Open Access
REVIEW
Microfluidic platform for circulating tumor cells isolation and detection
1 School of Mechanical Engineering, Hebei University of Technology, Tianjin, 300401, China
2 State Key Lab of Reliability and Intelligence of Electrical Equipment, Tianjin, 300401, China
3 National Engineering Research Center for Technological Innovation Method and Tool, Tianjin, 300401, China
4 College of Biological, Chemical Sciences and Engineering, Jiaxing University, Jiaxing, 314001, China
* Corresponding Authors: ZIRUI LI. Email: ; YIXING GOU. Email:
BIOCELL 2023, 47(7), 1439-1447. https://doi.org/10.32604/biocell.2023.028628
Received 29 December 2022; Accepted 20 March 2023; Issue published 21 June 2023
Abstract
Circulating tumor cells (CTCs) are essential biomarkers for liquid biopsies, which are important in the early screening, prognosis, and real-time monitoring of cancer. However, CTCs are less abundant in the peripheral blood of patients, therefore, their isolation is necessary. Recently, the use of microfluidics for CTC sorting has become a research hotspot owing to its low cost, ease of integration, low sample consumption, and unique advantages in the manipulation of micron-sized particles. Herein, we review the latest research on microfluidics-based CTC sorting. Specifically, we consider active sorting using external fields (electric, magnetic, acoustic, and optical tweezers) and passive sorting using the flow effects of cells in specific channel structures (microfiltration sorting, deterministic lateral displacement sorting, and inertial sorting). The advantages and limitations of each method and their recent applications are summarized here. To conclude, a forward-looking perspective is presented on future research on the microfluidic sorting of CTCs.Keywords
Tumor metastasis causes approximately 90% of cancer-related deaths (Dillekas et al., 2019); therefore, detecting the metastatic process in real-time is crucial. In contrast to invasive biopsy techniques, which have been found previously to cause pain to patients (Hirahata et al., 2022), liquid biopsy techniques that are minimally invasive and enable real-time detection have recently gained increased attention (Belotti and Lim, 2021; Cortes-Hernandez et al., 2020; de Rubis et al., 2019). For cancer diagnosis and treatment, early screening, and prognostic analysis, liquid biopsy is performed by extracting effective components, such as circulating tumor cells (CTCs) (Alix-Panabières and Pantel, 2016), circulating tumor DNA (ctDNA) (Gorgannezhad et al., 2018), and exosomes (Contreras-Naranjo et al., 2017), which are present in human body fluids. Among these, CTC and ctDNA are approved by the Food and Drug Administration (FDA) to be utilized as important biomarkers in clinical diagnosis, which has become an important milestone in clinical trials of liquid biopsies (Alix-Panabieres and Pantel, 2021).
CTCs are cells shed by a tumor lesion (primary or metastatic lesion); they break through the tissue matrix and eventually enter the bloodstream (Nowell, 1976). Compared with ctDNA, which is a free gene fragment, CTCs can express the entire genetic information of a tumor from genome to functional protein, thereby making it a hot research topic in the field of liquid biopsy (Alix-Panabieres and Pantel, 2013). Compared with traditional clinical imaging tests, CTC testing has broad application prospects in clinical practice (de Wit et al., 2018), such as assisting in clinical diagnosis and cancer staging, reflecting early disease status of the patients for determining patient prognosis (Shaw et al., 2017), detecting treatment effects in real-time, and providing individualized medical treatment (D’Avola et al., 2018). Meanwhile, the RNA sequencing of CTCs can reveal the biological significance of CTCs in the metastatic process (Baccelli et al., 2013).
Despite the importance of CTCs in cancer diagnosis and detection, the number of CTCs in peripheral blood is extremely low, with only 1–10 CTCs/mL in early-stage cancer patients and a few hundred CTCs/mL in advanced-stage patients, compared with the billions of blood cells/mL (Kahn et al., 2004), hence, the efficient enrichment of CTCs in peripheral blood is essential. Early physical crude separation techniques were based on the separation of CTCs by special membrane devices and density gradient centrifugation. Among theseThe typical application is the isolation by the size of epithelial tumor cells (ISET system) developed by Rarecells Diagnostics (Paris, France) (Farace et al., 2011). To improve the purity of CTC enrichment, many pharmaceutical companies have focused on using immunomagnetic beads. For example, Johnson & Johnson (New Jersey, USA) launched the FDA-approved CellSearch system (Rushton et al., 2021). However, this product was discontinued in early 2016 because of its low detection sensitivity and inability to isolate live CTCs. Apart from the CellSearch system, the AdnaTest by Qiagen (Todenhofer et al., 2012), the MACS developed by Miltenyi (Voena et al., 2002), and the MagSweeper system conducted by Illumina (Cann et al., 2012), were also conducted in the past few years. These commercial sorting methods based on immunomagnetic beads are highly specific and highly integrated but still limited in clinical applications due to the high cost of the instruments and supporting consumables and missed inspection. Therefore, miniaturized, low-cost, and efficient sorting systems remain a common focus of scientific and industrial interest.
Microfluidics has rapidly evolved in recent decades from molecular analysis to cell biology because of their ability to control the mechanical, biological, and fluidic environment at the molecular and cellular levels (Autebert et al., 2012). Cell sorting on microchips offers many advantages over conventional methods, as it reduces the size of the required equipment, eliminates potentially biohazardous procedures, and simplifies the complex protocols typically associated with cell sorting (Bhat et al., 2022). In addition, microchip devices are ideally suited for parallelization, allowing complete lab-on-a-chip equipment to be used for cell isolation, analysis, and experimental processing (Shields et al., 2015). Microfluidic technology offers many advantages over conventional non-microfluidic devices, including portability, improved sensitivity, lower operating costs, and higher throughput, making this technology promising in CTC sorting applications. This review focuses on the recent advances and the advantages and disadvantages of two types of microfluidic chip-based CTC sorting: active and passive sorting. The latest methods proposed in the same category (active sorting or passive sorting) are similar in many aspects, so this classification strategy could provide more information and guidance on chip manufacturing (whether to integrate with additional devices), sorting mechanism (whether to involve additional forces), sorting indicators (recovery, purity, throughput, etc.), which could facilitate subsequent scheme comparison and methods selection based on the actual situation.
According to the different cell manipulation methods by microfluidic chips, CTC sorting based on microfluidic platforms can be divided into active and passive sorting techniques (Fig. 1).
Figure 1: Summary of CTC isolation method including active sorting and passive sorting, where active sorting includes electric field sorting, magnetic field sorting, acoustic field sorting, and optical tweezers sorting. Passive sorting includes microfiltration, deterministic lateral displacement, and inertial sorting.
Active sorting involves the manipulation of different cells by external field sources (e.g., electric, magnetic, optical, and acoustic fields) to sort CTCs from blood cells. Passive sorting involves using various migration trajectories of different cells based on the hydrodynamic properties of the flow channel with no interference from any external field.
Among the active sorting methods, electrical sorting is currently the most widely used particle control method, primarily in the form of electro-permeation, electrophoresis, and dielectrophoresis (Hajba and Guttman, 2014; Semaan et al., 2021). Electrical sorting is based on the dielectric properties of CTCs (Fig. 2a). Jahangiri et al. (2020) introduced a label-free cytological slide chip (CSC) based on the AC electric field stimulation of breast cell lines and blood cells at low frequencies (1–200 kHz). AC-CSC can be used to separate CTCs from leukocytes (1% MDA-MB-231:99% white blood cells (WBC)) with a capture efficiency of 90%. Arslan et al. (2022) proposed a continuous-flow, antibody-free, dielectrophoresis-based microfluidic device to separate CTCs from blood cells. CTC recoveries ranged from 74% to 98% at a frequency of 1 MHz and an amplitude of 10–12 Vpp. The main commercial cell sorting systems based on electrophoresis technology are the DEPArray system (Medoro, 2003) (Menarini Silicon Biosystems, Castel Maggiore (BO), Italy) and ApoStream system (Gupta et al., 2012) (ApoCell, Houston, TX, USA). Although such systems could achieve a high sorting accuracy, the additional electrodes and other equipment increase the cost of microfluidic chip fabrication and affect cell activity owing to problems such as cell electroporation caused by the electric field.
Figure 2: Schematic diagram of the microfluidic device for separating circulating tumor cells (CTC) in active sorting. (a) Schematic diagram of the dielectrophoresis apparatus used to separate CTCs (Gupta et al., 2012); (b) Schematic representation of label-free isolation of HeLa cells in a ferromagnetic fluid by magnetic buoyancy under a magnetic field (Zhao et al., 2016); (c) Schematic diagram of the integrated acoustic-fluidic device for the separation of CTC (Wu et al., 2017); (d) Schematic diagram of the optical separation device used to separate CTCs (Hu et al., 2019).
Magnetic sorting is primarily based on the immunological properties of cells (Fig. 2b). It involves binding magnetic beads coupled with specific immunological markers to the target cells and their separation from unlabeled nontarget cells through the magnetic field generated by a permanent magnet or electromagnet (Bakhshi et al., 2021; Ghafouri and Badieirostami, 2021). Depending on the coupling target, magnetic sorting can be positive sorting, which directly captures tumor cells, and negative sorting, which captures WBC. Wang et al. (2021) developed an immunomagnetic bead made of triiron tetroxide and calcium carbonate, which enabled the complete sorting of all subpopulations of tumor cells. Kim et al. (2013) proposed a CTC separator using immunomagnetic beads bound to the CTC, separated by transverse magnetic electrophoresis to purify 90% of the spiked CTC to 97% at a flow rate of 5 mL/h. Mishra et al. (2020) proposed an ultra-high-throughput microfluidic chip, the LPCTC-iChip, which rapidly separates leukocytes from over six billion nucleated cells. This increased the CTC separation capacity by two orders of magnitude, with a recovery rate of 86% at an enrichment of 105. During the capture process, the nonspecific interaction between magnetic beads and leukocytes worsens the purity problem. To address this problem, Zhu et al. (2018) proposed the coating of cancer-targeting molecules, such as folic acid and magnetic beads on red blood cells, that would adhere to CTCs to obtain CTC-RBCs. Subsequently, red blood cells could be lysed to obtain CTCs. The CTC purity was >75%. Shi et al. (2017) used a microfluidic device with a wavy herringbone structure to separate CTCs using magnetic nanoparticles coated with anti-EpCAM. The magnetic nanoparticles were trapped on the wavy herringbone U-shaped sites on the polydimethylsiloxane (PDMS) surface by an external magnetic field and the magnetic particles were released by removing the magnetic force. Tests were performed on whole blood at low concentrations (down to 100 mL−1 of HCT-116 cells) with high capture efficiencies in the range of 81%–95%. Although magnetic field sorting has a high sorting accuracy, it requires an external field and is not easily integrated with low throughput. The positive sorting method does not remove the magnetic beads; moreover, it is harmful to cells, and is unsuitable for subsequent tumor cell culture analysis. The negative sorting method does not ensure the purity of separation and has a long processing time.
Acoustic sorting uses the various directions of motion of different particles under the force of acoustic radiation to achieve sorting (Fig. 2c). Acoustic radiation is generated by piezoelectric crystals and sound surface waves (Augustsson et al., 2012; Karthick et al., 2018). The most popular method was developed by Professor Huang Jun of Duke University, in which multiple pairs of forked-finger transducers were used to generate acoustic surface waves and create standing waves to assist in the aggregation of different cells in the flow channel at locations such as wave peaks and troughs, thereby completing sorting (Wu et al., 2017). Antfolk et al. (2015) described a simple acoustic absorption-based cell separator that recovered 86.5% of cancer cells at a sample flow rate of 100 µmL/min. Increasing the acoustic intensity resulted in the 94.8% recovery of cancer cells with 2.2% leukocyte contamination. Wu et al. (2019) presented an acoustic device integrated with 36° lithium niobium oxide coated with indium tin oxide to separate CTCs. Their results showed 91.5% ± 4.5% average separation efficiency of cancer cell lines. A method for the acoustic separation of CTCs from leukocytes was investigated by Wu’s group (Wu et al., 2018). This method integrates acoustics and microfluidics to isolate rare CTCs from peripheral blood at high throughput with minimal cellular damage, maintaining the structural, biological, and functional integrity of the cells. At a throughput of 7.5 mL/h, the method achieves at least 86% recovery of CTCs from leukocytes while maintaining cell proliferation capacity. This acoustic sorting method can achieve high throughput. However, the integrated acoustic radiation generation device increases the integration difficulty and fabrication cost of the chip, while the cell size-based sorting method limits the purity of sorting and is harmful to cells.
Optical sorting is based on the different deflection angles of the trajectories of particles of different sizes and refractive indices under the action of energy traps formed by laser beams (Polimeno et al., 2018). Hu et al. (2019) constructed a light-controlled system to trap tumor cells in the blood (Fig. 2d). The tumor cells were used to target and bind homologous blood cells, which significantly enhanced the difference in optical constants between tumor cells and blood cells and the tumor cells were efficiently sorted (tumor cell recovery of 90% and purity >50%). Chou et al. (2017) proposed a cell manipulation and flow rate control technique based on optical-channeled electrophoresis, in which a four-stage cell isolation system using four optical-based virtual cell filters were designed in the optically-induced-dielectrophoresis system to improve the cell separation efficiency. The method resulted in a cell separation purity of 94.9% ± 0.3% and cancer cell recovery of 54% ± 7%. However, the auxiliary optical system is limited in its highly complex setup, extremely low throughput, and the damage to cells is also unacceptable for clinical use.
Among the passive sorting techniques, microfiltration sorting (Kolostova et al., 2014; Rawal et al., 2016; Sonoda et al., 2020; Zinggeler et al., 2015), such as the use of microcolumns to complete tumor cell screening based on cell particle size, was proposed by Professor Mehmet Toner of Harvard Medical School and the work is published in Nature (Nagrath et al., 2007). Han et al. (2022) designed a reusable membrane filtration device that uses a horizontal rotor and fluid assist in passing CTCs through a centrifugal filtration membrane for rapid and efficient cell capture (Fig. 3a). The device achieved an average capture rate of 95.8% for cancer cells, with a survival rate of >90% and a leukocyte removal rate of 99.72% after four treatments. A new method based on biophysical properties was developed by Liu’s group (Liu et al., 2018), who designed a pyramid-shaped microchamber for efficient isolation of CTC from breast cancer patients at an outlet height of 6 µm and a flow rate of 200 µL/min, with a CTC capture efficiency of over 85%, and no blockage problems. To date, filter-based sorting solutions no longer use a single cutoff diameter. For example, McMasters has reported a lamellar microporous sieve filter that uses microfabrication processes, such as photolithography, to provide arrays of 10, 15, and 20 µm microporous pores that can effectively achieve the screening goals of simultaneously sorting multiple cell sizes; moreover, it is not harmful to health and does not require any external field. However, the sorting purity of microfiltration sorting is extremely low, making it difficult to meet clinical testing requirements.
Figure 3: Schematic diagram of the microfluidic device for separating circulating tumor cells (CTC) in passive sorting. (a) Schematic diagram of a polydimethylsiloxane microfiltration membrane integrated microfluidic device for CTC capture (Han et al., 2022); (b) Schematic diagram of a deterministic lateral displacement microfluidic chip for CTC isolation (Fachin et al., 2017); (c) Schematic of the inertial sorting microfluidic chip for CTC separation (Kulasinghe et al., 2017).
The concept of deterministic lateral displacement (DLD) was introduced by Huang of Princeton University in their study published in Science (Huang et al., 2004). The main design idea was to set up a series of inclined microcolumn arrays in the microfluidic channel to achieve particle sorting based on the different angles of deflection of the trajectories of particles of different sizes after perturbation by the columns (Edd et al., 2020; Fachin et al., 2017; Khodaee et al., 2016; Liu et al., 2018; Tang et al., 2022) (Fig. 3b). Liu et al. (2013) introduced a microfluidic system integrating a microfluidic DLD array and a cell capture structure. The system achieved a CTC capture rate of 90% and capture purity of >50% at a cell concentration of 102 cells/mL. Au et al. (2017) developed a two-stage continuous microfluidic chip to isolate and recover of viable CTC clusters from blood. The method used both the size and asymmetric geometric properties to sort clusters via DLD, with a 99% CTC recovery rate and cell survival rate of over 87%. In 2021, Liu et al. (2021) proposed the concept of the deterministic lateral displacement of filters by combining filtration concepts with DLD structures for hydrodynamic sorting. This resulted in a separation efficiency of >96%, high cell purity of 99.995% through WBC removal, high cell viability of >98%, and high processing efficiency of 1 mL/min. Bhattacharjee et al. (2022) designed an asymmetric microcolumn array that regulated the flow resistance of fluid in the flow channel, which was more conducive to the differentiation of tumor cells from leukocytes. Ahmed et al. (2017) developed a size-controlled immunocapture chip with a triangular microarray structure that selectively enhances CTC interactions by deterministic lateral displacement. The microcolumns of this chip successfully captured more than 90% of CTCs (92.2% ± 6.4%). However, the sorting purity of deterministic lateral displacement sorting was lower, and the processing of microcolumn arrays was more difficult, resulting in the low success rate of microarray fabrication.
Currently, inertial sorting is the most popular and widely used sorting scheme in passive sorting (Fig. 3c). The main principle is based on the hydrodynamic properties of particles in the flow channel under the joint action of inertial lift, Dean’s traction, and other forces to complete the high-throughput separation of particles of different sizes (Mutlu et al., 2020; Oakey et al., 2010; Smith et al., 2021; Zhou et al., 2019). In addition to Toner’s group at Harvard University, which pioneered the concept of inertial microfluidics in the Nature Journal, di Carlo et al. (2009) at the University of California, Los Angeles, proposed the concept of inertial microfluidics. Khoo et al. (2018) at the National University of Singapore have been working on developing and commercializing spiral inertial microfluidic chips for tumor cell sorting. Wang et al. (2022) at Southeast University have been working on the theory and application of inertial microfluidic chips. A double-spiral microfluidic device with a finely tunable cutoff value of 9 µm and a separation range of 2 µm was proposed and validated by Pena (Rodriguez-Pena et al., 2022). They isolated 17 CTCs/mL from the peripheral blood of a hepatocellular carcinoma patient with leukocyte and erythrocyte removal rates of 96.03% and 99.99%, respectively. Using the inertial lift, Gao et al. (2020) developed a label-free separation microfluidic device using multi-stage channels, a chip with mainly a herringbone channel, a rectangular vessel, and inertial focusing microchannels, which can be used to separate CTC. At a flow rate of 9 L/min, the device had a separation efficiency of 90% and a purity of 84.96%. However, this method is based solely on cell size, and the purity of separation is unable to meet clinical requirement.
The above methods were compared in detail and summarized in Table 1.
CTC, as one of the main markers of liquid biopsy, is an effective means of improving our understanding of cancer metastasis. The ability to sort high-purity, high-viability CTCs is, therefore, critical to the development of clinical medicine and cell biology. Researchers have conducted various more efficient microfluidic devices than traditional filtration, centrifugation, and immunomagnetic bead methods. This paper reviewed the latest developments in microfluidic-based CTC sorting via active and passive sorting and compared the advantages and disadvantages of different methods. In general, additional external sources, such as electrodes, signal generators, electromagnets, forked-finger transducers, and light transmission paths, must be integrated into the microchip when building an active sorting system. However, these additional devices increase the complexity of the system and the difficulty of processing. More importantly, the addition of sources is detrimental to cell activity and, consequently, to clinical studies of downstream cancers. In contrast, the passive sorting method does not affect cell activity. However, because the cell particle size is the main sorting criterion, it cannot overcome the problem of the size overlap between CTCs and leukocytes owing to the presence of cell heterogeneity. Therefore, chips with integrated active and passive sorting should be the focus of subsequent research. The multimethod coupled integrated system could solve the current problems of CTC sorting, such as the inability to simultaneously achieve a high recovery rate, high purity, and high throughput. Based on the current research status, we believe the microfluidic device could be more reliable and stable in the future when separating CTCs, and these novel microfluidic technologies for CTC capture and isolation will play a crucial role in the early detection of cancer, real-time monitoring and more.
Funding Statement: This work was supported by the Science and Technology Project of the Hebei Education Department [No. BJK2023016] and the Central Guidance on Local Science and Technology Development Fund [Grant No. 226Z1701G].
Author Contributions: Jiahao Zhang and Yixing Gou conducted the literature review and prepared the manuscript. Zirui Li and Yixing Gou designed the manuscript structure. Jie Ren and Yixing Gou revised the manuscript. All authors have reviewed and approved the final version of the manuscript.
Ethics Approval: Not applicable.
Conflicts of Interest: The authors declare that they have no conflicts of interest to report regarding the present study.
References
Ahmed MG, Abate MF, Song YL, Zhu Z, Yan F, Xu Y, Wang XM, Li QB, Yang CY (2017). Isolation, detection, and antigen-based profiling of circulating tumor cells using a size-dictated immunocapture chip. Angewandte Chemie International Edition 56: 10681–10685. https://doi.org/10.1002/anie.201702675 [Google Scholar] [PubMed] [CrossRef]
Alix-Panabieres C, Pantel K (2013). Circulating tumor cells: Liquid biopsy of cancer. Clinical Chemistry 59: 110–118. https://doi.org/10.1373/clinchem.2012.194258 [Google Scholar] [PubMed] [CrossRef]
Alix-Panabieres C, Pantel K (2021). Liquid biopsy: From discovery to clinical application. Cancer Discovery 11: 858–873. https://doi.org/10.1158/2159-8290.CD-20-1311 [Google Scholar] [PubMed] [CrossRef]
Alix-Panabières C, Pantel K (2016). Clinical applications of circulating tumor cells and circulating tumor DNA as liquid biopsy. Cancer Discovery 6: 479–491. https://doi.org/10.1158/2159-8290.CD-15-1483 [Google Scholar] [PubMed] [CrossRef]
Antfolk M, Antfolk C, Lilja H, Laurell T, Augustsson P (2015). A single inlet two-stage acoustophoresis chip enabling tumor cell enrichment from white blood cells. Lab on a Chip 15: 2102–2109. https://doi.org/10.1039/C5LC00078E [Google Scholar] [PubMed] [CrossRef]
Arslan ZC, Yalcin YD, Kulah H (2022). Label-free enrichment of MCF7 breast cancer cells from leukocytes using continuous flow dielectrophoresis. Electrophoresis 43: 1531–1544. https://doi.org/10.1002/elps.202100318 [Google Scholar] [PubMed] [CrossRef]
Au SH, Edd J, Stoddard AE, Wong KHK, Fachin F, Maheswaran S, Haber DA, Stott SL, Kapur R, Toner M (2017). Microfluidic isolation of circulating tumor cell clusters by size and asymmetry. Scientific Reports 7: 2433. https://doi.org/10.1038/s41598-017-01150-3 [Google Scholar] [PubMed] [CrossRef]
Augustsson P, Magnusson C, Nordin M, Lilja H, Laurell T (2012). Microfluidic, label-free enrichment of prostate cancer cells in blood based on acoustophoresis. Analytical Chemistry 84: 7954–7962. https://doi.org/10.1021/ac301723s [Google Scholar] [PubMed] [CrossRef]
Autebert J, Coudert B, Bidard FC, Pierga JY, Descroix S, Malaquin L, Viovy JL (2012). Microfluidic: An innovative tool for efficient cell sorting. Methods 57: 297–307. https://doi.org/10.1016/j.ymeth.2012.07.002 [Google Scholar] [PubMed] [CrossRef]
Baccelli I, Schneeweiss A, Riethdorf S, Stenzinger A, Schillert A et al. (2013). Identification of a population of blood circulating tumor cells from breast cancer patients that initiates metastasis in a xenograft assay. Nature Biotechnology 31: 539–544. https://doi.org/10.1038/nbt.2576 [Google Scholar] [PubMed] [CrossRef]
Bakhshi A, Nasab EH, Ghafouri V, Rahmanian M, Badieirostami M, IEEE (2021). Design and demonstration of a novel microfluidic channel for trapping circulating tumor cells with magnetophoresis. 29th Iranian Conference on Electrical Engineering (ICEE), pp. 74–77. Mashhad. https://doi.org/10.1109/icee52715.2021.9544197 [Google Scholar] [CrossRef]
Belotti Y, Lim CT (2021). Microfluidics for liquid biopsies: Recent advances, current challenges, and future directions. Analytical Chemistry 93: 4727–4738. https://doi.org/10.1021/acs.analchem.1c00410 [Google Scholar] [PubMed] [CrossRef]
Bhat MP, Thendral V, Uthappa UT, Lee KH, Kigga M, Altalhi T, Kurkuri MD, Kant K (2022). Recent advances in microfluidic platform for physical and immunological detection and capture of circulating tumor cells. Biosensors 12: 220. https://doi.org/10.3390/bios12040220 [Google Scholar] [PubMed] [CrossRef]
Bhattacharjee R, Kumar R, Al-Turjman F (2022). A novel approach for tuning of fluidic resistance in deterministic lateral displacement array for enhanced separation of circulating tumor cells. Cognitive Computation 14: 1660–1676. https://doi.org/10.1007/s12559-021-09904-y [Google Scholar] [CrossRef]
Cann GM, Gulzar ZG, Cooper S, Li R, Luo SJ et al. (2012). mRNA-seq of single prostate cancer circulating tumor cells reveals recapitulation of gene expression and pathways found in prostate cancer. PLoS One 7: e49144. https://doi.org/10.1371/journal.pone.0049144 [Google Scholar] [PubMed] [CrossRef]
Chou WP, Wang HM, Chang JH, Chiu TK, Hsieh CH, Liao CJ, Wu MH (2017). The utilization of optically-induced-dielectrophoresis (ODEP)-based virtual cell filters in a microfluidic system for continuous isolation and purification of circulating tumour cells (CTCs) based on their size characteristics. Sensors and Actuators B-Chemical 241: 245–254. https://doi.org/10.1016/j.snb.2016.10.075 [Google Scholar] [CrossRef]
Contreras-Naranjo JC, Wu HJ, Ugaz VM (2017). Microfluidics for exosome isolation and analysis: Enabling liquid biopsy for personalized medicine. Lab on a Chip 17: 3558–3577. https://doi.org/10.1039/C7LC00592J [Google Scholar] [PubMed] [CrossRef]
Cortes-Hernandez LE, Eslami Z, Alix-Panabieres C (2020). Circulating tumor cell as the functional aspect of liquid biopsy to understand the metastatic cascade in solid cancer. Molecular Aspects of Medicine 72: 100816. https://doi.org/10.1016/j.mam.2019.07.008 [Google Scholar] [PubMed] [CrossRef]
D’Avola D, Villacorta-Martin C, Martins SN, Craig A, Labgaa I et al. (2018). High-density single cell mRNA sequencing to characterize circulating tumor cells in hepatocellular carcinoma. Scientific Reports 8: 11570. https://doi.org/10.1038/s41598-018-30047-y [Google Scholar] [PubMed] [CrossRef]
de Rubis G, Krishnan SR, Bebawy M (2019). Liquid biopsies in cancer diagnosis, monitoring, and prognosis. Trends in Pharmacological Sciences 40: 172–186. https://doi.org/10.1016/j.tips.2019.01.006 [Google Scholar] [PubMed] [CrossRef]
de Wit S, Zeune LL, Hiltermann TJN, Groen HJM, van Dalum G, Terstappen L (2018). Classification of cells in CTC-enriched samples by advanced image analysis. Cancers 10: 377. https://doi.org/10.3390/cancers10100377 [Google Scholar] [PubMed] [CrossRef]
di Carlo D, Edd JF, Humphry KJ, Stone HA, Toner M (2009). Particle segregation and dynamics in confined flows. Physical Review Letters 102: 094503. https://doi.org/10.1103/PhysRevLett.102.094503 [Google Scholar] [PubMed] [CrossRef]
Dillekas H, Rogers MS, Straume O (2019). Are 90% of deaths from cancer caused by metastases? Cancer Medicine 8: 5574–5576. https://doi.org/10.1002/cam4.2474 [Google Scholar] [PubMed] [CrossRef]
Edd JF, Mishra A, Dubash TD, Herrera S, Mohammad R et al. (2020). Microfluidic concentration and separation of circulating tumor cell clusters from large blood volumes. Lab on a Chip 20: 558–567. https://doi.org/10.1039/c9lc01122f [Google Scholar] [PubMed] [CrossRef]
Fachin F, Spuhler P, Martel-Foley JM, Edd JF, Barber TA et al. (2017). Monolithic chip for high-throughput blood cell depletion to sort rare circulating tumor cells. Scientific Reports 7: 10936. https://doi.org/10.1038/s41598-017-11119-x [Google Scholar] [PubMed] [CrossRef]
Farace F, Massard C, Vimond N, Drusch F, Jacques N et al. (2011). A direct comparison of CellSearch and ISET for circulating tumour-cell detection in patients with metastatic carcinomas. British Journal of Cancer 105: 847–853. https://doi.org/10.1038/bjc.2011.294 [Google Scholar] [PubMed] [CrossRef]
Gao RK, Cheng L, Wang SY, Bi XB, Wang XL et al. (2020). Efficient separation of tumor cells from untreated whole blood using a novel multistage hydrodynamic focusing microfluidics. Talanta 207: 120261. https://doi.org/10.1016/j.talanta.2019.120261 [Google Scholar] [PubMed] [CrossRef]
Ghafouri V, Badieirostami M (2021). Enhancement of the rates of CTCs trapping by integrating nanowire substrate and EpCAM antibody conjugated with magnetite nanoparticles in a microfluidic device. Microfluidics and Nanofluidics 25: 103. https://doi.org/10.1007/s10404-021-02505-z [Google Scholar] [CrossRef]
Gorgannezhad L, Umer M, Islam MN, Nguyen NT, Shiddiky MJA (2018). Circulating tumor DNA and liquid biopsy: Opportunities, challenges, and recent advances in detection technologies. Lab on a Chip 18: 1174–1196. https://doi.org/10.1039/C8LC00100F [Google Scholar] [PubMed] [CrossRef]
Gupta V, Jafferji I, Garza M, Melnikova VO, Hasegawa DK, Pethig R, Davis DW (2012). ApoStream (TMa new dielectrophoretic device for antibody independent isolation and recovery of viable cancer cells from blood. Biomicrofluidics 6: 024133. https://doi.org/10.1063/1.4731647 [Google Scholar] [PubMed] [CrossRef]
Hajba L, Guttman A (2014). Circulating tumor-cell detection and capture using microfluidic devices. TrAC-Trends in Analytical Chemistry 59: 9–16. https://doi.org/10.1016/j.trac.2014.02.017 [Google Scholar] [CrossRef]
Han JT, Lu CY, Shen MZ, Sun XY, Mo XD, Yang G (2022). Fast, reusable, cell uniformly distributed membrane filtration device for separation of circulating tumor cells. ACS Omega 7: 20761–20767. https://doi.org/10.1021/acsomega.2c01153 [Google Scholar] [PubMed] [CrossRef]
Hirahata T, Ul Quraish R, Quraish AU, Ul Quraish S, Naz M, Razzaq MA (2022). Liquid biopsy: A distinctive approach to the diagnosis and prognosis of cancer. Cancer Informatics 21: 11769351221076062. https://doi.org/10.1177/11769351221076062 [Google Scholar] [PubMed] [CrossRef]
Hu XJ, Zhu DM, Chen M, Chen KK, Liu HL, Liu W, Yang Y (2019). Precise and non-invasive circulating tumor cell isolation based on optical force using homologous erythrocyte binding. Lab on a Chip 19: 2549–2556. https://doi.org/10.1039/C9LC00361D [Google Scholar] [PubMed] [CrossRef]
Huang LR, Cox EC, Austin RH, Sturm JC (2004). Continuous particle separation through deterministic lateral displacement. Science 304: 987–990. https://doi.org/10.1126/science.1094567 [Google Scholar] [PubMed] [CrossRef]
Jahangiri M, Ranjbar-Torkamani M, Abadijoo H, Ghaderinia M, Ghafari H, Mamdouh A, Abdolahad M (2020). Low frequency stimulation induces polarization-based capturing of normal, cancerous and white blood cells: A new separation method for circulating tumor cell enrichment or phenotypic cell sorting. Analyst 145: 7636–7645. https://doi.org/10.1039/D0AN01033B [Google Scholar] [PubMed] [CrossRef]
Kahn HJ, Presta A, Yang LY, Blondal J, Trudeau M, Lickley L, Holloway C, McCready DR, Maclean D, Marks A (2004). Enumeration of circulating tumor cells in the blood of breast cancer patients after filtration enrichment: Correlation with disease stage. Breast Cancer Research and Treatment 86: 237–247. https://doi.org/10.1023/B:BREA.0000036897.92513.72 [Google Scholar] [PubMed] [CrossRef]
Karthick S, Pradeep PN, Kanchana P, Sen AK (2018). Acoustic impedance-based size-independent isolation of circulating tumour cells from blood using acoustophoresis. Lab on a Chip 18: 3802–3813. https://doi.org/10.1039/C8LC00921J [Google Scholar] [PubMed] [CrossRef]
Khodaee F, Movahed S, Fatouraee N, Daneshmand F (2016). Numerical simulation of separation of circulating tumor cells from blood stream in deterministic lateral displacement (DLD) microfluidic channel. Journal of Mechanics 32: 463–471. https://doi.org/10.1017/jmech.2015.91 [Google Scholar] [CrossRef]
Khoo BL, Grenci G, Lim YB, Lee SC, Han J, Lim CT (2018). Expansion of patient-derived circulating tumor cells from liquid biopsies using a CTC microfluidic culture device. Nature Protocols 13: 34–58. https://doi.org/10.1038/nprot.2017.125 [Google Scholar] [PubMed] [CrossRef]
Kim S, Han SI, Park MJ, Jeon CW, Joo YD, Choi IH, Han KH (2013). Circulating tumor cell microseparator based on lateral magnetophoresis and immunomagnetic nanobeads. Analytical Chemistry 85: 2779–2786. https://doi.org/10.1021/ac303284u [Google Scholar] [PubMed] [CrossRef]
Kolostova K, Zhang Y, Hoffman RM, Bobek V (2014). In vitro culture and characterization of human lung cancer circulating tumor cells isolated by size exclusion from an orthotopic nude-mouse model expressing fluorescent protein. Journal of Fluorescence 24: 1531–1536. https://doi.org/10.1007/s10895-014-1439-3 [Google Scholar] [PubMed] [CrossRef]
Kulasinghe A, Tran THP, Blick T, O’Byrne K, Thompson EW, Warkiani ME, Nelson C, Kenny L, Punyadeera C (2017). Enrichment of circulating head and neck tumour cells using spiral microfluidic technology. Scientific Reports 7: 42517. https://doi.org/10.1038/srep42517 [Google Scholar] [PubMed] [CrossRef]
Liu ZB, Chen R, Li Y, Liu JQ, Wang P, Xia XF, Qin LD (2018). Integrated microfluidic chip for efficient isolation and deformability analysis of circulating tumor cells. Advanced Biosystems 2: 1800200. https://doi.org/10.1002/adbi.201800200 [Google Scholar] [CrossRef]
Liu ZB, Huang YQ, Liang WL, Bai J, Feng HT et al. (2021). Cascaded filter deterministic lateral displacement microchips for isolation and molecular analysis of circulating tumor cells and fusion cells. Lab on a Chip 21: 2881–2891. https://doi.org/10.1039/D1LC00360G [Google Scholar] [PubMed] [CrossRef]
Liu F, Wang SB, Lu ZG, Sun YM, Yang CG et al. (2018). A simple pyramid-shaped microchamber towards highly efficient isolation of circulating tumor cells from breast cancer patients. Biomedical Microdevices 20: 83. https://doi.org/10.1007/s10544-018-0326-0 [Google Scholar] [PubMed] [CrossRef]
Liu ZB, Zhang W, Huang F, Feng HT, Shu WL, Xu XP, Chen Y (2013). High throughput capture of circulating tumor cells using an integrated microfluidic system. Biosensors & Bioelectronics 47: 113–119. https://doi.org/10.1016/j.bios.2013.03.017 [Google Scholar] [PubMed] [CrossRef]
Medoro G (2003). Introducing DEPARRAY (TMIndividual manipulation and detection of more than 10,000 cells in a drop of sample. 8th Italian Conference on Sensors and Microsystems, pp. 361–363. Trento. https://doi.org/10.1142/9789812702944_0056 [Google Scholar] [CrossRef]
Mishra A, Dubash TD, Edd JF, Jewett MK, Garre SG et al. (2020). Ultrahigh-throughput magnetic sorting of large blood volumes for epitope-agnostic isolation of circulating tumor cells. Proceedings of the National Academy of Sciences of the United States of America 117: 16839–16847. https://doi.org/10.1073/pnas.2006388117 [Google Scholar] [PubMed] [CrossRef]
Mutlu BR, Dubash TD, Dietsche C, Mishra A, Ozbey A, Keim K, Edd JF, Haber DA, Maheswaran S, Toner M (2020). In-flow measurement of cell-cell adhesion using oscillatory inertial microfluidics. Lab on a Chip 20: 1612–1620. https://doi.org/10.1039/D0LC00089B [Google Scholar] [PubMed] [CrossRef]
Nagrath S, Sequist LV, Maheswaran S, Bell DW, Irimia D et al. (2007). Isolation of rare circulating tumour cells in cancer patients by microchip technology. Nature 450: 1235-1239. https://doi.org/10.1038/nature06385 [Google Scholar] [PubMed] [CrossRef]
Nowell PC (1976). The clonal evolution of tumor cell populations. Science 194: 23–28. https://doi.org/10.1126/science.959840 [Google Scholar] [PubMed] [CrossRef]
Oakey J, Applegate RW, Arellano E, di Carlo D, Graves SW, Toner M (2010). Particle focusing in staged inertial microfluidic devices for flow cytometry. Analytical Chemistry 82: 3862–3867. https://doi.org/10.1021/ac100387b [Google Scholar] [PubMed] [CrossRef]
Polimeno P, Magazzu A, Iati MA, Patti F, Saija R et al. (2018). Optical tweezers and their applications. Journal of Quantitative Spectroscopy & Radiative Transfer 218: 131–150. https://doi.org/10.1016/j.jqsrt.2018.07.013 [Google Scholar] [CrossRef]
Rawal S, Ao Z, Agarwal A (2016). Capture and release of viable circulating tumor cells from blood. JoVE (Journal of Visualized Experiments) 54435. https://doi.org/10.3791/54435-v [Google Scholar] [CrossRef]
Rodriguez-Pena A, Armendariz E, Oyarbide A, Morales X, Ortiz-Espinosa S et al. (2022). Design and validation of a tunable inertial microfluidic system for the efficient enrichment of circulating tumor cells in blood. Bioengineering & Translational Medicine 7: e10331. https://doi.org/10.1002/btm2.10331 [Google Scholar] [PubMed] [CrossRef]
Rushton AJ, Nteliopoulos G, Shaw JA, Coombes RC (2021). A review of circulating tumour cell enrichment technologies. Cancers 13: 970. https://doi.org/10.3390/cancers13050970 [Google Scholar] [PubMed] [CrossRef]
Semaan A, Bernard V, Kim DU, Lee JJ, Huang J et al. (2021). Characterisation of circulating tumour cell phenotypes identifies a partial-EMT sub-population for clinical stratification of pancreatic cancer. British Journal of Cancer 124: 1970–1977. https://doi.org/10.1038/s41416-021-01350-9 [Google Scholar] [PubMed] [CrossRef]
Shaw JA, Guttery DS, Hills A, Fernandez-Garcia D, Page K et al. (2017). Mutation analysis of cell-free DNA and single circulating tumor cells in metastatic breast cancer patients with high circulating tumor cell counts. Clinical Cancer Research 23: 88–96. https://doi.org/10.1158/1078-0432.CCR-16-0825 [Google Scholar] [PubMed] [CrossRef]
Shi WT, Wang SQ, Maarouf A, Uhl CG, He R, Yunus D, Liu YL (2017). Magnetic particles assisted capture and release of rare circulating tumor cells using wavy-herringbone structured microfluidic devices. Lab on a Chip 17: 3291–3299. https://doi.org/10.1039/C7LC00333A [Google Scholar] [PubMed] [CrossRef]
Shields CW, Reyes CD, Lopez GP (2015). Microfluidic cell sorting: A review of the advances in the separation of cells from debulking to rare cell isolation. Lab on a Chip 15: 1230–1249. https://doi.org/10.1039/C4LC01246A [Google Scholar] [PubMed] [CrossRef]
Smith KJ, Jana JA, Kaehr A, Purcell E, Opdycke T, Paoletti C, Cooling L, Thamm DH, Hayes DF, Nagrath S (2021). Inertial focusing of circulating tumor cells in whole blood at high flow rates using the microfluidic CTCKey (TM) device for CTC enrichment. Lab on a Chip 21: 3559–3572. https://doi.org/10.1039/D1LC00546D [Google Scholar] [PubMed] [CrossRef]
Sonoda T, Yanagitani N, Suga K, Yoshizawa T, Nishikawa S et al. (2020). A novel system to detect circulating tumor cells using two different size-selective microfilters. Anticancer Research 40: 5577–5582. https://doi.org/10.21873/anticanres.14570 [Google Scholar] [PubMed] [CrossRef]
Tang H, Niu JQ, Pan XN, Jin H, Lin SJ, Cui DX (2022). Topology optimization based deterministic lateral displacement array design for cell separation. Journal of Chromatography A 1679: 463384. https://doi.org/10.1016/j.chroma.2022.463384 [Google Scholar] [PubMed] [CrossRef]
Todenhofer T, Hennenlotter J, Feyerabend S, Aufderklamm S, Mischinger J et al. (2012). Preliminary experience on the use of the adnatest (R) system for detection of circulating tumor cells in prostate cancer patients. Anticancer Research 32: 3507–3513. [Google Scholar] [PubMed]
Voena C, Locatelli G, Castellino C, Omede P, Ladetto M et al. (2002). Qualitative and quantitative polymerase chain reaction detection of the residual myeloma cell contamination after positive selection of CD34+ cells with small- and large-scale Miltenyi cell sorting system. British Journal of Haematology 117: 642–645. https://doi.org/10.1046/j.1365-2141.2002.03448.x [Google Scholar] [PubMed] [CrossRef]
Wang CL, Chen Y, Gu XY, Zhang XX, Gao CC, Dong LJ, Zheng SY, Feng SC, Xiang N (2022). Low-cost polymer-film spiral inertial microfluidic device for label-free separation of malignant tumor cells. Electrophoresis 43: 464–471. https://doi.org/10.1002/elps.202100232 [Google Scholar] [PubMed] [CrossRef]
Wang P, Xue JL, Wu SM, Pei YB, Xu LH, Wang YL (2021). Cell-friendly isolation and pH-sensitive controllable release of circulating tumor cells by Fe3O4@CaCO3 nanoplatform. Advanced Materials Interfaces 8: 2101191. https://doi.org/10.1002/admi.202101191 [Google Scholar] [CrossRef]
Wu MX, Huang PH, Zhang R, Mao ZM, Chen CY et al. (2018). Circulating tumor cell phenotyping via high-throughput acoustic separation. Small 14: 1801131. https://doi.org/10.1002/smll.201801131 [Google Scholar] [PubMed] [CrossRef]
Wu ZZ, Jiang HQ, Zhang LL, Yi KZ, Cui H, Wang FB, Liu W, Zhao XZ, Zhou FL, Guo SS (2019). The acoustofluidic focusing and separation of rare tumor cells using transparent lithium niobate transducers. Lab on a Chip 19: 3922–3930. https://doi.org/10.1039/C9LC00874H [Google Scholar] [PubMed] [CrossRef]
Wu MX, Ouyang YS, Wang ZY, Zhang R, Huang PH et al. (2017). Isolation of exosomes from whole blood by integrating acoustics and microfluidics. Proceedings of the National Academy of Sciences of the United States of America 114: 10584–10589. https://doi.org/10.1073/pnas.1709210114 [Google Scholar] [PubMed] [CrossRef]
Zhao WJ, Zhu TT, Cheng R, Liu YF, He J et al. (2016). Label-free and continuous-flow ferrohydrodynamic separation of HeLa cells and blood cells in biocompatible ferrofluids. Advanced Functional Materials 26: 3990–3998. https://doi.org/10.1002/adfm.201503838 [Google Scholar] [PubMed] [CrossRef]
Zhou J, Kulasinghe A, Bogseth A, O’Byrne K, Punyadeera C, Papautsky I (2019). Isolation of circulating tumor cells in non-small-cell-lung-cancer patients using a multi-flow microfluidic channel. Microsystems & Nanoengineering 5: 8. https://doi.org/10.1038/s41378-019-0045-6 [Google Scholar] [PubMed] [CrossRef]
Zhu DM, Wu L, Suo M, Gao S, Xie W et al. (2018). Engineered red blood cells for capturing circulating tumor cells with high performance. Nanoscale 10: 6014–6023. https://doi.org/10.1039/C7NR08032H [Google Scholar] [PubMed] [CrossRef]
Zinggeler M, Brandstetter T, Ruhe J (2015). Towards high performance detection of circulating tumor cells in whole blood. Procedia Engineering 120: 380–383. https://doi.org/10.1016/j.proeng.2015.08.645 [Google Scholar] [CrossRef]
Cite This Article
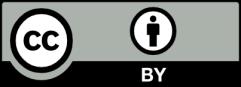
This work is licensed under a Creative Commons Attribution 4.0 International License , which permits unrestricted use, distribution, and reproduction in any medium, provided the original work is properly cited.