 ± 2.2% at 600 μg/mL. Consistent with this observation, we found that this A. montana methanol extract was unable to affect the viability of PCS-600-010 primary normal mammary epithelial cells (compare the viability of non-treated cells (92 ± 8%) with cells treated with 600 μg/mL of A. montana methanol extract (90 ± 6% cell viability) as evidenced by the quantification of LDH as described in Material and Methods section. Furthermore, adding 0.01% DMSO did not affect the cell viability of any of the treated cell lines (Figs. 1A and 1B). As a positive control, we used wortmannin, a potent inhibitor of phosphatidylinositol-4,5-bisphosphate 3-kinase (PI3K) activity, which also inhibited the proliferation of MDA-MB-231 and MCF-7 cells with 43 ± 9% and 57 ± 5% of inhibition, respectively. Furthermore, the addition of wortmannin (100 μg/mL; 0.233 μM) did not significantly affect the viability of MCF-10A cells (96 ± 4% cell viability) as compared with control cells (100 ± 3% cell viability), and it is consistent with our previous observations (Chin et al., 2014). These results suggest a strong activity in inhibiting the proliferation of the A. montana methanol extract on these cancer cells. To obtain additional information about the nature of the active compound in A. montana leaves, the methanol extract was subsequently fractionated as described in the Materials and Methods section. Three fractions (named hexane, ethyl acetate, and water fractions) were obtained. The A. montana hexane fraction exhibited the most potent inhibitory effect (IC50 164 ± 4 μg/mL) on cell proliferation of MDA-MB-231 cells as compared with the A. montana ethyl acetate fraction, which showed inhibition of cell proliferation with IC50 225 ± 5 μg/mL (Fig. 2A). Interestingly, the aqueous fraction did not show any effect (Fig. 2A), and more importantly, these A. montana fractions showed a limited inhibitory effect on the proliferation of MCF-10A cells (Fig. 2B). As expected, these fractions did not show a significant effect on the viability of MDA-MB-231 cells (compare the viability of non-treated cells (94 ± 6% cell viability) and 0.01% DMSO-treated cells (93 ± 5% cell viability) with cells treated with 300 µg/mL of A. montana hexane (94 ± 5% cell viability), ethyl acetate (95±3% cell viability) and water extracts (98 ± 2% cell viability) as evidenced by the quantification of LDH as described in Material and Methods (Fig. 2C). We also fractionated the hexane fraction with a combination of Hexane: Ethyl acetate in order of increasing polarity. Each sub-fraction was tested for its activity on cell proliferation. As shown in Table 1, we observed that A. montana hexane subfraction V, and in less extent, fraction VII, strongly decreased the proliferation of MBA-MD-231 cells. On the other hand, the IC50 results for the anti-proliferative effect of the A. montana hexane sub-fraction V on several types of cancer cell lines (i.e., HeLa, PC-3, HepG2, A549, A431, SK-MEL-28, MDA-MB-231, and MCF-7) are shown in Table 2. The proliferation of these cancer cell lines was susceptible to A. montana hexane subfraction V. A549, and SK-MEL-28 cell lines were the least affected by adding A. montana hexane subfraction V (i.e., high IC50). In contrast, the proliferation of HeLa and MCF-7 cell lines showed high activity inhibiting cell proliferation as they have lower IC50 values. Interestingly, HeLa and MCF-7 cell lines were the most affected after 72 h of treatment with A. montana hexane subfraction V. We also considered the possibility that the extent of inhibition is time-dependent. Thus, we then determine the effect of adding A. montana hexane subfraction V on the proliferation of MDA-MB-231 cells for up to 72 h. Specifically, MDA-MB-231 cells were incubated with 0.01% DMSO, 20 μg/mL, or 40 μg/mL of A. montana hexane extract for three different time intervals: 24, 48, and 72 h, respectively. After the last incubation, the cell proliferation was analyzed using an MTT assay described in Material and Methods. As shown in Fig. 3, 40 μg/mL of A. montana hexane subfraction V significantly suppressed cell proliferation of MDA-MB-231 cells in a time-dependent matter with potent inhibition of cell proliferation (>90% inhibition) at 72 h of treatment.
Figure 1: A dose-dependent inhibitory effect of Annona montana methanol extract on cell proliferation. (A) Cells (MCF-10A [⚫], MCF-7 [○], and MDA-MB-231 [◻]) were treated with several concentrations (0 to 600 μg/mL) of A. montana methanol extract for 8 h, respectively. Then, cellular proliferation was quantified spectroscopically using the MTT assay described in the Material and Methods. Dimethyl sulfoxide (0.01%) [◊] was used as a control with MCF-7 cells. IC50 was expressed as μg/mL. Data represent the mean ± SEM of three independent experiments. Results were expressed as a percentage of non-treated cells (control cells). *p < 0.05 and ***p < 0.001 as determined by two-way ANOVA followed by Tukey’s post hoc test. (B) Cells [MDA-MB-231(231), MCF-10A (10A), MCF-7 (7) PCS-600-100 (010)] were cultured in medium alone and containing either 0.01% dimethyl sulfoxide or 600 μg/mL of A. montana methanol extract for 8 h. Cell viability was determined by the LDH assay. All values are presented as the mean ± SEM of three experiments performed in triplicate. ns: it means not significant.
Figure 2: Effect of hexane, ethyl acetate, and water Anonna montana extracts on cell proliferation. Cells ((A) MDA-MB-231 (B): MCF-10) were treated with several concentrations (0 to 300 μg/mL) of hexane (○), ethyl acetate (◻), and water (⚫) fractions of A. montana methanol extract for 8 h. Then, cellular proliferation was quantified spectroscopically using the MTT assay described in the Material and Methods section. IC50 was expressed as μg/mL. Data represent the mean ± standard error of three independent experiments’ mean (SEM). Results were expressed as a percentage of non-treated cells (control cells). *p < 0.05 and ***p < 0.001 as determined by two-way analysis of variance (ANOVA) followed by Tukey’s post hoc test. (C) Cells (MDA-MB-231 and MCF-10A) were cultured in medium alone (-) and containing either 0.01% dimethyl sulfoxide (D) or 300 μg/mL of A. montana extracts (e.g., hexane-H, ethyl acetate-EA, water-W) for 8 h. Cell viability was determined by the LDH assay. All values are presented as the mean ± SEM of three experiments performed in triplicate. *p < 0.05 and ***p < 0.001 as determined by two-way ANOVA followed by Tukey’s post hoc test.
Figure 3: Annona montana hexane sub-fraction V suppressed cell proliferation. MDA-MB-231 cells were treated with 0.01% dimethyl sulfoxide (DMSO) alone (⚫), 20 μg/mL (◻), and 40 μg/mL (○) of hexane sub-fraction V of A. montana several times (24, 48, and 72 h). Cell proliferation was quantified by spectrophotometric analysis using MTT assay as described in Material and Methods. Results were expressed as a percentage of 0.01% DMSO-treated cells (control cells). Data represent the mean ± SEM of three independent experiments. *p < 0.05 and ***p < 0.001 as determined by two-way ANOVA followed by Tukey’s post hoc test.
Anonna montana hexane subfraction V extract induced apoptosis in MDA-MB-231 breast cancer cells
To further define the inhibitory effect of A. montana hexane sub-fraction V, we investigate the effect of this extract on the cell cycle by flow cytometry as described in Material and Methods. In Figs. 4A and 4B, we show that after incubation of cells with 20 μg/mL A. montana hexane sub-fraction V for 48 h, a significant increase in the SubG1 peak (i.e., untreated cells: 3 ± 0.2; treated cells: 27 ± 2.1). Also, the percentage of cell population in the Go/G1, S, and G2+M phases was significantly lower than in untreated cells. Taken together, our data indicate that treatment of cells with A. montana hexane sub-fraction V was effective and led to the suppression of cell proliferation and induced apoptosis, as evidenced by the increased accumulation of cells in the Sub-G1 phase of the cell cycle. Based on our observations that continued the exposure of A. montana hexane sub-fraction V to MDA-MB-231 cells strongly diminished cell proliferation with an increase in the Sub-G1 peak, we decided to investigate the effect of this extract on apoptosis. We stained MDA-MB-231 cells with Annexin V-FITC after treating them with 20 μg/mL of A. montana hexane subfraction V for 24 h. Figs. 5A and 5B shows that cells stained with Annexin V antibodies (regarded as early apoptosis) increase compared to non-treated cells (Control). We then examined for late apoptosis using the TUNEL assay. Again, apoptotic cells were significantly increased on treatment with A. montana hexane subfraction V for 24 h, as observed by flow cytometry (compare Figs. 5C and 5D). In Table 3, we also show more specific results of Annexin V FITC and PI staining of MDA-MB-231 cells treated with 20 μg/mL of A. montana hexane subfraction V at two different times intervals in addition to 24 h intervals (i.e., 48 and 72 h). A significant increase in the population of early apoptotic cells from 1.14% (untreated cells) to 21.70% (treated cells) when cells were incubated with 20 μg/mL A. montana hexane subfraction V for 48 h. Moreover, a further increase in the population of early apoptotic MDA-MB-231 cells after 72 h of incubation (i.e., untreated cells: 3.09; treated cells: 37.01). Similarly, we also observed an increase in the population of late apoptosis at 48 h (i.e., untreated cells: 1.33; treated cells: 12.21) and 72 h (i.e., untreated cells: 1.39; treated cells: 15.31). After treatment, dead cells were also increased with 20 μg/mL A. montana hexane subfraction V (Table 3). These results suggest that A. montana hexane subfraction V arrested the cell cycle at the Sub-G0/G1 phase and induced apoptosis in vitro in a time-dependent manner.
Figure 4: Histogram analysis of the cell cycle in MDA-MB-231 cells in the presence of Annona montana hexane subfraction. (A, B) MDA-MB-231 cells were treated with 0.01% DMSO alone (control) or 20 μg/mL of hexane sub-fraction V (hexane-V) of A. montana for 8 h. Then, the cells were fixed, stained with propidium iodide, and analyzed using a flow cytometer as described in the Material and Methods section. The data are representative of one of four independent experiments. Inset: Data represent the mean ± SEM of four independent experiments.
Figure 5: Annona montana hexane subfraction V induced apoptosis in MDA-MB-231 cells. MDA-MB-231 cells were treated with 0.01% DMSO alone (control) or 20 μg/mL of hexane sub-fraction V (hexane-V) of A. montana for 24 h. Then, the cells were fixed, stained with a FITC-conjugated Annexin V antibody (A, B), incubated using TUNEL reaction solution (C, D), and then analyzed using a flow cytometer as described in Material and Methods. The data are representative of one of four independent experiments.
The anti-metastatic potential of Anonna montana hexane subfraction V extract on MDA-MB-231 cells
The anti-metastatic abilities of the A. montana hexane subfraction V were evaluated in MDA-MB-231 cells by using several in vitro assays (i.e., wound healing analysis, migration, invasion, and clonogenic assay). In Fig. 6A, we show a significant decrease in the percentage of wound closure in the A. montana hexane subfraction V extract-treated MDA-MB-231 cells compared with untreated cells (compare 67 ± 4% of closure of treated cells vs. 100% closure for untreated-control cells) after 48 h incubation. We also observed that cells treated with A. montana hexane sub-fraction V inhibited migration (83 ± 7% inhibition) through the Transwell membrane compared to untreated cells (Fig. 6B). Then, we evaluated the invasive capacity of MDA-MB-231 cells in an in vitro system. From the Matrigel invasion assay, as shown in Fig. 6C, the ability of MDA-MB-231 cells to invade this loaded membrane was significantly compromised (72 ± 5% inhibition invasion) in the A. montana hexane sub-fraction V-treated cells relative to the control cells. We also investigated the effect of the A. montana hexane sub-fraction V on the ability of MDA-MB-231 breast cancer cells to form colonies to understand better the inhibitory effect of A. montana hexane sub-fraction V on MDA-MB-231 breast cancer cells. From the clonogenic assay, as shown in Fig. 6D, the ability of MDA-MB-231 cells to form colonies was significantly reduced (64 ± 5% inhibition of invasion) in the A. montana hexane sub-fraction V-treated cells relative to the control cells. In addition, the inhibitory effect of A. montana hexane subfraction V on all these assays was time and concentration-dependent (data not shown). Furthermore, we observed that the protein expressions of MMP-2 and MMP-9 were analyzed to evaluate the effect of A. montana hexane sub-fraction V on the motility of MDA-MB-231 cells. It showed that A. montana hexane sub-fraction V significantly suppressed the protein expressions of MMP-2 (84 ± 5% inhibition of protein expression) and MMP-9 (54 ± 3% inhibition of protein expression) (Fig. 6E). Thus, these results indicated that A. montana hexane sub-fraction V significantly inhibited the migration, invasion, and colony formation of MDA-MB-231 breast cancer cells in vitro. These observations are consistent with the fact that MMP-2 and MMP-9 proteins are closely related to the migration and invasion of various types of cancer cells (Brown and Murray, 2015; Folgueras et al., 2004).
Figure 6: Effect of Annona montana hexane subfraction V on migration, invasion, and colony formation. MDA-MB-231 cell migration was analyzed by wound healing assay (scale bar is 200 µm) (A) or by Transwell invasion assay (scale bar is 50 µm) (B) in the presence of 0.1% dimethyl sulfoxide (DMSO) alone (control) or 20 µg/mL of hexane subfraction V (hexane-V) of A. montana. (C) MDA-MB-231 cell invasion was examined by Transwell invasion assay in the presence of 0.01% DMSO alone (control) or 20 µg/mL of hexane subfraction V (hexane-V) of A. montana (scale bar is 50 µm). (D) MDA-MB-231 cell colony formation was detected following culturing with 30 µg/mL A. montana hexane subfraction V (hexane-V) of A. montana for 14 days (scale bar is 100 µm). (E) The expression of MMP-2 and MMP-9 was analyzed by western blotting t using A. montana hexane subfraction V (hexane-V) for 8 h. Then, equal amounts of lysates were separated by sodium dodecyl sulfate-polyacrylamide gel electrophoresis, blotted to a nitrocellulose membrane, and antibodies specific to MMP-2 and MMP-9 antibodies were used. Glyceraldehyde 3-phosphate dehydrogenase (GAPDH) was used as a loading control. Inset: the data are representative of one of four independent experiments. Western blotting was performed three times, and a representative image of the three independent experiments is shown. The results are expressed as the mean ± SEM of three independent experiments, and each was performed in triplicate. *p < 0.05 vs. DMSO-treated group (control). Hexane-V, A. montana hexane subfraction V; OD, optical density.
Anonna montana hexane subfraction V extract inhibits Ras signaling cascades
The Ras small GTPases control signaling pathways that are key regulators of several aspects of the uncontrol cell growth (Downward, 2003). The downstream of Ras proteins is the RAF/MEK/ERK kinase cascade (Kolch et al., 1991). Also, the active Ras turn on the PI3K/AKT/mTOR/S6K1 pathway regulates different cellular functions (i.e., anti-apoptotic pathways) (Yuan et al., 2020). We first investigated whether A. montana hexane sub-fraction V extract affects the PI3K/AKT/mTOR/S6K1 activation in MDA-MB-231 breast cancer cells. As shown in Figs. 7A–7D, PI3K and Akt activation was suppressed by 20 μg/mL of A. montana hexane subfraction V. In addition, the activation of S6K1 and mTOR was also decreased upon A. montana hexane extract treatment in MDA-MB-231 breast cancer cells. Our data indicate that inhibiting PI3K/AKT/mTOR/S6K1 signaling cascade by A. montana hexane sub-fraction V leads to the suppression of cell proliferation in MDA-MB-231 breast cancer cells. We next determine whether A. montana hexane subfraction V could alter the activation of MAPK cascades, including JNK, ERK1/2, and p38 MAPK in MDA-MB-231 breast cancer cells. As shown in Figs. 7E–7H, A. montana hexane subfraction V substantially decreased the phosphorylation of Raf-1 (84 ± 5% inhibition) and Erk1/2 (56 ± 3% inhibition) at a concentration of 20 μg/mL and slightly downregulated the phosphorylation of JNK (30 ± 4% inhibition) and p38 MAPK (19 ± 5% inhibition) in MDA-MB-231 breast cancer cells.
Figure 7: Annona montana hexane subfraction V suppressed PI3K/AKT/mTOR/S6K1 and MAPKs signaling pathways in breast cancer cells. (A–H) MDA-MB-231 cells were incubated with 0.01% DMSO or 20 μg/mL hexane subfraction of V (hexane-V) A. montana for 8 h as described in Material and Methods. Then, equal amounts of lysates were subset to sodium dodecyl sulfate-polyacrylamide gel electrophoresis, blotted to nitrocellulose, and antibodies specific to tyrosine-phosphorylated (p)-PI3K (Tyr458), PI3K, p-AKT (Ser473), AKT, p-mTOR (Ser2448), mTOR, p-S6K1 (Thr 421/Ser424), S6K1, p-ERK (Thr202/Tyr204), t-ERK, p-JNK (Thr183/Tyr185), JNK, p-p38 (Thr180/Tyr182), and p38. Relative levels of phosphorylated proteins were determined by densitometry as described in Material and Methods, and they were expressed as a ratio of phosphorylated/total proteins. The western blotting was performed three times, and a representative image of the three independent experiments is shown. Data represent the mean ± SEM of three independent experiments. *p < 0.05, **p < 0.01 by student’s t-test compared to non-treated cells.
Anonna montana hexane subfraction V extract inhibits the expression of essential signaling proteins involved in apoptosis, proliferation, and angiogenesis
Our observations indicate that AKT and ERK1/2 activities are affected by the A. montana hexane subfraction V. We next examined the effects of A. montana hexane subfraction V on the expression of survivin, IAP-1, and IAP-2 proteins. They have been implicated in apoptosis and act downstream to PI3K/Akt/mTOR/S6K1 signaling cascade (Hassan et al., 2013; Paplomata and O’Regan, 2014). We found that A. montana hexane subfraction V suppressed the expression of anti-apoptotic proteins (Figs. 8A–8C). Also, A. montana hexane sub-fraction V decreased the expression of Cyclin D1, COX-2, and VEGF (Figs. 8D–8F). In addition, we determined the effect of A. montana hexane sub-fraction V on the expression and cleaved procaspase-3 and PARP. Procaspase-3 is an excellent marker to monitor activated apoptotic and triggers cleavage of PARP, which is involved in repairing DNA damage (Kitazumi and Tsukahara, 2011). In Figs. 8G and 8H, we showed that A. montana hexane subfraction V induced the cleavage of procaspase-3 and PARP, as observed by the disappearance of the main band and the appearance of its cleaved forms. The tumor-suppressor proteins p53 and p21 are significant regulators of the cell–cycle checkpoint (Kulaberoglu et al., 2016). The p53 protein is a tumor suppressor in preventing cancer development (Luo et al., 1995). The cyclin-dependent kinase inhibitor p21 is regulated by p53 and negatively modulates cell cycle progression by inhibiting the activities of cyclin E/CDK2 and cyclin D/CDK4 complexes (Pandey et al., 2019). We found that A. montana hexane subfraction V induced the expression of both p53 and p21 in MDA-MB-231 breast cancer cells (Figs. 8I and 8J). These results demonstrate that A. montana hexane subfraction V induces apoptosis via the down-regulation of anti-apoptotic, proliferative, metastatic, and angiogenetic proteins via caspase-3 activation in MDA-MB-231 breast cancer cells.
Figure 8: Annona montana hexane subfraction V decreases the expression of proteins involved in proliferation, anti-apoptotic, metastatic, and angiogenetic proteins. (A–H) MDA-MB-231 cells were treated with 0.01% DMSO or 20 μg/mL hexane subfraction (hexane-V) of A. montana for 8 h. Thereafter, equal amounts of lysates were analyzed by western blot analysis using antibodies against survivin, IAP-1, IAP-2, Cyclin D1, COX-2, VEGF, procaspase-3, cleaved caspase-3, and PARP. (I, J) MDA-MB-231 cells were treated with 0.01% DMSO or 20 μg/mL hexane subfraction V of A. montana for 8 h. After, equal amounts of lysates were analyzed by western blotting analysis using antibodies against p21 and p53. Glyceraldehyde 3-phosphate dehydrogenase (GAPDH) was used as a loading control. Relative levels of each protein were determined by densitometry as described in the Material and Methods section, and they were expressed as a ratio of each protein/GAPDH. The data represents the mean ± SEM of three independent experiments. The western blots were performed three times, and a representative image of the three independent experiments is shown. *p < 0.05, and **p < 0.01.
Anonna montana hexane subfraction V extract potentiates the inhibition of pharmacological blockers
To further understand how A. montana hexane subfraction V may modulate various signaling molecules downstream of Ras pathways, we decided to treat MDA-MB-231 breast cancer cells with the following inhibitors (i.e., PF-3758309, FR180204, Wortmannin, and ISIS2503). We then investigated whether the A. montana hexane subfraction V was able to enhance its inhibitory effect by combination with the pharmacological inhibitors mentioned above. As shown in Figs. 9A–9D, treatment of cells with either A. montana hexane subfraction V or wortmannin inhibited AKT activation but had a weak inhibitory effect on mTOR and S6K1 activation. Interestingly, mTOR and S6K1 activation were blocked by combining A. montana hexane subfraction V alone or with wortmannin. In Figs. 9E–9G, we showed that the PF-3758309, a PAK1 inhibitor, decreased the phosphorylation of RAF. Moreover, it also affected the activation of ERK1/2. However, the addition of FR180204, an ERK1/2 inhibitor, strongly diminished the activation of ERK1/2 without affecting the activity of RAF. Interestingly, we also observed inhibition of the phosphorylation of RAF and ERK1/2 when A. montana hexane subfraction V was combined individually with each inhibitor. We also observed that the addition of A. montana hexane subfraction V was unable to affect the expression of Ras when compared with the ISIS2503 inhibitor alone in MDA-MB-231 breast cancer cells. As expected, the combination of A. montana hexane subfraction V with ISIS2503 inhibitor did not potentiate the inhibitory effect of the ISIS2503 inhibitor alone (Figs. 9H and 9I). We next examined the effects of A. montana hexane subfraction V extract on the activation of AKT and ERK1/2 activities upon IGF-1 stimulation. Fig. 10 shows that AKT and ERK1/2 activities were affected by the A. montana hexane subfraction V upon IGF-1 stimulation. However, neither IGF-1 receptor phosphorylation nor activation of Ras was affected. These data imply that A. montana hexane subfraction V can act downstream of the Ras protein through modulation of multiple signal transduction pathways to induce apoptosis in tumor cells.
Figure 9: Annona montana hexane subfraction potentiated the apoptotic effects of pharmacological PI3K/AKT blockers and Ras inhibitors on the blockage of the Ras/RAF/ERK signaling pathway in MDA-MB-231 cells. (A) MDA-MB-231 cells were treated with 0.01% DMSO (Control) and the indicated combination of hexane sub-fraction V (H, 20 μg/mL) or wortmannin (W, 0.233 μM) for 8 h. Then, equal amounts of lysates were analyzed by western blotting using antibodies against p-AKT (Ser473), t-AKT, p-mTOR (Ser2448), t-mTOR, p-S6K1 (Thr421/Ser424), and t-S6K1. The western blots were performed three times, and a representative image of the three independent experiments is shown. (B–D) Relative levels of phosphorylated proteins (i.e., p-AKT, p-mTOR, p-S6K1) were determined by densitometry as described in the Material and Methods section. They were expressed as a ratio of phosphorylated/total proteins. Data represent the mean ± SEM of three independent experiments. *p < 0.05, and **p < 0.01 by student’s t-test compared to non-treated cells. (E) MDA-MB-231 cells were treated with 0.01% dimethyl sulfoxide (control), and the indicated combination of hexane subfraction V (H, 20 µg/mL), PF3758309 (PF, 20 μM), or FR180204 (FR, 50 μM) for 8 h. Then equal amounts of lysates were analyzed by western blotting using antibodies against p-RAF (Ser259), t-RAF, p-ERK (Thr202/Tyr204), and t-ERK. The western blots were performed three times, and a representative image of the three independent experiments is shown. (F, G) Relative levels of phosphorylated proteins (i.e., p-RAF, p-ERK) were determined by densitometry as described in the Material and Methods section. They were expressed as a ratio of phosphorylated/total proteins. Data represent the mean ± SEM of three independent experiments. *p < 0.05 by student’s t-test compared to non-treated cells. (H) MDA-MB-231 cells were treated with the indicated combination of hexane subfraction V (H, 20 μg/mL) or Ras Inhibitor-ISIS 2503 (IS). Then, equal amounts of lysates were analyzed by western blotting using antibodies against Ras. Western blotting was performed three times, and a representative image of the three independent experiments is shown. Glyceraldehyde 3-phosphate dehydrogenase (GAPDH) was used as a loading control. (I) Relative levels of Ras proteins were determined by densitometry as described in the Material and Methods section, and they were expressed as a ratio of Ras/GAPDH proteins. Data represent the mean ± SEM of three independent experiments. *p < 0.05 by student’s t-test compared to non-treated cells.
Figure 10: Annona montana hexane sub-fraction V suppressed AKT and ERK activities signaling in human breast cancer cells without affecting Ras activation upon IGF-1 stimulation. MDA-MB-231 cells were serum-starved for 16 h and then incubated in the presence of 0.01% dimethyl sulfoxide or 20 μg/mL hexane subfraction V (H) of A. montana for 8 h. Subsequently, cells were incubated in the presence of 2 ng/mL IGF-1 at 4°C for 90 min, washed with cold HBSS-BSA and incubated for 3 min at 37°C as described in the Material and Methods section. (A–C) Equal amounts of lysates were subset to sodium dodecyl sulfate-polyacrylamide gel electrophoresis, blotted to nitrocellulose, and antibodies specific to tyrosine-phosphorylated (p)-AKT (Ser473), total (t)-AKT, p-ERK (Thr202/Tyr204), t-ERK, p-IGF-1R (Tyr1131), and t-IGF-1R. Relative levels of phosphorylated proteins were determined by densitometry as described in the Material and Methods section, and they were expressed as a ratio of phosphorylated/total proteins. (D) After incubation, cells were washed with cold HBSS-BSA, lysed, and allowed to bind to glutathione beads in the presence of GST-RBD at 4°C for 60 min. Beads were washed with cold HBSS-BSA, and the presence of activated Ras (i.e., GTP-Ras) was analyzed by western blotting. Added GST-RBD and t-Ras proteins in the cell lysate were also evaluated by western blotting analysis. The Data represent the mean ± SEM of three independent experiments. *p < 0.05 by student’s t-test compared to non-treated cells.
Chemical composition of the Anonna montana hexane subfraction V extract
Chemical studies with species of the Annona genus have reported on the essential oils from fruits because many fruits from the Annonaceae family are edible (Nugraha et al., 2019; Al Kazman et al., 2022). On the contrary, only some studies have been performed on the leaves. Thus, we conducted a GC-MS analysis as described in Material and Methods section to gain more information about the composition of the constituents, specifically in the A. montana hexane sub-fraction V. Our results on A. montana hexane sub-fraction V indicated that ten compounds out of a total of fifteen compounds were identified, (Table 4 and Suppl. Table S1) in a complex mixture of phytosterols (i.e., campesterol, γ-sitosterol, β-sitosterol, and γ-stigmasterol), γ-tocopherol, 1,2-epxyoctadecane, methyl-elaidolinolenate, 2-clhloroethyllinoleate, solanesol, and palmitic acid. Interestingly, some of these compounds have been reported to affect cell proliferation and apoptosis cellular mechanisms in cancer cells (Awad and Fink, 2000; Nugraha et al., 2019; Al Kazman et al., 2022; Cioccoloni et al., 2022).
Natural products from a variety of sources have been investigated to target several diseases, including cancer, for many years due to their biological activities and potential medicinal values contained in them. In this study, the anti-proliferative effect of the hexane leaf extract of A. montana was evaluated on breast cancer cell lines and other cancer cell lines with several methodologies. This hexane extract (i.e., A. montana hexane sub-fraction V) was found to contain a variety of bioactive compounds, including Campesterol, γ-Sitosterol, β-Sitosterol, and γ-Stigmasterol, which could explain the efficient and selective anti-breast cancer activity against triple-negative breast cancer cells (e.g., MDA-MB-231) and other cancer cell lines. Consistent with earlier findings (Leung et al., 2022), A. montana extracts inhibited the differentiation of both mouse and human pre-adipocytes without any toxic effect by blocking the early steps of the differentiation process. Based on the anti-proliferative profile obtained, the hexane leaf sub-fraction V of A. montana was more selective towards MCF-7 than the MDA-MB-231 cell line. The proliferation of the HeLa cell line was also inhibited by adding A. montana hexane sub-fraction V. However, the treatment with the same subfraction affected the proliferation of A459 and Sk-MEL-28 less (Table 2). The anti-proliferative effect of primary mammalian epithelial cells and MCF-10A cell line in the presence of A. montana hexane extract was determined. It appeared that adding A. montana hexane extract was significantly less effective for normal cells as it required a higher dosage to kill cancer cells, suggesting the low side effect of this A. montana hexane extract. Together, these results indicate the selective effect of A. montana hexane extract in affecting cancerous cells with no damage to normal healthy cells. Several investigations on other related Annona species, like A. muricata and A. squamosa, have concentrated on their anti-proliferative effect against different cancer cell lines (Wang et al., 2014; Syed Najmuddin et al., 2016). Other studies revealed the anti-proliferative effect of A. cherimola leaves and seeds on cancer and leukemic cells (Arunjyothi et al., 2012; Suresh et al., 2011; Haykal et al., 2019). Recent studies revealed the anti-proliferative effect of A. montana fruit on several cancer cell lines (Bailon-Moscoso et al., 2016). Here, we observed that the hexane subfraction V of A. montana exerted significant anti-proliferative and apoptotic effects and suppressed the PI3K/AKT/mTOR/S6K1 activation and in less extent, the MAPKs activation in human breast cancer cells. Consequently, this A. montana hexane sub-fraction V down-regulated various gene products involved in cell migration, proliferation, apoptosis, and invasion. Thus, the treatment of cancer cells with A. montana hexane sub-fraction V targeted more selectively the PI3K/AKT/mTOR/S6K1 more than the MAPK signaling pathways and, more critical, induced apoptosis through caspase-3 activation. We found that A. montana hexane subfraction V blocked proliferation and the expression of various genes involved in the cell cycle. The cyclin D1, as well as p70S6K, are required for cell growth and the cell cycle progression in tumor cells (Mita et al., 2003). In addition, we also found that the expression of p53 and p21 tumor suppressors were upregulated upon Am hexane sub-fraction V treatment. These tumor suppressors can regulate the activities of cyclin-dependent kinase complexes, were also upregulated upon A. montana hexane sub-fraction V treatment. We also observed that A. montana hexane sub-fraction V could block surviving expression, suggesting that the use of A. montana hexane sub-fraction V can lead to the development of a novel strategy to regulate the stimulatory effect of IGF-1 on the proliferation of breast cancer cells (Costa-Silva et al., 2020). Interestingly, we also found that either the expression and activation of IGF-1R and Ras were not affected, suggesting a selective effect of A. montana hexane sub-fraction V on these signaling pathways. Furthermore, we found that this A. montana hexane sub-fraction V can also significantly potentiate the apoptotic effects of specific AKT inhibitors. This potentiation is likely mediated by suppressing anti-apoptosis genes regulated by the PI3K/mTOR pathways. Our results also indicate, for the first time, that A. montana hexane sub-fraction V suppressed constitutive mTOR (Ser2448) activation in breast cancer cells. Recently, several mTOR inhibitors have shown great promise in clinical trials for treating various malignant tumors, where activation of mTOR is closely associated with tumorigenesis (Gentric et al., 2017; Ciolczyk-Wierzbicka et al., 2020; Xu et al., 2020; Popova and Jucker, 2021). Thus, it is possible that targeting the mTOR signaling pathway with a variety of compounds from natural sources can reveal a number of potential candidates for the prevention and treatment of cancer. Consistent with this observation, we also found that A. montana hexane sub-fraction V blocked the expression of MMP-1 and MMP-9, vital tissue remodeling enzymes with multiple overlapping activities critical for wound healing, invasion, migration, and tumor progression in vivo (Hingorani et al., 2018). We additionally discovered that the expression of various genes involved in tumor initiation and promotion was also repressed by A. montana hexane sub-fraction V. These include proliferative (cyclin D1), anti-apoptotic (survivin, IAP-1, and IAP-2), metastatic (COX-2), and angiogenic (VEGF) gene products. AKT signaling through mTOR and MAPK activation in tumor cells are essential mechanisms of oncogenesis that can shield cancer cells from apoptosis and drug resistance in vivo (Populo et al., 2012). Thus, suppressing COX-2 and VEGF may be the potential link for inhibiting metastasis, angiogenesis, and invasion by A. montana hexane sub-fraction V.
Our results indicate for the first time that A. montana hexane sub-fraction V can block constitutive PI3K/mTOR signaling transduction pathways stimulate MAPKs activation, and lead to apoptosis induction by decreasing gene expression, facilitating tumor cell survival, metastasis, and angiogenesis in human breast cancer cells. This selective effect on signaling transduction pathways of the A. montana hexane sub-fraction V could be attributed to one or more compounds identified in this subfraction. Nevertheless, a further chemical and cellular evaluation of A. montana hexane sub-fraction V is needed to learn about its anti-proliferative mechanism.
Acknowledgement: We thank the members of our laboratory for providing generous assistance.
Funding Statement: This work was partially supported by the National Institutes of Health Grant SC1DK084343 (to MAB) and by Mass Spectrometry Research and Education Center must cite funding from NIH S10 OD021758-01A1. This study was partially supported by intramural funds from the Dean’s Office (College of Arts and Sciences and Education).
Author Contributions: The authors confirm their contribution to the paper: study conception and design: MAB, MLV; data collection: MA, SS, LA, WZ, LA, IL; analysis and interpretation of results: WZ, IL, LA; draft manuscript preparation: MLV, MAB, RB. All authors reviewed the results and approved the final version of the manuscript.
Availability of Data and Materials: The datasets generated during and/or analyzed during the current study are available from the corresponding author upon reasonable request.
Ethics Approval: Not applicable.
Conflicts of Interest: The authors declare no conflicts of interest to report regarding the present study.
References
Adams RP (2007). Identification of Essential Oil Components by Gas Chromatography/Mass Spectrometry, 4th Edition. Carol Stream, IL: Allured Publishing Corporation. [Google Scholar]
Adeyemi DO, Komolafe OA, Adewole OS, Obuotor EM, Adenowo TK (2008). Anti hyperglycemic activities of Annona muricata (Linn). African Journal of Traditional, Complementary and Alternative Medicines 6: 62–69. [Google Scholar]
Al Kazman BSM, Harnett JE, Hanrahan JR (2022). Traditional uses, phytochemistry and pharmacological activities of annonacae. Molecules 27: 3462. [Google Scholar] [PubMed]
Alvarez Colom O, Neske A, Chahboune N, Zafra-Polo MC, Bardon A (2009). Tucupentol, a novel mono-tetrahydrofuranic acetogenin from Annona montana, as a potent inhibitor of mitochondrial complex I. Chemistry Biodiversity 6: 335–340. [Google Scholar] [PubMed]
Alvarez DF, Helm K, Degregori J, Roederer M, Majka S (2010). Publishing flow cytometry data. American Journal of Physiology-Lung Cellular and Molecular Physiology 298: L127-30. [Google Scholar] [PubMed]
Araujo HC, Lacerda ME, Lopes D, Bizzo HR, Kaplan MA (2007). Studies on the aroma of mate (Ilex paraguariensis St. Hil.) using headspace solid-phase microextraction. Phytochemical Analysis 18: 469–474. [Google Scholar] [PubMed]
Arunjyothi B, Venkatesh K, Chakrapani P, Anupalli R (2012). Phytochemical and pharmacological potential of Annona cherimola–A review. International Journal of Phytomedicine 3: 439–447. [Google Scholar]
AsghariHanjani N, Vafa M (2019). The role of IGF-1 in obesity, cardiovascular disease, and cancer. Medical Journal of the Islamic Republic of Iran 33: 56. [Google Scholar] [PubMed]
Awad AB, Fink CS (2000). Phytosterols as anticancer dietary components: Evidence and mechanism of action. The Journal of Nutrition 130: 2127–2130. [Google Scholar] [PubMed]
Babushok V, Linstrom P, Zenkevich I (2011). Retention indices for frequently reported compounds of plant essential oils. Journal of Physical and Chemical Reference Data 40: 043101-043101-47. [Google Scholar]
Bailon-Moscoso N, Romero Benavides JC, Ramirez Orellana MIA, Ojeda K, Granda G, Ratoviski EA, Ostrosky-Wegman P (2016). Cytotoxic and genotoxic effects of extractsfrom Annona montana M. fruit. Food and Agricultural Immunology 27: 559–569. [Google Scholar]
Borowicz S, Van Scoyk M, Avasarala S, Karuppusamy Rathinam MK, Tauler J, Bikkavilli RK, Winn RA (2014). The soft agar colony formation assay. Journal of Visualized Experiments 92: 51998. [Google Scholar]
Brown GT, Murray GI (2015). Current mechanistic insights into the roles of matrix metalloproteinases in tumour invasion and metastasis. Journal of Pathology 237: 273–281. [Google Scholar] [PubMed]
Camalier CE, Young MR, Bobe G, Perella CM, Colburn NH, Beck Jr GR (2010). Elevated phosphate activates N-ras and promotes cell transformation and skin tumorigenesis. Cancer Prevention Research 3: 359–370. [Google Scholar] [PubMed]
Cappiello F, Casciaro B, Mangoni ML (2018). A novel in vitro wound healing assay to evaluate cell migration. Journal of Visualized Experiments 133: 56825. [Google Scholar]
Chang FR, Wu YC (2001). Novel cytotoxic annonaceous acetogenins from Annona muricata. Journal of Natural Products 64: 925–931. [Google Scholar] [PubMed]
Chen G, Oh S, Monia BP, Stacey DW (1996). Antisense oligonucleotides demonstrate a dominant role of c-Ki-RAS proteins in regulating the proliferation of diploid human fibroblasts. Journal of Biological Chemistry 271: 28259–28265. [Google Scholar] [PubMed]
Chin C, Veisaga ML, Priestap H, Commock T, Campbell K, Jestrow B, Francisco-Ortega J, Barbieri M (2014). Antiproliferative effect of portlandia extracts and their fractions on breast cancer cells. European Journal of Medicinal Plants 4: 1186–1199. [Google Scholar]
Chuang PH, Hsieh PW, Yang YL, Hua KF, Chang FR, Shiea J, Wu SH, Wu YC (2008). Cyclopeptides with anti-inflammatory activity from seeds of Annona montana. Journal of Natural Products 71: 1365–1370. [Google Scholar] [PubMed]
Cioccoloni G, Soteriou C, Websdale A, Wallis L, Zulyniak MA, Thorne JL (2022). Phytosterols and phytostanols and the hallmarks of cancer in model organisms: A systematic review and meta-analysis. Critical Reviews in Food Science and Nutrition 62: 1145–1165. [Google Scholar] [PubMed]
Ciolczyk-Wierzbicka D, Gil D, Zarzycka M, Laidler P (2020). mTOR inhibitor everolimus reduces invasiveness of melanoma cells. Human Cell 33: 88–97. [Google Scholar] [PubMed]
Costa-Silva DR, Barros-Oliveira MDC, Alves-Ribeiro FA, Campos-Verdes LC, Nery Junior EJ et al. (2020). Assessment of IGF-1 expression in the peripheral blood of women with recurrent breast cancer. Medicine 99: e22890. [Google Scholar] [PubMed]
de Felice F, Megiorni F, Pietrantoni I, Tini P, Lessiani G et al. (2019). Sulodexide counteracts endothelial dysfunction induced by metabolic or non-metabolic stresses through activation of the autophagic program. European Review for Medical and Pharmacological Sciences 23: 2669–2680. [Google Scholar] [PubMed]
Downward J (2003). Targeting RAS signalling pathways in cancer therapy. Nature Reviews Cancer 3: 11–22. [Google Scholar] [PubMed]
Elmore SA, Dixon D, Hailey JR, Harada T, Herbert RA et al. (2016). Recommendations from the INHAND apoptosis/necrosis working group. Toxicologic Pathology 44: 173–188. [Google Scholar] [PubMed]
Fares J, Fares MY, Khachfe HH, Salhab HA, Fares Y (2020). Molecular principles of metastasis: A hallmark of cancer revisited. Signal Transduction and Targeted Therapy 5: 28. [Google Scholar] [PubMed]
Folgueras AR, Pendas AM, Sanchez LM, Lopez-Otin C (2004). Matrix metalloproteinases in cancer: From new functions to improved inhibition strategies. International Journal of Developmental Biology 48: 411–424. [Google Scholar] [PubMed]
Formagio AS, Vieira MC, Volobuff CR, Silva MS, Matos AI, Cardoso CA, Foglio MA, Carvalho JE (2015). In vitro biological screening of the anticholinesterase and antiproliferative activities of medicinal plants belonging to Annonaceae. The Brazilian Journal of Medical and Biological Research 48: 308–315. [Google Scholar] [PubMed]
Gentric G, Mieulet V, Mechta-Grigoriou F (2017). Heterogeneity in cancer metabolism: New concepts in an old field. Antioxidants & Redox Signaling 26: 462–485. [Google Scholar]
Gupta RK, Kesari AN, Murthy PS, Chandra R, Tandon V, Watal G (2005). Hypoglycemic and antidiabetic effect of ethanolic extract of leaves of Annona squamosa L. in experimental animals. Journal of Ethnopharmacology 99: 75–81. [Google Scholar] [PubMed]
Hassan B, Akcakanat A, Holder AM, Meric-Bernstam F (2013). Targeting the PI3-kinase/Akt/mTOR signaling pathway. Surgical Oncology Clinics of North America 22: 641–664. [Google Scholar] [PubMed]
Haykal T, Nasr P, Hodroj MH, Taleb RI, Sarkis R, Moujabber MNE, Rizk S (2019). Annona cherimola seed extract activates extrinsic and intrinsic apoptotic pathways in leukemic cells. Toxins 11: 506. [Google Scholar] [PubMed]
Hingorani DV, Lippert CN, Crisp JL, Savariar EN, Hasselmann JPC, Kuo C, Nguyen QT, Tsien RY, Whitney MA, Ellies LG (2018). Impact of MMP-2 and MMP-9 enzyme activity on wound healing, tumor growth and RACPP cleavage. PLoS One 13: e0198464. [Google Scholar] [PubMed]
Huang M, Lu JJ, Ding J (2021). Natural products in cancer therapy: Past, present and future. Natural Products and Bioprospectingt 11: 5–13. [Google Scholar] [PubMed]
Hulkower KI, Herber RL (2011). Cell migration and invasion assays as tools for drug discovery. Pharmaceutics 3: 107–124. [Google Scholar] [PubMed]
Kaleem M, Asif M, Ahmed QU, Bano B (2006). Antidiabetic and antioxidant activity of Annona squamosa extract in streptozotocin-induced diabetic rats. Singapore Medical Journal 47: 670–675. [Google Scholar] [PubMed]
Kitazumi I, Tsukahara M (2011). Regulation of DNA fragmentation: The role of caspases and phosphorylation. FEBS Journal 278: 427–441. [Google Scholar] [PubMed]
Kolch W, Heidecker G, Lloyd P, Rapp UR (1991). Raf-1 protein kinase is required for growth of induced NIH/3T3 cells. Nature 349: 426–428. [Google Scholar] [PubMed]
Kulaberoglu Y, Gundogdu R, Hergovich A (2016). Chapter the role of p53/p21/p16 in DNA-damage signaling and DNA repair, 243–256. https://doi.org/10.1016/B978-0-12-803309-8.00015-X [Google Scholar] [CrossRef]
Kumar AS, Venkatarathanamma V, Saibabu VN (2015). Phytochemical and phytotherapeutic properties of Annona squamosa, Annona reticulata and Annona muricata: A review. Asian Journal of Plant Science & Research 5: 28–33. [Google Scholar]
Lederer AK, Huber R (2022). The relation of diet and health: You are what you eat. International Journal of Environmental Research and Public Health 19: 7774. [Google Scholar] [PubMed]
Leung I, Veisaga ML, Espinal M, Zhang W, Barnum R, Barbieri MA (2022). Anti-lipid droplets accumulation effect of Annona montana (mountain soursop) leaves extract on differentiation of preadipocytes. BIOCELL 46: 567–578. https://doi.org/10.32604/biocell.2022.014009 [Google Scholar] [PubMed] [CrossRef]
Lim TK (2012). Cydonia oblonga. In: Edible Medicinal and Non-Medicinal Plants: Fruits, vol. 4, pp. 371–380. The Netherlands: Springer. https://doi.org/10.1007/978-94-007-4053-2_45 [Google Scholar] [CrossRef]
Luo Y, Hurwitz J, Massague J (1995). Cell-cycle inhibition by independent CDK and PCNA binding domains in p21Cip1. Nature 375: 159–161. [Google Scholar] [PubMed]
Mita MM, Mita A, Rowinsky EK (2003). Mammalian target of rapamycin: A new molecular target for breast cancer. Clinical Breast Cancer 4: 126–137. [Google Scholar] [PubMed]
Mosmann T (1983). Rapid colorimetric assay for cellular growth and survival: Application to proliferation and cytotoxicity assays. Journal of Immunological Methods 65: 55–63. [Google Scholar] [PubMed]
Nugraha AS, Damayanti YD, Wangchuk P, Keller PA (2019). Anti-infective and anti-cancer properties of the annona species: Their ethnomedicinal uses, alkaloid diversity, and pharmacological activities. Molecules 24: 4419. https://doi.org/10.3390/molecules24234419 [Google Scholar] [PubMed] [CrossRef]
Pandey K, An HJ, Kim SK, Lee SA, Kim S, Lim SM, Kim GM, Sohn J, Moon YW (2019). Molecular mechanisms of resistance to CDK4/6 inhibitors in breast cancer: A review. International Journal of Cancer 145: 1179–1188. [Google Scholar] [PubMed]
Paplomata E, O’Regan R (2014). The PI3K/AKT/mTOR pathway in breast cancer: Targets, trials and biomarkers. Therapeutic Advances in Medical Oncologyl 6: 154–166. [Google Scholar]
Pardhasaradhi BV, Reddy M, Ali AM, Kumari AL, Khar A (2005). Differential cytotoxic effects of Annona squamosa seed extracts on human tumour cell lines: Role of reactive oxygen species and glutathione. Journal of Biosciences 30: 237–244. [Google Scholar] [PubMed]
Popova NV, Jucker M (2021). The role of mTOR signaling as a therapeutic target in cancer. International Journal of Molecular 22: 1743. [Google Scholar]
Populo H, Lopes JM, Soares P (2012). The mTOR signalling pathway in human cancer. International Journal of Molecular 13: 1886–1918. [Google Scholar]
Pratheeshkumar P, Sreekala C, Zhang Z, Budhraja A, Ding S et al. (2012). Cancer prevention with promising natural products: Mechanisms of action and molecular targets. Anti-Cancer Agents in Medicinal Chemistry 12: 1159–1184. [Google Scholar] [PubMed]
Priestap H, Veisaga M, Commockm T, Campbell K, Jestrow B, Francisco-Ortega J, Barbieri M (2014). Volatile constituents of five species of Portlandia (Rubiaceae). Journal of Essential Oil Research 26: 125–129. [Google Scholar]
Ribeiro LR, Santos MF, Silva QM, Palmieri MJ, Larissa F, Andrade-Vieira LF, Davide LC (2013). Cytogenotoxic effects of ethanolic extracts of Annona crassiflora (Annonaceae). Biologia 68: 433–438. [Google Scholar]
Rupprecht JK, Hui YH, McLaughlin JL (1990). Annonaceous acetogenins: A review. Journal Natural Products 53: 237–278. [Google Scholar]
Sharifi-Rad J, Ozleyen A, Boyunegmez Tumer T, Oluwaseun Adetunji C, El Omari N et al. (2019). Natural products and synthetic analogs as a source of antitumor drugs. Biomolecules 9: 679. [Google Scholar] [PubMed]
Sharma P, Khan MA, Najmi AK, Chaturvedi S, Akhtar M (2022). Myricetin-induced apoptosis in triple-negative breast cancer cells through inhibition of the PI3K/Akt/mTOR pathway. Medical Oncology 39: 248. [Google Scholar] [PubMed]
Smith SM, Wunder MB, Norris DA, Shellman YG (2011). A simple protocol for using a LDH-based cytotoxicity assay to assess the effects of death and growth inhibition at the same time. PLoS One 6: e26908. [Google Scholar] [PubMed]
Suresh HM, Shivakumar B, Hemalatha K, Heroor SS, Hugar DS, Rao KR (2011). In vitro antiproliferativeactivity of Annona reticulata roots on human cancer cell lines. Pharmacognosy Research 3: 9–12. [Google Scholar] [PubMed]
Syed Najmuddin SUF, Romli MF, Hamid M, Alitheen NB, Nik Abd Rahman NM (2016). Anti-cancer effect of Annona Muricata Linn Leaves Crude Extract (AMCE) on breast cancer cell line. BMC Complementary Medicine and Therapies 16: 311. [Google Scholar]
Tall GG, Barbieri MA, Stahl PD, Horazdovsky BF (2001). Ras-activated endocytosis is mediated by the Rab5 guanine nucleotide exchange activity of RIN1. Developmental Cell 1: 73–82. [Google Scholar] [PubMed]
Tundis R, Xiao J, Loizzo MR (2017). Annona species (AnnonaceaeA rich source of potential antitumor agents? Annals of the New York Academy of Sciences 1398: 30–36. [Google Scholar] [PubMed]
Wang LQ, Li Y, Min BS, Nakamura N, Qin GW, Li CJ, Hattori M (2001). Cytotoxic mono-tetrahydrofuran ring acetogenins from leaves of Annona montana. Planta Medica 67: 847–852. [Google Scholar] [PubMed]
Wang DS, Rizwani GH, Guo H, Ahmed M, Ahmed M, Hassan SZ, Hassan A, Chen ZS, Xu RH (2014). Annona squamosa Linn: Cytotoxic activity found in leaf extract against human tumor cell lines. Pakistan Journal of Pharmaceutical Sciences 27: 1559–1563. [Google Scholar] [PubMed]
WHO (2019). WHO Global Report on Traditional and Complementary Medicine. Geneva: World Health Organization. Licence: CC BY-NC-SA 3.0 IGO. 2019. [Google Scholar]
Wu YC, Chang GY, Ko FN, Teng CM (1995). Bioactive constitutents from the stems of Annona montana. Planta Medica 61: 146–149. [Google Scholar] [PubMed]
Xu Z, Han X, Ou D, Liu T, Li Z, Jiang G, Liu J, Zhang J (2020). Targeting PI3K/AKT/mTOR-mediated autophagy for tumor therapy. Applied Microbiology and Biotechnology 104: 575–587. [Google Scholar] [PubMed]
Yan J, Huang JH, He M, Lu HB, Yang R, Kong B, Xu QS, Liang YZ (2013). Prediction of retention indices for frequently reported compounds of plant essential oils using multiple linear regression, partial least squares, and support vector machine. Journal of Separation Science 36: 2464–2471. [Google Scholar] [PubMed]
Yang Z, Zhang Q, Yu L, Zhu J, Cao Y, Gao X (2021). The signaling pathways and targets of traditional Chinese medicine and natural medicine in triple-negative breast cancer. Journal of Ethnopharmacology 264: 113249. [Google Scholar] [PubMed]
Yuan J, Dong X, Yap J, Hu J (2020). The MAPK and AMPK signalings: Interplay and implication in targeted cancer therapy. Journal of Hematology & Oncology 13: 113. [Google Scholar]
Zhang Z, Richmond A, Yan C (2022). Immunomodulatory properties of PI3K/AKT/mTOR and MAPK/MEK/ERK inhibition augment response to immune checkpoint blockade in melanoma and triple-negative breast cancer. International Journal of Molecular Sciences 23: 7353. [Google Scholar] [PubMed]
Supplementary Materials
Cite This Article
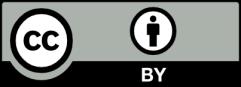
This work is licensed under a Creative Commons Attribution 4.0 International License , which permits unrestricted use, distribution, and reproduction in any medium, provided the original work is properly cited.