Open Access
REVIEW
Mechanisms and applications of antitumor immunotherapy of responsive drug-loaded nanoparticles in breast cancer
School of Medical Imaging, Hangzhou Medical College, Hangzhou, 310053, China
* Corresponding Authors: DAJIANG WANG. Email: ; JIAHUI LU. Email:
(This article belongs to the Special Issue: Immunotherapy for Breast Cancer)
BIOCELL 2023, 47(7), 1483-1498. https://doi.org/10.32604/biocell.2023.028457
Received 19 December 2022; Accepted 06 March 2023; Issue published 21 June 2023
Abstract
During the chemotherapy of tumors, the cytotoxic effect of drugs is vital to kill tumor cells, and the delivery of a chemotherapeutic agent is of great importance for optimal therapeutic effects. The high in vivo clearance rate and low delivery efficiency of conventional chemotherapeutic agents affect the therapeutic effect. In recent years, the responsive drug delivery nanosystem has received increasing concern owing to its excellent biocompatibility, stable delivery performance, and controlled drug release strategies. To lucidly explain the cytocidal and immunotherapeutic effects of such responsive nanosystems in breast cancer, this review discusses the various stimuli and responses of drug-loaded liposomal nanosystems. The light/magnetic response of drug-loaded bionic membranes nanosystems and the heat/magnetic response of drug-loaded iron oxide nanosystems are also elaborated. Their cancer cell-killing efficacy and antitumor immunotherapeutic effects are also scrutinized.Keywords
Abbreviations
PEG | Polyethylene glycol |
FA | Folic acid |
HA | Hyaluronic acid |
CIS | Cisplatin |
EPI | Epirubicin |
Hol | Honokiol |
PTX | Paclitaxel |
AND | Adenosine |
oxHA | Oxidized HA |
CD44 | CD44-receptors |
Cu2+ | Copper ions |
TPGS | D-α-tocopheryl polyethylene glycol succinate |
PBAE | A non-toxic degradable pH-sensitive polymer-poly (β-amino ester) |
SS-PC | Disulfide phosphatidylcholine |
EBP | endoglin-binding peptide |
CHEMS | Cholesteryl hemisuccinate |
IDO | Indoleamine 2,3-dioxygenase |
DTX | Docetaxel |
shRNA | Short hairpin RNA |
Cys | Cystamine hydrochloride |
Poly IC | Polycytidylic acid |
SPIONs | Superparamagnetic iron oxide nanoparticles |
DMSA | Dimercaptosuccinic acid |
MNPs | Magnetic nanoparticles |
PDC | Phosphatidylcholine |
DOPE | 1,2-Dioleoyl-sn-glycero-3-phosphoethanolamine |
DSPE | 1,2-distearoyl-sn-glycero-3-phosphoethanolamine |
DSPE | 1,2-distearoyl-sn-glycero-3-phospho ethanol amine |
Breast cancer is a malignant tumor that seriously endangers human health. In 2020, 2261 thousand new cases of breast cancer and 685 thousand deaths from breast cancer in women were documented worldwide (Siegel et al., 2021). Cancer immunotherapy is an anti-tumor strategy that activates the body’s immune function to improve the recognition of tumor cells by the immune system. Conventional cancer immunotherapies in use include T-lymphocyte (T cell) activation of type 1 cytokines including interleukin 2 (IL-2) and interleukin 15 (IL-15), immune checkpoint inhibitors, and depletion of immunosuppressive cells. Research has illustrated that immunotherapy has some utility in clinical applications. However, the systemic administration of immunotherapeutic agents can lead to their distribution in normal tissues as well, resulting in side effects on normal tissues. The non-specific off-target activation of the immune system also causes autoimmune-like damage to normal tissues. Theoretically, an ideal therapy probably should activate the local host immune response while selectively killing cancer cells.
The short circulation time, low targeting efficiency, poor tumor permeability, and uncontrolled drug release of conventional tumor-targeted drug delivery systems (DDS) limit their further clinical applications. The preparation of liposomal nanoparticles with the controlled release is one of the solutions. The excellent biocompatibility of liposomal drugs, the natural targeting advantages of bionic membranes with the advantages of liposomes, and the magnetic targeting advantages of iron oxide nanoparticles all provide new ideas for antitumor work with nanomaterials, as shown in Table 1. With different stimulus-response mechanisms, nanoparticle-based stimulus-responsive DDS can demonstrate more efficient trigger sensitivity and synergistic controlled release effects through rational “switch” design, effectively improving the side effects of antitumor therapy. As illustrated in Fig. 1, we present a review of liposomes, cell membrane biomimetic-modified nanoparticles, and iron oxide nanoparticles as cases of responsive nanoparticles which is indispensable in drug delivery and tumor therapy.
Figure 1: The stimuli and response mechanisms of drug-loaded nanosystems.
Drug-Loaded Liposome Nanosystems
Clinical experiments with drug-loaded liposomes
Liposomes are biofilm drug carriers with high biosafety, high bioavailability, high delivery potential, non-toxicity, and non-immunogenicity, and are considered to be efficient DDS (Shah et al., 2020). The mobility and amphiphilic molecular properties of phospholipid bilayers empower liposomes with the ability to protect their contents and control the drug release, as well as enhance the solubility of water- and fat-soluble drugs (Inglut et al., 2020; Witika et al., 2020; Thi et al., 2021). In 1995, Food and Drug Administration (FDA) approved the first liposomal adriamycin drug doxorubicin hydrochloride (Doxil) for clinical use. Doxil has shown excellent cancer inhibition in various solid tumors (Paridaens et al., 2000). At present, the main liposomal adriamycin drugs clinically approved for breast cancer treatment are Doxil®, Lipodox®, Liposomal doxorubicin (Liposomal), and non-PEGylated Myocet®. In recent years, Chinese generic liposomal adriamycin molecules (such as Fudan Zhangjiang’s “Ribodox”, ShiyaoOuyi’s “Domesol” and Changzhou Jinyuan’s “Lixin”) have also been approved for marketing. These FDA-approved liposomal adriamycin chemotherapy drugs are in different stages of breast cancer treatment clinical trials. A single polyglycolylated liposomal adriamycin has reached a Phase IV clinical trial (NCT00604968) and passed the efficacy and safety evaluation for treatment in older women (Green et al., 2011). Long-circulating liposome Doxil is in phase III clinical trial (NCT00003782) and has demonstrated efficacy in patients with axillary lymph node metastases. Further, the combination of chemotherapeutic agents with different killing mechanisms (Doxorubicin, Cyclophosphamide, and Paclitaxel) has demonstrated better efficacy than single-agent chemotherapy strategies in a phase III clinical trial (NCT00003782) (Budd et al., 2015).
Mechanism of cell killing and immune action of drug-loaded liposomes
The above-mentioned drug-loaded liposomes have been well used in clinical applications. Researchers have further investigated the mechanism of drug-loaded liposomes, among which Doxil has been most intensively studied. Studies have shown that Doxil drives cell death mainly through oxidative stress, accumulation of damaged organelles, and mitochondrial dysfunction. For example, Doxil can mediate cell death by enhancing tumor protein 53 (P53) signaling, resulting in decreased levels of GATA binding protein 4 (GATA-4) expression and protein 53 (p300) degradation. Glutathione S-transferase P1 (GSTP1) can be utilized to promote cellular autophagy by blocking the phosphatidylinositol-3-kinase and the mammalian target of rapamycin (PI3K-Akt-mTOR) pathway signaling through interaction with phosphoinositide 3-kinase (PI3K), protein 110α (p110α). Additionally, the protein transferrin receptor (TNFR)-associated death protein can be activated by transferrin receptor 1 (TRFR1) through tumor necrosis factor-α (TNF-α), leading to necrotic vesicle formation and release of inflammatory factors to induce the cell death eventually (Chen et al., 2011; Effimia, 2021; Wang et al., 2021b).
Meanwhile, the mechanism of liposomal adriamycin-triggered antitumor immune action also has received attention. A study by Dastmalchi et al. (2020) found that some chemotherapeutic agents, including liposomal adriamycin, can mediate immunogenic cell death (ICD) at low doses. Besides, the chemotherapeutic agent can mediate danger signals release and trigger damage-associated molecular patterns (DAMPs), thus enhancing anti-tumor immune efficacy in vivo (Ding et al., 2021). Liposomal adriamycin could also trigger immunogenic effects by inducing membrane exposure of highly immunostimulatory DAMPs, allowing low amounts of iDAMP/PGE2 to be released into the tumor microenvironment (TME) (Nikolos et al., 2022). Although chemoimmunotherapy has shown some effectiveness, a possible side effect of drug resistance and systemic immunosuppression could also emerge (Tokumaru et al., 2020). Preclinical studies have indicated that biomaterial-based nanocarriers might enhance the immunogenicity of ICD and reduce side effects (Mishchenko et al., 2019). In a recent study, Kim et al. (2022) prepared DP-NPs liposomes with sustained release that induced ICD. In local immunotherapy experiments in mice with breast cancer, increased infiltration of CD4 and CD8 T cells in the target area and triggering of systemic T cell responses induced strong local and systemic anti-tumor immunity (Gao et al., 2021). Drug-loaded liposome systems can compensate for the low efficiency of conventional therapeutic regimens, such as the systemic administration of drugs that disrupt the homeostasis of the immune system at non-target sites and produce lethal toxic effects of systemic immune homeostasis (Jin et al., 2021).
Currently, nano-drug delivery carriers are mainly used for targeted delivery of immunogenic chemotherapeutic drugs of the immunomonoclonal class (anti-programmed death l (PD-1), anti-programmed death ligand 1 (PD-L1), anti-cluster of differentiation 47 (CD47) antibodies, etc.). For these, tumor antigens are released and the damage-associated molecular patterns (DAMPs) is triggered by targeting tumor cells ICD. Therefore, the anti-tumor immunity of natural killer (NK) cells was enhanced. Further, the activity of antigen-presenting cells and cytotoxic T cells was promoted, and the expression of immunosuppressive molecules was reduced in the tumor immune microenvironment. The cytotoxic T-cell production and antigen presentation were increased in secondary lymphoid organs in the peripheral immune system, thereby enhancing cancer immunity (Shi et al., 2019; Zhang et al., 2021b). Among the new immunotherapeutic strategies, liposome-based drug delivery systems are now commonly used for vaccination, tumor normalization, tumor modulation, tumor targeting, and combination therapy (Gu et al., 2020). In addition to immunotherapy, nanoplatforms can be combined with existing antitumor regimens including radiotherapy, phototherapy, or chemotherapy to improve clinical treatment effects (Raju et al., 2022). In short, with the targeting performance of the liposome DDS, it is not only favorable to the effective drug delivery in tumor tissues but also solves the insufficient recruitment of target dendritic cells (DCs) and the interference of immunosuppressive cells or factors in the TME, thereby reducing the possibility of tumor recurrence (Soliman et al., 2022). Therefore, the smarter drug-loaded liposome delivery system is the key to further enhancing the antitumor effect.
Therapeutic and immunotherapeutic effects of responsive drug-loaded liposomes
Responsive nanoliposomes are capable of stable delivery under normal physiological conditions and can be triggered by specific stimuli to undergo carrier disruption (swelling, surface charge reversal, chemical bond breakage, etc.), thereby prolonging blood circulation and enhancing tumor cell uptake (Li et al., 2022; Zhang et al., 2022a). Responsive drug-loaded liposomes have developed rapidly, among which Thermodox® temperature-sensitive liposomes have successfully been used for advanced clinical stages (like breast cancer stage III). Under the performance of temperature-responsive polymers, drug-loaded temperature-sensitive liposomes can maintain structural integrity at room temperature, while the structure disintegrates at high temperatures. Therefore, by virtue of the relationship between temperature and the structure of heat-sensitive liposomes, the manufactured drug-loaded heat-sensitive liposomes can control drug release (Yuba, 2020).
Currently, common responsive drug-loaded liposomes include both endogenous responses (TME responses such as pH responsiveness, enzyme responsiveness, hypoxia response, and redox response) and exogenous responses (physical stimuli such as light heat, magnetic heat, ultrasound) (Sethuraman et al., 2021). Endogenous mechanical stimuli (pressure, tension, shear) can lead to changes in the mechanical properties of the carrier, which in turn release the drug. The responsive nanoliposomes are subjected to specific conditions (such as acidic pH, high enzyme expression, and high redox) in the TME, and the carrier composition sends conformational changes or chemical changes that cause the removal of the outer protective layer of the carrier and the exposure of the functional components for controlled drug release. The rational design of the carrier “switch” can ensure the stable passage of drug molecules through the complex in vivo environment at the expected bioavailability while maximizing the controllability of drug release, tumor tissue specificity, and stimulus-response sensitivity under the premise of therapeutic safety.
Response mechanism and antitumor immunotherapy of pH-responsive drug-loaded liposomes
The tumor microacidic environment is related to tumorigenesis, progression, and development of drug resistance. The cells and tissues in tumor foci in the human body accumulate acidic metabolites in the tumor interstitium, resulting in a slightly acidic (pH = 6.5–7.2) overall environment in the tumor interstitium in contrast to normal tissue sites (pH = 7.4) (Stubbs et al., 2000). Low pH and abnormal vascular systems can hinder the efficacy of drug therapy in solid tumors (Sharma et al., 2006). Based on low pH, high redox, and higher levels of certain enzymes of the TME, pH-responsive liposome delivery carriers can be constructed by taking advantage of changes in polymorphic groups at different pH values (ionization, conformational changes, and solubility changes) or by introducing structures with acid-sensitive bonds such as hydrazone, imine, acyl hydrazone, and acetal/ketone bonds (Norioka et al., 2017).
Stable delivery and controlled release of drugs enable pH-responsive liposomes to trigger local antitumor immune responses (Uthaman et al., 2018). Accelerated drug release and tumor tissue permeation enrichment to promote drug uptake by tumor cells through five ways: sensitive chemical bond breaking, surface charge inversion, responsive removal of the outer protective layer of the carrier, exposure of functional components, and responsive changes in carrier morphology (Meng et al., 2019). This strategy overcomes the deficiency of early or non-release of the drug while arriving target and can locally activate immunity and thus address the problem of a systemic toxic immune response. For example, liposomes constructed with pyridine betaine can reach the target area within 10 min and respond quickly to pH, thus releasing the drug for accumulation (Wang et al., 2021a). Lipids with phosphatidylcholine membranes can undergo structural changes under acidic conditions in response to electrostatic and van der Waals interactions to release drugs (Omolo et al., 2021). IN another study, de Oliveira Silva et al. (2019) constructed circulating pH-sensitive folate-coated doxorubicin-loaded liposomes, which increased the aggregation of drug carriers in the tumor mesenchyme through the enhanced permeability and retention (EPR) effect of liposomes, exocytosis, and active targeting ability of folate molecules. The low pH conditions in the TME could destabilize the hexagonal structure of 1,2-Dioleoyl-sn-glycero-3-phosphoethanolamine (DOPE) molecules and influence the cell membrane affinity. The endocytosis was enhanced to improve doxorubicin (DOX) uptake by cancer cells while also inducing p53-dependent apoptosis and autophagy under non-specific DNA damage and reactive oxygen species (ROS) generation, and enhancing the high immunogenicity in the TME to inhibit the depletion of immunosuppressive cells (regulatory T cells). All this mediated local antitumor immunity, enhanced the antitumor activity of drugs and avoided the occurrence of cardiotoxic side effects of systemic drug administration (Karanth and Murthy, 2007). A pH-sensitive targeted liposomal nanoparticle could improve the stability of the nanoparticle and prolong the circulation time in vivo, showing positive effects on the target tumor treatment (Zhang et al., 2021a). Upregulation of the enzyme content in the TME and lowering the pH concurrently in tumor cells can use the design of a dual-response liposome delivery system. This can include pH-responsive and redox-responsive processes for intracellular internalization and intracellular drug release can lead to significantly higher toxic effects of drugs on breast cancer cells (Gao et al., 2019). For example, the tumor suppression efficiency (~78.7%) of polymer-liposomes (LPDp) with the introduction of pH-responsive polymers was significantly enhanced (p < 0.01) antitumor effect than that of liposomes (LPp) containing PD-L1 inhibitors (~57.5%) and liposomes containing DOX (LD) in a single strategy (Liu et al., 2019b).
Response mechanism and antitumor immunotherapy of GSH-responsive liposomes
Redox homeostasis is one of the necessary conditions to maintain homeostasis in the body. However, some cells in the TME can disrupt local homeostasis to increase local ROS production and glutathione tripeptide (glutamate, cysteine, glycine) or GSH levels (Mirhadi et al., 2020). GSH is a tripeptide containing cysteine, which is an important intracellular reducing agent. The intracellular GSH concentration (0.5~10 mM) is higher than that in the extracellular fluid (2~20 μM). GSH and glutathione disulfide maintain the normal redox potential of cells (Santini et al., 2000). Abnormal ROS production with high levels of GSH can be used to construct redox-responsive vectors, making the vectors highly redox-responsive and sensitive. High levels of GSH in tumor cells destabilize chemical bonds such as disulfide and diselenium bonds, and the high GSH concentration gradient difference in breast cancer tumor cells can be exploited to cause rapid cleavage of disulfide bonds in prodrugs by glutathione, leading to the release of active anticancer drugs (Kong et al., 2016). Redox-responsive liposomes can solve the problem of drug resistance, reduce systemic immune side effects by improving local delivery efficiency, enter cells through passive diffusion of cell membranes, facilitate the cellular uptake of cell-penetrating peptides by endocytosis after completing the targeting step, and enhance immunotherapeutic effects based on the TME using stimulus-response mechanisms to avoid endosomal escape (Naziris et al., 2020). In a study, Luo et al. (2022) designed a fetal calf serum/calf serum (FCS/GCS) platform with high specificity and high efficiency tumor therapeutic nanoparticles using high intracellular GSH levels and hydrogen peroxide (H2O2) in tumors. Caspase activation mechanisms (pro-apoptotic factor cytochrome-C release, endoplasmic reticulum stress, etc.) can activate tumor necrosis factor receptor (TNFR) or Fas receptor to induce apoptosis and further trigger tumor antigen release while DAMP causes an increase in CD8 cells and cytotoxic T cells. The Fenton reaction is used to deplete GSH to produce large amounts of (•OH) to relieve the highly reductive immunosuppressive state of the TME, enhance the non-apoptotic regulatory cell death in the form of high iron levels, and enhance anti-tumor function (Pandrangi et al., 2022).
Most of the currently clinically approved liposomes, including breast cancer treatments, are based on passive targeting strategies. Active targeting strategies are a viable option to address the limited phagocytosis and drug delivery of the endothelial system (Fraguas-Sánchez et al., 2022). Small molecules, antibodies, peptides, and aptamers have been used as affinity molecules for liposomes to make progress in in vivo targeted delivery. Drug enrichment in the target region remains limited due to the complexity of the pathological environment, making active drug target development and clinical translation difficult (Liu et al., 2021). In addition, although liposome synthesis has a certain degree of flexibility and freedom of design, the design of active targeting systems is complex. Further, the parameters of in vitro preparation do not meet the complex environment in the organism and are susceptible to instability (aggregation, fusion, precipitation, drug leakage) due to their composition, the loaded drug and the environment in which the carrier is located (Weber et al., 2020; Ding et al., 2022). Designing drug carrier systems with better homologous targeting ability, good immunogenicity, and high histocompatibility will be one of the directions to be tackled for future immunotherapy (Wang et al., 2021b).
Nanobased formulations typically undergo a five-step cascade (Su et al., 2017). For each step, the development of drug-carrying particles with the required specific nano-properties allows for responsive drug release and thus better adaptation to the complex environment and diverse biological barriers of tumor tissues, Liposomal systems are being used to carry drug nanoparticles or nucleic acids in new gene therapies (Ermilova and Swenson, 2020). For example, a new nanoparticle, low-density lipoprotein–N-succinyl chitosan–cystamine–urocanic acid (LDL-NSC-SS-UA) could carry both breast cancer resistance protein small interfering RNA (siRNA) and paclitaxel (PTX) for addressing breast cancer multi-drug resistance (Zhu et al., 2017). The excellent biocompatibility of liposomal drugs, the advantages of natural targeting of bionanoplasmic membranes with the advantages of liposomes are promising. Additionally, magnetic targeting and inducing cell death via ferroptosis (iron) are advantages of iron oxide nanoparticles that provide new ideas for the anti-tumor immunization work with nanomaterials.
Drug-Loaded Bionic Membrane Nanosystems
Active targeting properties of drug-loaded bionic membrane nanosystems
In recent years, drug-loaded biomimetic membrane nanosystem has received the attention of researchers. On one hand, cell membranes of organic origin have low immunogenicity and excellent biocompatibility, which can effectively avoid the capture of nanocarriers by the monocyte-macrophage system and reticuloendothelial system, achieve long-lasting circulation in the body, and increase drug accumulation or inflammation. To assess the serum stability and half-life of blood circulation in vivo, a clearance half-life of 15.8 h was found for polyethylene glycolic nanoparticles and up to 39.6 h for nanoparticles (NPs) coated with erythrocyte membranes. On the other hand, specific targeting and homologous targeting capabilities can be obtained with the help of specific recognition units (proteins, polysaccharides, lipids, etc.) on the cell membrane. Currently, the main bionic membranes are erythrocyte membranes, exosome membranes, macrophage membranes, and tumor cell membranes. The nanoparticles modified with bionic membranes also showed cell targeting. Such cell membrane-encapsulated nanoparticles were composed of natural cell membranes with flexible biological properties and functionally integrated nanosystems which are indispensable in biomedical fields. The chemotaxis of some functional cells in the blood and the active recruitment of leukocytes such as neutrophils, and macrophages, allow carriers based on these cell membrane bionics to actively target inflammation and related diseases to bridge the active targeting shortcomings of liposomal drug carriers. For example, bionanoparticles modified with macrophage membranes could exhibit excellent diagnostic and therapeutic effects and drug delivery properties (good tumor targeting, biocompatibility, prolonged in vivo circulation time, and stable liposome structure) (Liang et al., 2020). The use of exosomes, which are cell-derived vesicles has many advantages. Since they originate from their parental cells, they have an innate homing ability due to the presence of homologous surface adhesion proteins, mRNA, lipid molecules, DNA. Further, the expression of the surface transmembrane protein CD47 on the membrane facilitates the avoidance of immune clearance by the mononuclear phagocytic system, prolongs the circulation time of nanomaterials in vivo, and facilitates systemic delivery and targeting (Chen et al., 2016; Ai et al., 2018; Cheng et al., 2021). A novel platelet mimetic dosage form prepared using platelet-loaded photothermal nanoparticles with immune agonists could ameliorate the uneven distribution of agents in phototherapy by exploiting the adhesion mechanisms of platelets. Thermal injury induced further post-injury which led to feedback-based additional platelet recruitment and improved the targeting capacity of the delivery system, thus enabling efficient combined tumor photothermal-immunotherapy in animal experiments (Lv et al., 2021).
Active targeting has also been shown to improve the distribution of NPs within target tissues. The use of targeting peptides overexpressed on breast cancer cells resulted in enhanced penetration and uniform distribution of NPs in a breast cancer mice model (Du et al., 2019). Once the NPs reach the tumor target, to the sufficient release of the drug to achieve the anti-tumor effect is another problem to be solved. Therefore, bio-nanoparticles are being designed to respond to the stimulation of the TME or intracellular signals to achieve rapid and accurate release of anti-tumor drugs at the target site or focal site at high doses. This can enhance their accumulation, thus improving the therapeutic effect of cancer. Common ways in which nanomedicines respond include the organism's environmental factors (such as pH and redox reactions) and external physical stimuli (such as light and heat).
Mechanism of cell killing and immune action of drug-loaded bionic membrane nanosystems
The immune system can recognize and remove cancer cells from the TME. However, cancer cells can survive in various stages of antitumor immune response by suppressing the immune system so that the immune system cannot identify and kill cells, which is called immune escape. Tumor cells may employ multiple immune escape approaches or immunosuppressive mechanisms, such as defection of immune cells, loss or downregulation of MHC expression, and upregulation of various suppressor molecules, including PD-L1, CD47, NK cell ligand, and T cell depletion, ultimately leading to rapid tumor growth (Ahmad et al., 2004; Sade-Feldman et al., 2017; Adams et al., 2015; Liu et al., 2015; Vodnala et al., 2019).
Suppressed immune response in breast cancer patients is not only due to the lack of cytotoxic T cell-associated antigens and helper T cells in the body but also due to the relatively high titer of soluble immunosuppressive molecules produced by tumor cells, resulting in immune tolerance. PD-1 and PD-L1 are involved in the formation of a TME that inhibits the activation of T-cells and the reduction of cytokine production, thereby mediating negative immune regulation. This prompts tumor cells to evade surveillance and killing by the immune system by sending “Don’t find me” signals, and upregulating tumor activity (Konishi et al., 2004; Arasanz et al., 2017). In conclusion, inhibition of PD-1 or PD-L1, thereby enhancing the endogenous anti-tumor immune effect and reducing tumor infiltration and metastasis is important for breast cancer immunotherapy.
A bionic membrane-modified nano-DDS carries immunomodulatory drugs into the body disguised as endogenous substances while retaining the corresponding functions and surface physicochemical properties of the original cell membrane structure. This can avoid immunological recognition such as reducing the uptake of the reticuloendothelial system, improving the anti-immune clearance ability, thus escaping immune clearance and prolonging the circulation time in the organism. It can help the specific targeting and homologous targeting ability with the specific recognition units (proteins, polysaccharides, lipids, etc.) on the bionic membrane, and targeted delivery to the drug target area to regulate immune function (Vijayan et al., 2018; Xie et al., 2019). Tumor cells can induce effector cytotoxic T cell deactivation or apoptosis by transducing “Don’t find me” signals by the high expression of PD-L1 molecules. Bionic membrane-encapsulated NPs target PD-1/PD-L1 inhibitors through active or passive targeting and release PD-L1 inhibitors to block PD-L1 inhibitory signals. This promotes the reactivation of immune responses, upregulates T cell growth and proliferation, enhances T cell recognition of tumors, and thereby reduces tumor load for immunotherapy of breast cancer (Sangro et al., 2021). PD-L1 signaling blockade associated with fibrinogen-like protein 1 (FGL1) gene silencing was found to have a highly synergistic therapeutic effect on breast cancer. FGL1 is overproduced by tumor cells and is critical for the negative regulation of T-cell function, and plasma concentrations of FGL1 are associated with prognosis and chemoresistance. The antitumor assay showed that a hybrid Met-loaded bionic membrane camouflaged Poly (lactic-co-glycolic acid) NP delivery system could effectively alleviate tumor hypoxia and induce M1-type differentiation of tumor-associated macrophages, thereby improving the tumor immunosuppressive microenvironment (Gong et al., 2021).
By transmitting the “Don’t eat me” signal, tumor cells can avoid macrophage phagocytosis through the expression of CD47. CD47 can bind to inhibitory immune receptors and send a “Don’t eat me” signal to the innate immune system, which is the main intrinsic immune escape mechanism of tumor cells. CD47 expressed on cells binds to inhibitory immune receptors on macrophages, which activates tyrosine phosphorylase. This thereby inhibits macrophage phagocytosis of target cells and leads to immune evasion of tumors. CD47 is gradually down-regulated in the process of red blood cell senescence, which eventually leads to phagocytosis and clearance of red blood cells by macrophages (Oldenborg et al., 2000). The CD47 expression of tumor cells is on average about 3-fold higher than normal cell levels. Besides, the anti-CD47 antibody is also known as a “universal antibody” that is expected to be effective against all tumors. The anti-CD47 antibody attaches to the antigen CD47 and blocks it by attaching to the natural receptor, thus blocking the “Don’t eat me” signaling pathway and inducing phagocytosis of tumor cells by macrophages. Thereafter, macrophages present tumor cell antigens to adaptive immune cells hence acting as antigen-presenting cells.
Mechanisms of action and immunotherapeutic effects of responsive drug-loaded bionic membrane nanosystems: response mechanism of photoresponsive bionic membrane nanosystems and anti-tumor immunotherapy
Unlike active drug targeting strategies based on pathophysiology, photo-responsive nanodrug release systems use near-infrared light at a wavelength range of 700–1000 nm to irradiate the target area. This is independent of the heterogeneity of cells, tissue types, and the TME to exhibit more direct and controlled targeting. They are triggered by remote photo-stimuli that are absorbed by photo-responsive molecules on the nanodrug, which subsequently absorb the light and converts it into heat or reactive oxygen clusters inducing the release of the drug (Yang et al., 2017). For example, the total drug release of a nanodrug in a normal environment without 980 nm laser irradiation is less than 14%, while the release of drug is up to 75% in 16 h after 10 min of irradiation of the nanodrug using a 980 nm laser. The photo-responsive nanodrug release system not only has the tunability of external stimulation but also the modulation of the power and duration of light stimulation, which can regulate the degree of ROS production or temperature change. Not only that, the repetitive cycle of local photo-stimulation replaces the repetitive administration of drugs. For example, Shim et al. (2017) constructed a nano DDS triggered by long-range near-infrared light stimulation, where the light stimulus would be absorbed by light-responsive molecules or three-dimensional nanostructures.
The release mechanism of photothermal therapy in responsive drug-loaded bio-nanostructured nanosystems is based on the absorption of light energy and its conversion to thermal energy by the light-absorbing component of the photo-responsive molecular structure. This rise in the local temperature led to a phase transition of the thermosensitive lipid component of the bio-nanostructured membrane that affects the release of the drug, enhancing the spatial and temporal controllability of drug release (Zhang et al., 2011). Drug release further induces ICD to mediate antitumor immunity further, dead cells can secrete DAMPs that can promote significant DC maturation and the activation of specific effector T cells can eliminate tumor cells (Krysko et al., 2012; Kroemer et al., 2013). In a study, Pei et al. (2020) showed that the photothermal conversion efficiency of the NPs were constant after covering the bionic film coating and accelerated the release rate of the drug after laser irradiation of the NPs reaching the target region. The drug could be released in a targeted way, thus eliminating mouse 4T1 breast cancer cells. The drug release mechanism of photodynamic therapy with a responsive drug-loaded bionic membrane nanosystem can selectively enrich the drug-loaded bionic membrane nanosystem carrying photosensitizer in tumor tissues by the targeting advantage of the bionic membrane. After laser-triggered treatment in the target area, the nanosystem can convert oxygen into cell-killing ROS and induce tumor cell necrosis and apoptosis. It can also seal tumor blood vessels and cause vascular damage through oxidative damage to the surrounding organelles and biomolecules, thus realizing the anti-tumor effect in the target area. Thus, photo-switching systems have been employed to make endosomal drug escape active, adjust the chemical and biological drugs release, and control drug release. This strategy would hopefully overcome the limitations of physiological DDS and has important implications for phototherapy-based remote control of drug applications.
Mechanisms of action and immunotherapeutic effects of responsive drug-loaded bionic membrane nanosystems: response mechanism of magnetically responsive bionic membrane nanosystems and anti-tumor immunotherapy
As a novel targeted drug delivery method, a magnetically manipulated targeted system is based on the principle that magnetic nanocarriers can be magnetically resonantly contrasted under strong magnetic fields (MFs). This can generate high heat under alternating high-frequency MFs, and achieve controlled motion under simple low-frequency MF manipulation.
Magnetically responsive nanodrug release systems use MFs as a response stimulus for nanomaterials. The advantage of MFs is their unique properties: they can mediate the targeting of NPs by a constant MF, can heat NPs in vivo by an alternating MF, or combine both to mediate the magnetic response of nanomaterials synergistically. Magnetic nanocarriers can be injected intravenously into the body, modified by bionic membranes to obtain an active targeting effect, and pooled to the tumor site to inhibit tumor growth in combination with magnetic heat or controlled drug release, or both (Sergio et al., 2015).
When placed in an alternating MF, magnetic nanoparticles can absorb energy and convert magnetic energy into heat release due to hysteresis loss or Nier relaxation. Therefore, magnetic nanoparticles are widely used to generate high heat locally in tumors for thermally responsive drug release. The most typical application is to use magnetic nanoparticles as the core, and the outer layer is covered with temperature-sensitive lipids or polymers. Here, the magnetic core generates hyperthermia with the existing alternating MF, and the temperature-sensitive nanoparticle shell undergoes a conformational transition. The drug is encapsulated in the nanoparticle core when no exogenous MF is present, and the superparamagnetic nanoparticles are magnetized when an exogenous MF is applied. Thus, they are mutually compressed to release the encapsulated drug and trigger the rapid drug release (Qin et al., 2009). In one report, Zhang et al. (2017) coated superparamagnetic and magnetically responsive magnetic nanoclusters (MNC) with azide (N3)-modified leukocyte membrane fragments and obtained artificial antigen-presenting cells by the click reaction. This enabled the magnetically responsive nanodrugs to avoid immune clearance with the addition of a bionic membrane. Further effective guidance to the tumor tissue was done under the visual control of MF and magnetic resonance imaging, followed by synergistic cytotoxic effects of magnetic nanoparticles in combination with thermal therapy. Xuan et al. (2018) developed a magnetic mesoporous silica nanoparticle (MMSN) covered with red blood cell (RBC) membrane, which effectively integrated immune adjuvant, photosensitizer delivery and MF-assisted targeted photodynamic therapy. The RBC-based nanoparticle could avoid immune clearance and obtain MF-induced tumor hyperaccumulation in in vivo experiments. When light irradiation was applied, single-linear oxygen loaded with dextran B rapidly generated RBC@MMSNs and led to tumor tissue necrosis. Another strategy controls the drug release rate by controlling the duration of the alternating MF and adjusting the rate of contraction of the temperature-sensitive shell pore size. Satarkar and Zach Hilt (2008) demonstrated the feasibility of triggering drug release when magnetically induced temperatures rise.
In magnetic responsiveness-mediated enhanced nanodrug transport, a research team constructed a liposome connected to magnetic nanostrands, which are linked by DNA and loaded with the drug in the liposome. When the magnetic composite nanocarrier reached a specific site, the magnetic nanostrands oscillated upon application of a radiofrequency field, thereby disrupting the liposome structure and releasing the drug rapidly in large quantities (Peiris et al., 2012). When an MF is coupled to magnetic nanoparticles, the MF can be manipulated to initiate signaling pathways. For example, Cho et al. (2012) developed magnetic nanoparticle magnetic switches capable of initiating apoptotic cells. The magnetic switch was made of a zinc-doped structure, iron oxide magnetic nanoparticles. By controlling the magnetic switch using a remote and noninvasive MF signaling approach, an MF was applied to accumulate nanoparticles to bind DR4s while in its operation. This promoted the apoptotic signaling pathway and caused apoptosis of tumor cells for therapeutic effects. However, there are limitations in MF-mediated targeting, as the MF strength shows a little effect with tissue depth, the magnetic nanocarriers cannot be manipulated at the deep tissues due to their small size and weak magnetic response. Thus, magnetic targeting is limited to the surface or outside of the body. The extracorporeal circulation magnetic manipulation targeting delivery system directs blood flow outside the body and reduces the distance between the magnetic nanoparticles and MF, which favors achieving magnetic manipulation. Therefore, researchers have shifted their research on magnetic nanoparticles toward iron oxide nanoparticle systems and further investigate their anti-tumor immune effects.
Drug-Loaded Iron Oxide Nanosystems
Iron oxide nanoparticles (IONPs) are a series of iron oxide nanomaterials such as iron oxides and hydroxyl oxides (Xu et al., 2012), mainly FeO, Fe2O3, and Fe3O4. Among them, Fe3O4 nanoparticles have been approved by the FDA for clinical treatment in iron deficiency anemia (Soetaert et al., 2020), as they increase the hemoglobin content making them the first generation of nanomaterials to be used in the clinic (Frtús et al., 2020). On the one hand, Fe3O4 nanoparticles are a cure for iron deficiency anemia. On the other hand, Fe3O4 nanoparticles are prominently effective in the treatment of tumors. Recent publications explained that IONPs not only produce thermal effects through the Fenton reaction and laser irradiation but also participate in immune regulation through the ferroptosis pathway and act on tumor-associated immune cells. This brings new opportunities to further promote the research of IONPs in clinical practice.
Mechanism of cell killing and immune action of drug-loaded iron oxide nanosystems
Ferroptosis is an interesting mode of cell death caused by the massive accumulation of regulated iron ion-dependent forms of lipid ROS (Dixon et al., 2012). It regulates tumor development through mechanisms such as lipid peroxidation, iron metabolism, and amino acid metabolism (Jiang et al., 2021). Ferroptosis can drive cell death through pathways that promote the Fenton reaction, GSH synthesis, and polyunsaturated fatty acid metabolism (Dolma et al., 2003). In one study, ferumoxytol iron oxide nanoparticles were used to induce chemokine-mediated recruitment of monocytes into breast cancer cells. They polarized to an anti-inflammatory M2 phenotype, and subsequently induced to a pro-inflammatory M1 phenotype, which could trigger apoptosis via the Fenton response pathway (Zanganeh et al., 2016). Triple-negative breast cancer was reported to be more sensitive to ferroptosis due to its iron-rich death gene profile (Verma et al., 2020). Therefore, immunotherapy has become a popular therapy for malignancy treatment in recent years.
Immune cells, a key to immunotherapy, can participate in their mediated anti-tumor immunity through ferroptosis. Among them, CD8+ T cells can enhance the sensitivity of breast cancer to ferroptosis by releasing interferon gamma (IFNγ) to promote T cell-mediated antitumor immunity (Wang et al., 2019). In addition to conventional therapies, immunotherapy combined with ferroptosis can also achieve good performance in breast cancer treatment. Mimetic Fe3O4 magnetic vesicles (Pa-M/Ti-NC) camouflaged by the leukocyte membrane could create an immunogenic microenvironment as a basis to increase the intracellular H2O2 content. This could acquire a large amount of Fe2+ by reduction to promote the ferroptosis of tumor cells (Zhang et al., 2019), thus achieving the anti-tumor ferroptosis synergistic immunotherapy.
T cells are significant mediators of antitumor immunity, and ferroptosis is involved in the killing process against tumors. Thus, regulating the ferroptosis pathway contributes to enhancing the antitumor effects of immunotherapy. Mechanistically, immunotherapy-activated CD8+ T cells downregulated the expression of Xc-, SLC3A2, and SLC7A11, by secreting IFNγ, thereby achieving reduced uptake of cystine by tumor cells to induce lipid peroxidation and ferroptosis in tumor cells (Wang et al., 2019). In contrast, a bionic magnetic nanoplatform (Fe3O4SAS@PLT) constructed by Jiang et al. (2020) could not only induce an effective immune response by inhibiting System xc-effective triggering of cystine into cells to synthesize GSH for ferroptosis of tumor cells, and also induce an effective immune response. In summary, the ferroptosis pathway in breast cancer cells is regulated by T cells and is involved in T cell-mediated antitumor immunity. Hence, targeting the ferroptosis pathway might be a promising approach for future tumor therapy. Tumor cells undergoing ferroptosis can release a variety of immunostimulatory signals (Tang et al., 2021) that can activate DCs and macrophages (Xu et al., 2021). Moreover, these signals can also accelerate the maturation of DCs and increase the phagocytosis of ferroptosis-induced dead cancer cells by macrophages. Luo et al. (2021) identified an important phagocytic signal, 1-steaoryl-2-15-HpETE-sn-glycero-3-PE, on ferroptosis-induced dead cells, which could enhance the phagocytic efficacy of macrophages (Luo et al., 2021). To summarize, ferroptosis-induced cancer cells can enhance phagocytosis by releasing immunostimulatory signals to promote immune cell maturation and identify tumor cells (Lei et al., 2022).
Mechanism of action and immunotherapeutic effects of responsive drug-loaded iron oxide nanosystems: response mechanism of thermally responsive iron oxide nanosystems and antitumor immunotherapy
Although ferroptosis is widely used as a new approach to cell death mode in cancer therapy, the cell death immunogenicity during ferroptosis still needs to be improved. Based on the fact that temperature modulation of antioxidants can also make tumors more sensitive to ferroptosis through its pathway, this approach can achieve better therapeutic effects. For example, Xie et al. (2021) designed a co-encapsulated system (GBP@Fe3O4) of targeted peptide modified, liquid 1H-perfluoropentane (1H-PFP) and Fe3O4 nanoparticles. They proposed a new strategy of thermally triggered tumor-specific ferroptosis, which allows Fe3O4 to generate strong ROS through the Fenton reaction in a moderately hot (45°C) environment to amplify oxidative damage, thus inducing tumor ferroptosis and achieving sufficient anti-tumor effects. This exogenous heat-triggered ferroptosis-based strategy has been successfully implemented on kidney cancer and breast cancer cell lines (Xie et al., 2021). Thus, such a treatment method is proposed as a new idea for cancer treatment. To extend the in vivo circulation time of Fe3O4 nanomicrospheres, wrapping them with erythrocyte membranes could effectively promote the enrichment of the composite microspheres, significantly enhancing the photothermal therapy effect in the animal model (Peng et al., 2017). Another popular method for treating malignancies is chemo-dynamic therapy (CDT) based on the Fenton reaction. A novel therapeutic nanomedicine called PTCGNPs was reacted with H2O2 to synthesize ROS through an iron-based Fenton reaction, resulting in a chemical/chemical kinetic combination (Ren et al., 2020). Single thermos-responsive type can be commonly used as a stand-alone carrier for controlled drug release. However, multiple stimuli-responsive drug carriers possess better specificity and flexibility. Another research team proposed a pH/thermosensitive dual-responsive nanotherapeutic system with excellent delivery capability. A pH/thermosensitive gel containing IONPs and adriamycin (Doxorubicin, Dox) was to produce local thermal therapy which accelerated drug release that irreversibly damaged the tumor, making tumor cells more sensitive to chemotherapy. Such research for increasing drug delivery and strengthening tumor combination therapy has gained increasing attention (Liu et al., 2022). In another study, Zhu et al. (2020) used an external heat source (near-infrared light, NIR) to load Comprisil A4 (Azo-CA4) into upconversion nanoparticles (UCNPs) for enhancing the release of chemicals from nanoparticles. AzoCA4-treated cells displayed isomerization following NIR light exposure led to microtubule breaking in UCNPs, releasing Azo-CA4 and enabling its function. A combination of NIR and UV conversion could also cause the reduction of Fe3+ to Fe2+ in UCNPs, which increased lipid peroxidation and caused ferroptosis in tumor cells, thus emerging as a synergistic anti-tumor approach.
Mechanism of action and immunotherapeutic effects of responsive drug-loaded iron oxide nanosystems: response mechanism of magnetically responsive iron oxide nanosystems and anti-tumor immunotherapy
The magnetic responsive DDS is a novel DDS designed on the basis of characteristics and “magnetic responsiveness” of the tumor tissue microenvironment and cell structure. The magnetically responsive nanosystem delivers the exogenous drug to a predetermined location, where the drug it carries is enriched to reduce its toxic side effects on healthy tissues. This helps achieve efficient transport and reduce toxicity, with the significant advantage of fast response time compared to other stimulus-responsive systems.
Using superparamagnetic Fe3O4 as a magnetically responsive drug carrier has advantages of low cytotoxicity, easy formation of superparamagnetic properties, and good stability (Zheng et al., 2018). A research team developed bovine serum albumin (Fe3O4/BSA) particles loaded with iron oxide nanoparticles that could internalize bone marrow mesenchymal stem cells (MSCs), thereby significantly reducing the release rate of MSCs in a static MF. The uptake of Fe3O4/BSA particles significantly increased the osteogenic differentiation of MSCs under constant static MF conditions, suggesting that magnetically manipulated stem cells induced differentiation (Jiang et al., 2016). The magnetic control method has free release control of nanomedicine, improves drug release, and increases mechanical lethality against tumors. In contrast to the pH and redox stimulation triggering drug release, magnetic control achieves external stimulation. With further study, magnetic control can achieve external and internal sensitive responses to tumor treatment.
To decrease drug resistance, Yao et al. (2020) used Zn0.2Fe2.8O4 magnetic nanoparticles with a strong magnetic response type and the anticancer drug adriamycin (DOX), co-carried in poly (lactic acid)-glycolic acid copolymer. The release of the chemical agent within it through the action of an external MF, resulted in a dual magnetic-chemical combination killing effect on tumor cells.
Nanomedicines are usually effective in the body through five processes: blood circulation, tumor accumulation, deep tumor tissue penetration, cellular internalization, and intracellular drug release. Therefore, based on these processes, the development of drug-loaded responsive nanoparticles can adapt to the complex environment and diverse biological barriers of tumor tissues to accomplish drug release efficiency. However, there are still unresolved challenges in this field, such as the effectiveness of active targeting of drug-loaded liposomal nanoparticles, which is still limited by the complexity of in vivo pathophysiology. Whether the interaction with the immune system after membrane modification by bionic membrane-loaded nanoparticles induces or exacerbates inflammation, which leads to the release of pathological inflammatory mediators is also not known. The less studied ferroptosis of drug-loaded iron oxide nanoparticles in targeting immune cells still needs further exploration.
Funding Statement: The work was funded by the Basic Scientific Research Funds of Department of Education of Zhejiang Province (KYQN202103 and KYZD202103), A Project Supported by Scientific Research Fund of Zhejiang Provincial Education Department (Y202249203), General Program of the National Natural Science Foundation of China (61976075 to XX), the Key Research and Development Program of Zhejiang Province (2019C03002 to XX), National Innovation and Entrepreneurship Training Program for College Students (202213023011), Innovation and Entrepreneurship Training Program for College Students of Zhejiang Province (S202213023052), Zhejiang Provincial Natural Science Foundation of China under Grant No. LTGY23H180019.
Author Contributions: The authors confirm contribution to the paper as follows: Drug-loaded liposome nanosystems section: LETIAN JIN; Drug-loaded iron oxide nanosystems section: HETING CHEN; Drug-loaded bionic membrane nanosystems section: QI RUAN; Drafting introduction and drawing the mechanism graphic: RUI LIU; Revising the drug-loaded iron oxide nanosystems section and critically revise the conclusion: YIFENG FAN. Drafting the abstract and keywords, Funding support: XIUFANG XU; Determine the subject, organize ideas and revise manuscript: DAJIANG WANG, JIAHUI LU. All authors reviewed the results and approved the final version of the manuscript.
Conflicts of Interest: The authors declare that they have no conflicts of interest to report regarding the present study.
References
Adams JL, Smothers J, Srinivasan R, Hoos A (2015). Big opportunities for small molecules in immuno-oncology. Nature Reviews Drug Discovery 14: 603–622. https://doi.org/10.1038/nrd4596 [Google Scholar] [PubMed] [CrossRef]
Aghanejad A, Babamiri H, Adibkia K, Barar J, Omidi Y (2018). Mucin-1 aptamer-armed superparamagnetic iron oxide nanoparticles for targeted delivery of doxorubicin to breast cancer cells. BioImpacts 8: 117–127. https://doi.org/10.15171/bi.2018.14 [Google Scholar] [PubMed] [CrossRef]
Ahmad M, Rees RC, Ali SA (2004). Escape from immunotherapy: Possible mechanisms that influence tumor regression/progression. Cancer Immunology Immunotherapy 53: 844–854. https://doi.org/10.1007/s00262-004-0540-x [Google Scholar] [PubMed] [CrossRef]
Ai X, Hu M, Wang Z, Zhang W, Li J, Yang H, Lin J, Xing B (2018). Recent advances of membrane-cloaked nanoplatforms for biomedical applications. Bioconjugate Chemistry 29: 838–851. https://doi.org/10.1021/acs.bioconjchem.8b00103 [Google Scholar] [PubMed] [CrossRef]
Arasanz H, Gato-Cañas M, Zuazo M, Ibañez-Vea M, Breckpot K, Kochan G, Escors D (2017). PD1 signal transduction pathways in T cells. Oncotarget 8: 51936–51945. https://doi.org/10.18632/oncotarget.17232 [Google Scholar] [PubMed] [CrossRef]
Budd GT, Barlow WE, Moore HC, Hobday TJ, Stewart JA, Isaacs C, Salim M, Cho JK, Rinn KJ, Albain KS (2015). SWOG S0221: A phase III trial comparing chemotherapy schedules in high-risk early-stage breast cancer. Journal of Clinical Oncology 33: 58–64. https://doi.org/10.1200/JCO.2014.56.3296 [Google Scholar] [PubMed] [CrossRef]
Chaudhari D, Katari O, Ghadi R, Kuche K, Date T, Bhargavi N, Jain S (2022). Unfolding the potency of adenosine in targeting triple negative breast cancer via paclitaxel-incorporated pH-responsive stealth liposomes. ACS Biomaterials Science & Engineering 8: 3473–3484. https://doi.org/10.1021/acsbiomaterials.2c00594 [Google Scholar] [PubMed] [CrossRef]
Chen Y, Wan Y, Wang Y, Zhang H, Jiao Z (2011). Anticancer efficacy enhancement and attenuation of side effects of doxorubicin with titanium dioxide nanoparticles. International Journal of Nanomedicine 6: 2321. https://doi.org/10.1016/j.fct.2010.04.007 [Google Scholar] [CrossRef]
Chen Z, Zhao P, Luo Z, Zheng M, Tian H et al. (2016). Cancer cell membrane-biomimetic nanoparticles for homologous-targeting dual-modal imaging and photothermal therapy. ACS Nano 10: 10049–10057. https://doi.org/10.1021/acsnano.6b04695 [Google Scholar] [PubMed] [CrossRef]
Cheng L, Zhang X, Tang J, Lv Q, Liu J (2021). Gene-engineered exosomes-thermosensitive liposomes hybrid nanovesicles by the blockade of CD47 signal for combined photothermal therapy and cancer immunotherapy. Biomaterials 275: 120964. https://doi.org/10.1016/j.biomaterials.2021.120964 [Google Scholar] [PubMed] [CrossRef]
Cho MH, Lee EJ, Son M, Lee JH, Yoo D, Kim JW, Park SW, Shin JS, Cheon J (2012). A magnetic switch for the control of cell death signalling in vitro and in vivo systems. Nature Materials 11: 1038–1043. https://doi.org/10.1038/nmat3430 [Google Scholar] [PubMed] [CrossRef]
Curcio M, Brindisi M, Cirillo G, Frattaruolo L, Leggio A, Rago V, Nicoletta FP, Cappello AR, Iemma F (2022). Smart lipid-polysaccharide nanoparticles for targeted delivery of doxorubicin to breast cancer cells. International Journal of Molecular Sciences 23: 2386–2393. https://doi.org/10.3390/ijms23042386 [Google Scholar] [PubMed] [CrossRef]
Dastmalchi N, Safaralizadeh R, Baghbanzadeh A, Hajiasgharzadeh K, Asl ER, Amini M, Baradaran B (2020). Molecular mechanisms of breast cancer chemoresistance by immune checkpoints. Life Sciences 263: 118604. https://doi.org/10.1016/j.lfs.2020.118604 [Google Scholar] [PubMed] [CrossRef]
de Oliveira Silva J, Fernandes RS, Oda CMR, Ferreira TH, Botelho AFM, Melo MM, de Miranda MC, Gomes DA, Cassali GD, Townsend DM (2019). Folate-coated, long-circulating and pH-sensitive liposomes enhance doxorubicin antitumor effect in a breast cancer animal model. Biomedicine & Pharmacotherapy 118: 109323. https://doi.org/10.1016/j.biopha.2019.109323 [Google Scholar] [PubMed] [CrossRef]
Ding L, Li H, Wang Y (2021). Application of Jianpi Xiaoai recipe combined with cisplatin and adriamycin in the treatment of endometrial cancer and its effect on disease control rate. Evidence-Based Complementary and Alternative Medicine 2021. https://doi.org/10.1155/2021/2258183 [Google Scholar] [PubMed] [CrossRef]
Ding Y, Wang L, Li H, Miao F, Zhang Z, Hu C, Yu W, Tang Q, Shao G (2022). Application of lipid nanovesicle drug delivery system in cancer immunotherapy. Journal of Nanobiotechnology 20: 1–22. https://doi.org/10.1186/s12951-022-01429-2 [Google Scholar] [PubMed] [CrossRef]
Dixon SJ, Lemberg KM, Lamprecht MR, Skouta R, Zaitsev EM et al. (2012). Ferroptosis: An iron-dependent form of nonapoptotic cell death. Cell 149: 1060–1072. https://doi.org/10.1016/j.cell.2012.03.042 [Google Scholar] [PubMed] [CrossRef]
Dolma S, Lessnick SL, Hahn WC, Stockwell BR (2003). Identification of genotype-selective antitumor agents using synthetic lethal chemical screening in engineered human tumor cells. Cancer Cell 3: 285–296. https://doi.org/10.1016/S1535-6108(03)00050-3 [Google Scholar] [PubMed] [CrossRef]
Du Y, Liu X, Liang Q, Liang XJ, Tian J (2019). Optimization and design of magnetic ferrite nanoparticles with uniform tumor distribution for highly sensitive MRI/MPI performance and improved magnetic hyperthermia therapy. Nano Letters 19: 3618–3626. https://doi.org/10.1021/acs.nanolett.9b00630 [Google Scholar] [PubMed] [CrossRef]
Effimia C (2021). Regulated cell death pathways in doxorubicin-induced cardiotoxicity. Cell Death and Disease 12: 339. https://doi.org/10.1038/s41419-021-03614-x [Google Scholar] [PubMed] [CrossRef]
Ermilova I, Swenson J (2020). DOPC versus DOPE as a helper lipid for gene-therapies: Molecular dynamics simulations with DLin-MC3-DMA. Physical Chemistry Chemical Physics 22: 28256–28268. https://doi.org/10.1039/D0CP05111J [Google Scholar] [PubMed] [CrossRef]
Fraguas-Sánchez AI, Lozza I, Torres-Suárez AI (2022). Actively targeted nanomedicines in breast cancer: From pre-clinal investigation to clinic. Cancers 14: 1198. https://doi.org/10.3390/cancers14051198 [Google Scholar] [PubMed] [CrossRef]
Frtús A, Smolková B, Uzhytchak M, Lunova M, Jirsa M, Kubinová Š., Dejneka A, Lunov O (2020). Analyzing the mechanisms of iron oxide nanoparticles interactions with cells: A road from failure to success in clinical applications. Journal of Controlled Release 328: 59–77. https://doi.org/10.1016/j.jconrel.2020.08.036 [Google Scholar] [PubMed] [CrossRef]
Gao Y, Jia L, Wang Q, Hu H, Zhao X, Chen D, Qiao M (2019). pH/Redox dual-responsive polyplex with effective endosomal escape for codelivery of siRNA and doxorubicin against drug-resistant cancer cells. ACS Applied Materials & Interfaces 11: 16296–16310. https://doi.org/10.1021/acsami.9b02016 [Google Scholar] [PubMed] [CrossRef]
Gao J, Yuan X, Yuan J, Li L (2021). Complete rejection of large established breast cancer by local immunochemotherapy with T cell activation against neoantigens. Cancer Immunology Immunotherapy 70: 3291–3302. https://doi.org/10.1007/s00262-021-02919-2 [Google Scholar] [PubMed] [CrossRef]
Gong C, Yu X, Zhang W, Han L, Wang R, Wang Y, Gao S, Yuan Y (2021). Regulating the immunosuppressive tumor microenvironment to enhance breast cancer immunotherapy using pH-responsive hybrid membrane-coated nanoparticles. Journal of Nanobiotechnology 19: 58. https://doi.org/10.1186/s12951-021-00805-8 [Google Scholar] [PubMed] [CrossRef]
Green H, Stål O, Bachmeier K, Bäcklund L, Carlsson L, Hansen J, Lagerlund M, Norberg B, Franzén Å., Åleskog A (2011). Pegylated liposomal doxorubicin as first-line monotherapy in elderly women with locally advanced or metastatic breast cancer: Novel treatment predictive factors identified. Cancer Letters 313: 145–153. https://doi.org/10.1016/j.canlet.2011.07.017 [Google Scholar] [PubMed] [CrossRef]
Gu Z, da Silva CG, van der Maaden K, Ossendorp F, Cruz LJ (2020). Liposome-based drug delivery systems in cancer immunotherapy. Pharmaceutics 12: 1054–1079. https://doi.org/10.3390/pharmaceutics12111054 [Google Scholar] [PubMed] [CrossRef]
Inglut CT, Sorrin AJ, Kuruppu T, Vig S, Cicalo J, Ahmad H, Huang HC (2020). Immunological and toxicological considerations for the design of liposomes. Nanomaterials 10: 190. https://doi.org/10.3390/nano10020190 [Google Scholar] [PubMed] [CrossRef]
Jiang X, Stockwell BR, Conrad M (2021). Ferroptosis: Mechanisms, biology and role in disease. Nature Reviews Molecular Cell Biology 22: 266–282. https://doi.org/10.1038/s41580-020-00324-8 [Google Scholar] [PubMed] [CrossRef]
Jiang Q, Wang K, Zhang X, Ouyang B, Liu H, Pang Z, Yang W (2020). Platelet membrane-camouflaged magnetic nanoparticles for ferroptosis-enhanced cancer immunotherapy. Small 16: e2001704. https://doi.org/10.1002/smll.202001704 [Google Scholar] [PubMed] [CrossRef]
Jiang P, Zhang Y, Zhu C, Zhang W, Mao Z, Gao C (2016). Fe3O4/BSA particles induce osteogenic differentiation of mesenchymal stem cells under static magnetic field. Acta Biomaterialia 46: 141–150. https://doi.org/10.1016/j.actbio.2016.09.020 [Google Scholar] [PubMed] [CrossRef]
Jin Q, Liu Z, Chen Q (2021). Controlled release of immunotherapeutics for enhanced cancer immunotherapy after local delivery. Journal of Controlled Release 329: 882–893. https://doi.org/10.1016/j.jconrel.2020.10.019 [Google Scholar] [PubMed] [CrossRef]
Karanth H, Murthy R (2007). pH-sensitive liposomes-principle and application in cancer therapy. Journal of Pharmacy and Pharmacology 59: 469–483. https://doi.org/10.1211/jpp.59.4.0001 [Google Scholar] [PubMed] [CrossRef]
Kim J, Choi Y, Yang S, Lee J, Choi J, Moon Y, Kim J, Shim N, Cho H, Shim MK (2022). Sustained and long-term release of doxorubicin from PLGA nanoparticles for eliciting anti-tumor immune responses. Pharmaceutics 14: 474. https://doi.org/10.3390/pharmaceutics14030474 [Google Scholar] [PubMed] [CrossRef]
Kong F, Liang Z, Luan D, Liu X, Xu K, Tang B (2016). A glutathione (GSH)-responsive near-infrared (NIR) theranostic prodrug for cancer therapy and imaging. Analytical Chemistry 88: 6450–6456. https://doi.org/10.1021/acs.analchem.6b01135 [Google Scholar] [PubMed] [CrossRef]
Konishi J, Yamazaki K, Azuma M, Kinoshita I, Dosaka-Akita H, Nishimura M (2004). B7-H1 expression on non-small cell lung cancer cells and its relationship with tumor-infiltrating lymphocytes and their PD-1 expression. Clinical Cancer Research 10: 5094–5100. https://doi.org/10.1158/1078-0432.CCR-04-0428 [Google Scholar] [PubMed] [CrossRef]
Kroemer G, Galluzzi L, Kepp O, Zitvogel L (2013). Immunogenic cell death in cancer therapy. Annual Review of Immunology 31: 51–72. https://doi.org/10.1146/annurev-immunol-032712-100008 [Google Scholar] [PubMed] [CrossRef]
Krysko DV, Garg AD, Kaczmarek A, Krysko O, Agostinis P, Vandenabeele P (2012). Immunogenic cell death and DAMPs in cancer therapy. Nature Reviews Cancer 12: 860–875. https://doi.org/10.1038/nrc3380 [Google Scholar] [PubMed] [CrossRef]
Lei G, Zhuang L, Gan B (2022). Targeting ferroptosis as a vulnerability in cancer. Nature Reviews Cancer 22: 381–396. https://doi.org/10.1038/s41568-022-00459-0 [Google Scholar] [PubMed] [CrossRef]
Li Z, Ma P, Lai X, Luo Z, Chen Y, Loh XJ, Ye E, Wu C, Wu YL (2022). Recent advances on mechanical force-responsive drug delivery systems. Nanoscale Advances 8: 3462–3478. https://doi.org/10.1039/d2na00420h [Google Scholar] [PubMed] [CrossRef]
Liang B, Deng T, Li J, Ouyang X, Na W, Deng D (2020). Biomimetic theranostic strategy for anti-metastasis therapy of breast cancer via the macrophage membrane camouflaged superparticles. Materials Science & Engineering C: 115: 1091–1109. https://doi.org/10.1016/j.msec.2020.111097 [Google Scholar] [PubMed] [CrossRef]
Lin X, He T, Tang R, Li Q, Wu N et al. (2022). Biomimetic nanoprobe-augmented triple therapy with photothermal, sonodynamic and checkpoint blockade inhibits tumor growth and metastasis. Journal of Nanobiotechnology 20: 80–101. https://doi.org/10.1186/s12951-022-01287-y [Google Scholar] [PubMed] [CrossRef]
Liu Y, Bravo KMC, Liu J (2021). Targeted liposomal drug delivery: A nanoscience and biophysical perspective. Nanoscale Horizons 6: 78–94. https://doi.org/10.1039/D0NH00605J [Google Scholar] [PubMed] [CrossRef]
Liu D, Chen B, Mo Y, Wang Z, Qi T, Zhang Q, Wang Y (2019a). Redox-activated porphyrin-based liposome remote-loadedwith IDO inhibitor for synergistic photoimmunotherapy through induction of immunogenic cell death and blockage of IDO pathway. Nano Letters 19: 6964–6976. https://doi.org/10.1021/acs.nanolett.9b02306 [Google Scholar] [PubMed] [CrossRef]
Liu Y, Chen XG, Yang P-P, Qiao ZY, Wang H (2019b). Tumor microenvironmental pH and enzyme dual responsive polymer-liposomes for synergistic treatment of cancer immuno-chemotherapy. Biomacromolecules 20: 882–892. https://doi.org/10.1021/acs.biomac.8b01510 [Google Scholar] [PubMed] [CrossRef]
Liu X, Pu Y, Cron K, Deng L, Kline J, Frazier WA, Xu H, Peng H, Fu YX, Xu MM (2015). CD47 blockade triggers T cell-mediated destruction of immunogenic tumors. Nature Medicine 21: 1209–1215. https://doi.org/10.1038/nm.3931 [Google Scholar] [PubMed] [CrossRef]
Liu N, Wu L, Zuo W, Lin Q, Liu J et al. (2022). pH/thermal-sensitive nanoplatform capable of on-demand specific release to potentiate drug delivery and combinational hyperthermia/chemo/chemodynamic therapy. ACS Applied Materials & Interfaces 14: 29668–29678. https://doi.org/10.1021/acsami.2c09685 [Google Scholar] [PubMed] [CrossRef]
Lugert S, Unterweger H, Mühlberger M, Janko C, Draack S, Ludwig F, Eberbeck D, Alexiou C, Friedrich RP (2019). Cellular effects of paclitaxel-loaded iron oxide nanoparticles on breast cancer using different 2D and 3D cell culture models. International Journal of Nanomedicine 14: 161–180. https://doi.org/10.2147/IJN.S187886 [Google Scholar] [PubMed] [CrossRef]
Luo X, Gong HB, Gao HY, Wu YP, Sun WY et al. (2021). Oxygenated phosphatidylethanolamine navigates phagocytosis of ferroptotic cells by interacting with TLR2. Cell Death and Differentiation 28: 1971–1989. https://doi.org/10.1038/s41418-020-00719-2 [Google Scholar] [PubMed] [CrossRef]
Luo S, Ma D, Wei R, Yao W, Pang X, Wang Y, Xu X, Wei X, Guo Y, Jiang X (2022). A tumor microenvironment responsive nanoplatform with oxidative stress amplification for effective MRI-based visual tumor ferroptosis. Acta Biomaterialia 138: 518–527. https://doi.org/10.1016/j.actbio.2021.11.007 [Google Scholar] [PubMed] [CrossRef]
Lv Y, Li F, Wang S, Lu G, Bao W, Wang Y, Tian Z, Wei W, Ma G(2021). Near-infrared light-triggered platelet arsenal for combined photothermal-immunotherapy against cancer. Science Advances 7: eabd7614. https://doi.org/10.1126/sciadv.abd7614 [Google Scholar] [PubMed] [CrossRef]
Meng Q, Hu H, Zhou L, Zhang Y, Yu B, Shen Y, Cong H (2019). Logical design and application of prodrug platforms. Polymer Chemistry 10: 306–324. https://doi.org/10.1039/C8PY01160E [Google Scholar] [CrossRef]
Mirhadi E, Mashreghi M, Maleki MF, Alavizadeh SH, Arabi L, Badiee A, Jaafari MR (2020). Redox-sensitive nanoscale drug delivery systems for cancer treatment. International Journal of Pharmaceutics 589: 119882. https://doi.org/10.1016/j.ijpharm.2020.119882 [Google Scholar] [PubMed] [CrossRef]
Mishchenko T, Mitroshina E, Balalaeva I, Krysko O, Vedunova M, Krysko DV (2019). An emerging role for nanomaterials in increasing immunogenicity of cancer cell death. Biochimica et Biophysica Acta (BBA)-Reviews on Cancer 1871: 99–108. https://doi.org/10.1016/j.bbcan.2018.11.004 [Google Scholar] [PubMed] [CrossRef]
Moammeri A, Abbaspour K, Zafarian A, Jamshidifar E, Motasadizadeh H, Dabbagh Moghaddam F, Salehi Z, Makvandi P, Dinarvand R (2022). pH-responsive, adorned nanoniosomes for codelivery of cisplatin and epirubicin: Synergistic treatment of breast cancer. ACS Applied Bio Materials 5: 675–690. https://doi.org/10.1021/acsabm.1c01107 [Google Scholar] [PubMed] [CrossRef]
Mu QG, Lin G, Jeon M, Wang H, Chang FC, Revia RA, Yu J, Zhang M (2021). Iron oxide nanoparticle targeted chemo-immunotherapy for triple negative breast cancer. Mater Today 50: 149–169. https://doi.org/10.1016/j.mattod.2021.08.002 [Google Scholar] [PubMed] [CrossRef]
Naziris N, Saitta F, Chrysostomou V, Libera M, Trzebicka B, Fessas D, Pispas S, Demetzos C (2020). pH-responsive chimeric liposomes: From nanotechnology to biological assessment. International Journal of Pharmaceutics 574: 118849. https://doi.org/10.1016/j.ijpharm.2019.118849 [Google Scholar] [PubMed] [CrossRef]
Nikolos F, Hayashi K, Hoi XP, Alonzo ME, Mo Q, Kasabyan A, Furuya H, Trepel J, di Vizio D, Guarnerio J (2022). Cell death-induced immunogenicity enhances chemoimmunotherapeutic response by converting immune-excluded into T-cell inflamed bladder tumors. Nature Communications 13: 1–16. https://doi.org/10.1038/s41467-022-29026-9 [Google Scholar] [PubMed] [CrossRef]
Norioka C, Okita K, Mukada M, Kawamura A, Miyata T (2017). Biomolecularly stimuli-responsive tetra-poly (ethylene glycol) that undergoes sol-gel transition in response to a target biomolecule. Polymer Chemistry 8: 6378–6385. https://doi.org/10.1039/C7PY01370A [Google Scholar] [CrossRef]
Oldenborg PA, Zheleznyak A, Fang YF, Lagenaur CF, Gresham HD, Lindberg FP (2000). Role of CD47 as a marker of self on red blood cells. Science 288: 2051–2054. https://doi.org/10.1126/science.288.5473.2051 [Google Scholar] [PubMed] [CrossRef]
Oltolina F, Peigneux A, Colangelo D, Clemente N, D'Urso A, Valente G, Iglesias GR, Jiménez-Lopez C, Prat M (2020). Biomimetic magnetite nanoparticles as targeted drug nanocarriers and mediators of hyperthermia in an experimental cancer model. Cancers 12: 2564–2589. https://doi.org/10.3390/cancers12092564 [Google Scholar] [PubMed] [CrossRef]
Omolo CA, Megrab NA, Kalhapure RS, Agrawal N, Jadhav M, Mocktar C, Rambharose S, Maduray K, Nkambule B, Govender T (2021). Liposomes with pH responsive ‘on and off’ switches for targeted and intracellular delivery of antibiotics. Journal of Liposome Research 31: 45–63. https://doi.org/10.1080/08982104.2019.1686517 [Google Scholar] [PubMed] [CrossRef]
Pan P, Dong X, Chen Y, Ye JJ, Sun YX, Zhang XZ (2022). A heterogenic membrane-based biomimetic hybrid nanoplatform for combining radiotherapy and immunotherapy against breast cancer. Biomaterials 289: 121810–121821. https://doi.org/10.1016/j.biomaterials.2022.121810 [Google Scholar] [PubMed] [CrossRef]
Pandrangi SL, Chittineedi P, Chalumuri SS, Meena AS, Neira Mosquera JA, Sánchez Llaguno SN, Pamuru RR, Mohiddin GJ, Mohammad A (2022). Role of intracellular iron in switching apoptosis to ferroptosis to target therapy-resistant cancer stem cells. Molecules 27: 3011. https://doi.org/10.3390/molecules27093011 [Google Scholar] [PubMed] [CrossRef]
Paridaens R, Biganzoli L, Bruning P, Klijn J, Gamucci T, Houston S, Coleman R, Schachter J, van Vreckem A, Sylvester R (2000). Paclitaxel versus doxorubicin as first-line single-agent chemotherapy for metastatic breast cancer: A European organization for research and treatment of cancer randomized study with cross-over. Journal of Clinical Oncology 18: 724–724. https://doi.org/10.1200/JCO.2000.18.4.724 [Google Scholar] [PubMed] [CrossRef]
Pei W, Huang B, Chen S, Wang L, Xu Y, Niu C (2020). Platelet-mimicking drug delivery nanoparticles for enhanced chemo-photothermal therapy of breast cancer. International Journal of Nanomedicine 15: 10151–10167. https://doi.org/10.2147/IJN.S285952 [Google Scholar] [PubMed] [CrossRef]
Peiris PM, Bauer L, Toy R, Tran E, Pansky J et al. (2012). Enhanced delivery of chemotherapy to tumors using a multicomponent nanochain with radio-frequency-tunable drug release. ACS Nano 6: 4157–4168. https://doi.org/10.1021/nn300652p [Google Scholar] [PubMed] [CrossRef]
Peng H, Tang J, Zheng R, Guo G, Dong A, Wang Y, Yang W (2017). Nuclear-targeted multifunctional magnetic nanoparticles for photothermal therapy. Advanced Healthcare Materials 6: 1601289. https://doi.org/10.1002/adhm.201601289 [Google Scholar] [PubMed] [CrossRef]
Piehler S, Dähring H, Grandke J, Göring J, Couleaud P et al. (2020). Iron oxide nanoparticles as carriers for DOX and magnetic hyperthermia after intratumoral application into breast cancer in mice: Impact and future perspectives. Nanomaterials 10: 1016–1023. https://doi.org/10.3390/nano10061016 [Google Scholar] [PubMed] [CrossRef]
Qin J, Asempah I, Laurent S, Fornara A, Muller RN, Mamoun M (2009). Injectable superparamagnetic ferrogels for controlled release of hydrophobic drugs. Advanced Materials 21: 1354–1357. https://doi.org/10.1002/adma.200800764 [Google Scholar] [CrossRef]
Raju GSR, Pavitra E, Varaprasad GL, Bandaru SS, Nagaraju GP, Farran B, Huh YS, Han YK (2022). Nanoparticles mediated tumor microenvironment modulation: Current advances and applications. Journal of Nanobiotechnology 20: 274–294. https://doi.org/10.1186/s12951-022-01476-9 [Google Scholar] [PubMed] [CrossRef]
Ren Z, Sun S, Sun R, Cui G, Hong L, Rao B, Li A, Yu Z, Kan Q, Mao Z (2020). A metal-polyphenol-coordinated nanomedicine for synergistic cascade cancer chemotherapy and chemodynamic therapy. Advanced Materials 32: e1906024. https://doi.org/10.1002/adma.201906024 [Google Scholar] [PubMed] [CrossRef]
Sade-Feldman M, Jiao YJ, Chen JH, Rooney MS, Barzily-Rokni M et al. (2017). Resistance to checkpoint blockade therapy through inactivation of antigen presentation. Nature Communications 8: 1136. https://doi.org/10.1038/s41467-017-01062-w [Google Scholar] [PubMed] [CrossRef]
Sangro B, Sarobe P, Hervás-Stubbs S, Melero I (2021). Advances in immunotherapy for hepatocellular carcinoma. Nature Reviews Gastroenterology & Hepatology 18: 525–543. https://doi.org/10.1038/s41575-021-00438-0 [Google Scholar] [PubMed] [CrossRef]
Santini J, John T, Richards AC, Scheidt R, Cima MJ, Langer R (2000). Microchips as controlled drug-delivery devices. Angewandte Chemie International Edition 39: 2396–2407. https://doi.org/10.1002/(ISSN)1521-3773 [Google Scholar] [CrossRef]
Satarkar NS, Zach Hilt J (2008). Hydrogel nanocomposites as remote-controlled biomaterials. Acta Biomaterialia 4: 11–16. https://doi.org/10.1016/j.actbio.2007.07.009 [Google Scholar] [PubMed] [CrossRef]
Sergio SB, Lorena P, Maldonado-Camargo, François O, Carlos R (2015). Assessing magnetic nanoparticle aggregation in polymer melts by dynamic magnetic susceptibility measurements. Journal of Magnetism and Materials 378: 64–72. https://doi.org/10.1016/j.jmmm.2014.10.171 [Google Scholar] [CrossRef]
Sethuraman V, Janakiraman K, Krishnaswami V, Kandasamy R (2021). Recent progress in stimuli-responsive intelligent nano scale drug delivery systems: A special focus towards pH-sensitive systems. Current Drug Targets 22: 947–966. https://doi.org/10.2174/1389450122999210128180058 [Google Scholar] [PubMed] [CrossRef]
Shah S, Dhawan V, Holm R, Nagarsenker MS, Perrie Y (2020). Liposomes: Advancements and innovation in the manufacturing process. Advanced Drug Delivery Reviews 154: 102–122. https://doi.org/10.1016/j.addr.2020.07.002 [Google Scholar] [PubMed] [CrossRef]
Sharma G, Anabousi S, Ehrhardt C, Ravi Kumar M (2006). Liposomes as targeted drug delivery systems in the treatment of breast cancer. Journal of Drug Targeting 14: 301–310. https://doi.org/10.1080/10611860600809112 [Google Scholar] [PubMed] [CrossRef]
Shi R, Zhang Z, Hu X (2019). Nickamine and analogous nickel pincer catalysts for cross-coupling of alkyl halides and hydrosilylation of alkenes. Accounts of Chemical Research 52: 1471–1483. https://doi.org/10.1021/acs.accounts.9b00118 [Google Scholar] [PubMed] [CrossRef]
Shim G, Ko S, Kim D, Le QV, Park GT, Lee J, Kwon T, Choi HG, Kim YB, Oh YK (2017). Light-switchable systems for remotely controlled drug delivery. Journal of Controlled Release 267: 67–79. https://doi.org/10.1016/j.jconrel.2017.09.009 [Google Scholar] [PubMed] [CrossRef]
Siegel RL, Miller KD, Jemal A (2021). Cancer statistics. CA: A Cancer Journal for Clinicians 70: 7–30. https://doi.org/10.3322/caac.21590 [Google Scholar] [PubMed] [CrossRef]
Soetaert F, Korangath P, Serantes D, Fiering S, Ivkov R (2020). Cancer therapy with iron oxide nanoparticles: Agents of thermal and immune therapies. Advanced Drug Delivery Reviews 163–164: 65–83. https://doi.org/10.1016/j.addr.2020.06.025 [Google Scholar] [PubMed] [CrossRef]
Soliman RA, Youness RA, Manie TM, Khallaf E, El-Shazly M, Abdelmohsen M, Handoussa H, Gad MZ (2022). Uncoupling tumor necrosis factor-α and interleukin-10 at tumor immune microenvironment of breast cancer through miR-17-5p/MALAT-1/H19 circuit. Biocell 46: 769–783. https://doi.org/10.32604/biocell.2022.016636 [Google Scholar] [CrossRef]
Stubbs M, McSheehy PM, Griffiths JR, Bashford CL (2000). Causes and consequences of tumour acidity and implications for treatment. Molecular Medicine Today 6: 15–19. https://doi.org/10.1016/S1357-4310(99)01615-9 [Google Scholar] [PubMed] [CrossRef]
Su YL, Yu TW, Chiang WH, Chiu HC, Chang CH, Chiang CS, Hu SH (2017). Hierarchically targeted and penetrated delivery of drugs to tumors by size-changeable graphene quantum dot nanoaircrafts for photolytic therapy. Advance Functional Materials 27: 1700056. https://doi.org/10.1002/adfm.201700056 [Google Scholar] [CrossRef]
Swami R, Kumar Y, Chaudhari D, Katiyar SS, Kuche K, Katare PB, Banerjee SK, Jain S (2021). pH sensitive liposomes assisted specific and improved breast cancer therapy using co-delivery of SIRT1 shRNA and Docetaxel. Materials Science & Engineering C: 120: 111664–111700. https://doi.org/10.1016/j.msec.2020.111664 [Google Scholar] [PubMed] [CrossRef]
Tang D, Chen X, Kang R, Kroemer G (2021). Ferroptosis: Molecular mechanisms and health implications. Cell Research 31: 107–125. https://doi.org/10.1038/s41422-020-00441-1 [Google Scholar] [PubMed] [CrossRef]
Thi TTH, Suys EJ, Lee JS, Nguyen DH, Park KD, Truong NP (2021). Lipid-based nanoparticles in the clinic and clinical trials: From cancer nanomedicine to COVID-19 vaccines. Vaccines 9: 359. https://doi.org/10.3390/vaccines9040359 [Google Scholar] [PubMed] [CrossRef]
Tokumaru Y, Joyce D, Takabe K (2020). Current status and limitations of immunotherapy for breast cancer. Surgery 167: 628–630. https://doi.org/10.1016/j.surg.2019.09.018 [Google Scholar] [PubMed] [CrossRef]
Uthaman S, Huh KM, Park IK (2018). Tumor microenvironment-responsive nanoparticles for cancer theragnostic applications. Biomaterials Research 22: 1–11. https://doi.org/10.1186/s40824-018-0132-z [Google Scholar] [PubMed] [CrossRef]
Verma N, Vinik Y, Saroha A, Nair NU, Ruppin E et al. (2020). Synthetic lethal combination targeting BET uncovered intrinsic susceptibility of TNBC to ferroptosis. Science Advances 6: eaba8968. https://doi.org/10.1126/sciadv.aba8968 [Google Scholar] [PubMed] [CrossRef]
Vijayan V, Uthaman S, Park IK (2018). Cell membrane-camouflaged nanoparticles: A promising biomimetic strategy for cancer theragnostics. Polymers 10: 983–1008. https://doi.org/10.3390/polym10090983 [Google Scholar] [PubMed] [CrossRef]
Vodnala SK, Eil R, Kishton RJ, Sukumar M, Yamamoto TN et al. (2019). T cell stemness and dysfunction in tumors are triggered by a common mechanism. Science 363: eaau0135. https://doi.org/10.1126/science.aau0135 [Google Scholar] [PubMed] [CrossRef]
Vyas D, Lopez-Hisijos N, Gandhi S, El-Dakdouki M, Basson MD, Walsh MF, Huang X, Vyas AK, Chaturvedi LS (2015). Doxorubicin-hyaluronan conjugated super-paramagnetic iron oxide nanoparticles (DOX-HA-SPION) enhanced cytoplasmic uptake of doxorubicin and modulated apoptosis, IL-6 release and NF-kappaB activity in human MDA-MB-231 breast cancer cells. Journal of Nanoscience and Nanotechnology 15: 6413–6422. https://doi.org/10.1166/jnn.2015.10834 [Google Scholar] [PubMed] [CrossRef]
Wang W, Green M, Choi JE, Gijón M, Kennedy PD et al. (2019). CD8+ T cells regulate tumour ferroptosis during cancer immunotherapy. Nature 569: 270–274. https://doi.org/10.1038/s41586-019-1170-y [Google Scholar] [PubMed] [CrossRef]
Wang X, Wang C, Guan J, Chen B, Xu L, Chen C (2021c). Progress of breast cancer basic research in China. International Journal of Biological Sciences 17: 2069–2079. https://doi.org/10.7150/ijbs.60631 [Google Scholar] [PubMed] [CrossRef]
Wang DY, Yang G, van der Mei HC, Ren Y, Busscher HJ, Shi L (2021a). Liposomes with water as a pH-responsive functionality for targeting of acidic tumor and infection sites. Angewandte Chemie International Edition 133: 17855–17860. https://doi.org/10.1002/ange.202106329 [Google Scholar] [CrossRef]
Wang J, Zhu M, Nie G (2021b). Biomembrane-based nanostructures for cancer targeting and therapy: From synthetic liposomes to natural biomembranes and membrane-vesicles. Advance Drug Delivery Reviews 178: 113974. https://doi.org/10.1016/j.addr.2021.113974 [Google Scholar] [PubMed] [CrossRef]
Weber F, Rahnfeld L, Luciani P (2020). Analytical profiling and stability evaluation of liposomal drug delivery systems: A rapid UHPLC-CAD-based approach for phospholipids in research and quality control. Talanta 220: 121320. https://doi.org/10.1016/j.talanta.2020.121320 [Google Scholar] [PubMed] [CrossRef]
Witika BA, Makoni PA, Matafwali SK, Chabalenge B, Mwila C, Kalungia AC, Nkanga CI, Bapolisi AM, Walker RB (2020). Biocompatibility of biomaterials for nanoencapsulation: Current approaches. Nanomaterials 10: 1649. https://doi.org/10.3390/nano10091649 [Google Scholar] [PubMed] [CrossRef]
Xie W, Deng WW, Zan M, Rao L, Yu GT et al. (2019). Cancer cell membrane camouflaged nanoparticles to realize starvation therapy together with checkpoint blockades for enhancing cancer therapy. ACS Nano 13: 2849–2857. https://doi.org/10.1021/acsnano.8b03788 [Google Scholar] [PubMed] [CrossRef]
Xie S, Sun W, Zhang C, Dong B, Yang J, Hou M, Xiong L, Cai B, Liu X, Xue W (2021). Metabolic control by heat stress determining cell fate to ferroptosis for effective cancer therapy. ACS Nano 15: 7179–7194. https://doi.org/10.1021/acsnano.1c00380 [Google Scholar] [PubMed] [CrossRef]
Xu H, Ye D, Ren M, Zhang H, Bi F (2021). Ferroptosis in the tumor microenvironment: Perspectives for immunotherapy. Trends in Molecular Medicine 27: 856–867. https://doi.org/10.1016/j.molmed.2021.06.014 [Google Scholar] [PubMed] [CrossRef]
Xu P, Zeng GM, Huang DL, Feng CL, Hu S et al. (2012). Use of iron oxide nanomaterials in wastewater treatment: A review. Science of the Total Environment 424: 1–10. https://doi.org/10.1016/j.scitotenv.2012.02.023 [Google Scholar] [PubMed] [CrossRef]
Xuan M, Shao J, Zhao J, Li Q, Dai L, Li J (2018). Magnetic mesoporous silica nanoparticles cloaked by red blood cell membranes: Applications in cancer therapy. Angewandte Chemie International Edition 57: 6049–6053. https://doi.org/10.1002/anie.201712996 [Google Scholar] [PubMed] [CrossRef]
Yang Y, Aw J, Xing B (2017). Nanostructures for NIR light-controlled therapies. Nanoscale 9: 3698–3718. https://doi.org/10.1039/C6NR09177F [Google Scholar] [PubMed] [CrossRef]
Yao C, Yang F, Sun L, Ma Y, Wu A (2020). Magnetically switchable mechano-chemotherapy for enhancing the death of tumour cells by overcoming drug-resistance. Nano Today 35: 100967–100974. https://doi.org/10.1016/j.nantod.2020.100967 [Google Scholar] [CrossRef]
Yu J, Wang Y, Zhou S, Li J, Wang J, Chi D, Wang X, Lin G, He Z, Wang Y (2020). Remote loading paclitaxel-doxorubicin prodrug into liposomes for cancer combination therapy. Acta Pharmaceutica Sinica B 10: 1730–1740. https://doi.org/10.1016/j.apsb.2020.04.011 [Google Scholar] [PubMed] [CrossRef]
Yuba E (2020). Development of functional liposomes by modification of stimuli-responsive materials and their biomedical applications. Journal of Materials Chemistry B 8: 1093–1107. https://doi.org/10.1039/C9TB02470K [Google Scholar] [PubMed] [CrossRef]
Zanganeh S, Hutter G, Spitler R, Lenkov O, Mahmoudi M et al. (2016). Iron oxide nanoparticles inhibit tumour growth by inducing pro-inflammatory macrophage polarization in tumour tissues. Nature Nanotechnology 11: 986–994. https://doi.org/10.1038/nnano.2016.168 [Google Scholar] [PubMed] [CrossRef]
1000. Zhang F, Li F, Lu GH, Nie W, Zhang L et al. (2019). Engineering magnetosomes for ferroptosis/immunomodulation synergism in cancer. ACS Nano 13: 5662–5673. https://doi.org/10.1021/acsnano.9b00892 [Google Scholar] [PubMed] [CrossRef]
Zhang P, Chen D, Li L, Sun K (2022a). Charge reversal nano-systems for tumor therapy. Journal of Nanobiotechnology 20: 1–27. https://doi.org/10.1186/s12951-021-01221-8 [Google Scholar] [PubMed] [CrossRef]
Zhang W, Guo Z, Huang D, Liu Z, Guo X, Zhong H (2011). Synergistic effect of chemo-photothermal therapy using PEGylated graphene oxide. Biomaterials 32: 8555–8561. https://doi.org/10.1016/j.biomaterials.2011.07.071 [Google Scholar] [PubMed] [CrossRef]
Zhang H, Li J, Yuan R, Li Y, Zhang Y, Hu X, Qu J, Chen Y, Wang Z, Xia M (2021a). Augment the efficacy of eradicating metastatic lesions and tumor proliferation in breast cancer by honokiol-loaded pH-sensitive targeted lipid nanoparticles. Colloids Surfaces B: Biointerfaces 207: 112008–112019. https://doi.org/10.1016/j.colsurfb.2021.112008 [Google Scholar] [PubMed] [CrossRef]
Zhang J, Pan S, Jian C, Hao L, Dong J, Sun Q, Jin H, Han X (2021b). Immunostimulatory properties of chemotherapy in breast cancer: From immunogenic modulation mechanisms to clinical practice. Frontiers in Immunology 12: 33. https://doi.org/10.3389/fimmu.2021.819405 [Google Scholar] [PubMed] [CrossRef]
Zhang Q, Wei W, Wang P, Zuo L, Li F, Xu J, Xi X, Gao X, Ma G, Xie HY (2017). Biomimetic magnetosomes as versatile artificial antigen-presenting cells to potentiate T-cell-based anticancer therapy. ACS Nano 11: 10724–10732. https://doi.org/10.1021/acsnano.7b04955 [Google Scholar] [PubMed] [CrossRef]
Zhang D, You Y, Xu Y, Cheng Q, Xiao Z, Chen T, Shi C, Luo L (2022b). Facile synthesis of near-infrared responsive on-demand oxygen releasing nanoplatform for precise MRI-guided theranostics of hypoxia-induced tumor chemoresistance and metastasis in triple negative breast cancer. Journal of Nanobiotechnology 20: 104–113. https://doi.org/10.1186/s12951-022-01294-z [Google Scholar] [PubMed] [CrossRef]
Zhao P, Wang M, Chen M, Chen Z, Peng X, Zhou F, Song J, Qu J (2020). Programming cell pyroptosis with biomimetic nanoparticles for solid tumor immunotherapy. Biomaterials 254: 120142–120151. https://doi.org/10.1016/j.biomaterials.2020.120142 [Google Scholar] [PubMed] [CrossRef]
Zheng XC, Ren W, Zhang S, Zhong T, Duan XC et al. (2018). The theranostic efficiency of tumor-specific, pH-responsive, peptide-modified, liposome-containing paclitaxel and superparamagnetic iron oxide nanoparticles. International Journal of Nanomedicine 13: 1495–1504. https://doi.org/10.2147/IJN [Google Scholar] [CrossRef]
Zhu J, Dai P, Liu F, Li Y, Qin Y et al. (2020). Upconverting nanocarriers enable triggered microtubule inhibition and concurrent ferroptosis induction for selective treatment of triple-negative breast cancer. Nano Letters 20: 6235–6245. https://doi.org/10.1021/acs.nanolett.0c00502 [Google Scholar] [PubMed] [CrossRef]
Zhu WJ, Qu CX, Zhu QI, Chen WI, Li F, Yuan ZQ, Liu Y, You BG, Zhang XN (2017). Low-density lipoprotein-coupled micelles with reduction and pH dual sensitivity for intelligent co-delivery of paclitaxel and siRNA to breast tumor. International Journal of Nanomedicine 12: 3375–3393. https://doi.org/10.2147/ijn.s126310 [Google Scholar] [PubMed] [CrossRef]
Cite This Article
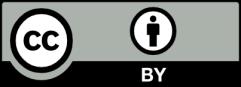
This work is licensed under a Creative Commons Attribution 4.0 International License , which permits unrestricted use, distribution, and reproduction in any medium, provided the original work is properly cited.